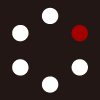
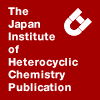
HETEROCYCLES
An International Journal for Reviews and Communications in Heterocyclic ChemistryWeb Edition ISSN: 1881-0942
Published online by The Japan Institute of Heterocyclic Chemistry
e-Journal
Full Text HTML
Received, 3rd July, 2008, Accepted, 15th August, 2008, Published online, 18th August, 2008.
DOI: 10.3987/COM-08-S(F)38
■ Synthetic Studies towards the Identification of Novel Capuramycin Analogs with Mycobactericidal Activity
Michio Kurosu* and Kai Li
Immunology & Patholog, Department of Microbiology, Colorado State University, 1682 Campus Delivery, Fort Collins, CO 80523-1682, U.S.A.
Abstract
Expeditious syntheses of capuramycin, an effective MraY inhibitor in vivo, analogs are described. Synthetic schemes reported here are extremely useful for the generation of capuramycin analogs to identify minimum structure requirement to exhibit antimycobactericidal activity.Mycobacterium tuberculosis (Mtb) causes tuberculosis (TB) and is responsible for nearly two million deaths annually.1 In particular, people who have HIV-AIDS patients are susceptible to TB infection.2 Moreover, the emergence of multidrug-resistant strains of Mtb seriously threatens TB control and prevention efforts.3 Recent studies have shown that infection with Mtb enhances replication of HIV and may accelerate the progression of HIV infection to AIDS. In addition, there are significant problems present with respect to treatment of AIDS and TB co-infected patients; rifampicin (a key drug of DOTS therapy) shows significant interactions with protease inhibitors and non-nucleoside reverse transcriptase inhibitors.4 Therefore, it is an urgent need to identify in vivo effective mycobactericidal drug leads which interfere with unexploited bacterial molecular targets.
Since peptidoglycan (PG) is an essential bacterial cell-wall polymer, the machinery for PG biosynthesis provides a unique and selective target for antibiotic action.5 By far, the most commonly exploited target in PG synthesis are the penicillin binding proteins (PBPs), which are inhibited by the β-lactams and glycopeptides. Unfortunately, these compounds are of limited use in TB infections due to the interplay of β-lactamase activity and the relative impermeability of the mycolic acid layer in mycobacteria.6 Three other biosynthetic steps in PG synthesis can be targeted by antibiotics in current clinical use; these antibiotics include bacitracin, D-cycloserine and fosfomycin.7 Regrettably, all are of limited usefulness in treating TB.8 Thus, PG biosynthesis appears to be a source of unexploited drug targets in mycobacterial pathogens. Earlier cytoplasmic steps in peptidoglycan biosynthesis are catalyzed by highly conserved Mur enzymes. Therefore, inhibitors of any of these enzymes (MurA~F) would likely possess a broad spectrum of action. However, in spite of significant efforts by pharmaceutical industries, MurA~F inhibitors have not yet yielded drug leads.9 Similarly, there is no antibiotic or a small molecule directed against MraY, which transfers UDP-N-acetylmuramyl-L-alanyl-γ-D-glutamyl-meso-diaminopimelyl- D-alanyl-D-alanine (Park’s nucleotide) to a molecule of prenyl phosphate forming Lipid I, being developed for drugs for clinical trials. However, MraY inhibitors such as nucleoside antibiotics exhibit significant antibacterial activity in vitro.10 Although many MraY inhibitors of natural product origin (i.e. liposidomycins, caprazamycins, and muraymycins)11 are very complex and no extensive medicinal chemistry efforts with these molecules have been reported,12 the structures of capuramycin (1)13 and A-500359A (214, Figure 1), isolated from Streptomyces spp., are less complicated and their chemical synthesis to perform medicinal chemistry seems to be feasible.
To date, however, only one total synthesis of capuramycin was reported by Knapp and Nandan.15 Their synthesis requires 21 linear steps from diisopropylidine-D-glucofuranose and seems to be difficult to apply to the synthesis of the diversity structures of capuramycin analogs. A Sankyo group in Japan reported chemical modification of A-500359A (2) and biological evaluation of A-500359A analogs.16 We have recently established a flexible synthesis of campuramycin using the synthons, i, ii, and iii (Figure 1), and obtained extensive knowledge of synthetic accessibility of capuramycin analogs.17 However, our synthetic route for campuramycin relies on 1) oxidative cleavage to generate the carboxyamide, and 2) oxidation of the 6-position of mannose moiety of the coupling product (i + ii) to form the manno-pyranuronate. These additional several steps required for the synthesis of intact capuramycin core structure are not ideal for the generation of a library of capuramycin analogs in a time-efficient manner. Therefore, we envisioned identifying the indispensable functionalities of capuramycin to exhibit antimycobactericidal activity. To this end we plan to synthesize the decarboxyamide-capuramycin 3a, and its congeners 4a, 5a, 6a, and their methyl isomer 3b, 4b, 5b, 6b (Figure 1). Because the saturated analogs 4a, 4b, 6a, and 6b can be synthesized by skipping the elimination step, we, thus, focused in establishing expeditious preparations of 3a, 3b, 5a, and 5b. Herein, we describe a highly flexible synthesis of capuramycin analogs 3a, 3b, 5a, and 5b with a minimum number of protecting group manipulations.
The revised synthetic route for 3a, 3b, 5a, and 5b includes 1) pyranulonidations of 7a and 7b (Segment A) with the pyranuroates donors 8a and 8b (Segment B), 2) the base-catalyzed elimination reactions of the coupling products (7 + 8) to furnish the α,β-unsaturated esters, 3) amide-forming reaction with Segment C, and 4) global deprotections in a single step (Figure 2). In order to perform pyranurosylation and elimination reactions with a minimum number of protecting group manipulations, choice of protecting groups for each building block (7 and 8) requires careful consideration. In addition, it is very important to devise a flexible synthetic route for the generation of a wide variety of capuramycin analogs from the point of medicinal chemistry.
Limited examples of glycosylations with pyranuronoates were found in literatures, and the Schmidt glycosylation conditions using Lewis acid are the standard for pyranulonidation reactions.18 Having considered the synthetic and medicinal chemistry aspects described above and limited examples of pyranulonidations, we decided to introduce the protecting group, (2,6-dichloro-4-methoxyphenyl)(2,4-dichlorophenyl)methyl alcohol (10) which showed an unusual stability to Brønstead acids (i.e. 15% TFA, 30% HF, 2N HCl, and HBr/AcOH), Lewis acids (i.e. TiCl4, ZnCl2, AlCl3, B(C6F5)3, BCl3, BBr3, TMSOTf, and La(OTf)3), and bases (i.e. 40% NH4OH, 10% LiOH, and DBU).19 Such a protecting group would enable us to accomplish the synthesis of capuramycin analogs 3a, 3b, 5a, and 5b with a minimum number of deprotection/protection manipulations.
The convenient syntheses of the glycosyl acceptors 7a and 7b were illustrated in Scheme 1. The N(3)-BOM uridine 1120 was subjected to double-silylation reactions with TBSCl and imidazole in DMF to furnish the 2’,5’-O-di-TBS product 12 in 89% yield. It was realized that the treatment of 12 with KH underwent the silyl group migration to furnish the potassium 2’-alkoxide which could be alkylated with a variety of electrophiles (i.e. MeI, EtI, octyl iodide, and allyl bromide); the silyl migration of 12 was completed within 0.5 h and followed by methylation with MeI provided 13b (R = Me) in 95% yield.21 The structures of 13a and 13b were established through 1H-NMR NOESY experiments. On the contrary, the treatment of 12 with KH in the presence of MeI (10 equiv) yielded a mixture of 13a and 13b with 3~5:1 (13a / 13b) selectivity. Selective desilylation of the primary TBS group of 13 with cooled 90% TFA (–5 oC) followed by hydrogenolysis of the BOM group gave rise to the partially protected-uridines 7a and 7b in 75~95 % overall yield.
D-Gluco-pyranuroate glycosyl donor 8b was synthesized in four steps with greater than 68% overall yield from glucuronic acid (19). On the other hand, synthesis of D-manno-pyranuroate donor 8a requires additional several steps to selectively oxidize the 6-OH group of 16.15 1,2,3,4-Tetra-O-acetyl-D-mannopyranose (16) was oxidized under typical Sharpless conditions (RuCl3, NaIO4) and the generated carboxylic acid was esterified with rac-10.22 Selective cleavage of the anomeric acetate of 17a followed by the reaction of the free anomeric alcohol with Cl3CCN in the presence of catalytic amount of DBU afforded the β-imidate 8a; the conversion of 8a from D-mannose was greater than 50% overall yield.
The gluculonidations of alcohols with 8b are known to be low yielding reactions and several variations of glucuronate imidates have been devised in order to improve reactivity.23 Nonetheless, the gluculonidations of 7a and 7b with 8b were examined under typical Schmidt conditions. It was observed that TMSOTf (5~10 mol%) catalyzed gluculonidation of 7a with 8b at –20 oC furnished the orthoester exclusively with near quantitative yield. Rearrangement of the orthoester to the desired β-glycoside 19a were not observed at elevated temperature or under the conditions of an increased amount of TMSOTf (100~250 mol%). Interestingly, the same reaction was successfully catalyzed by using BF3•OEt2 (300 mol%) at –20 oC to afford 19a in 50% yield without the formation of the orthoester; however, this transformation under these conditions required a relatively long reaction time of 10~14 h. Similarly, 7b could be coupled with 8b to furnish 19b in 49% yield. Although the desired product 20a and 20b could be synthesized by the reaction of 7a and 7b with 8a via the conditions performed for the syntheses of 19, D-manno-pyranulonidation resulted in lower isolation yields (25~40% yields) due to the formation of the orthoesters. In general, the orthoester formation observed using acetyl group-protected glycosyl donors is able to be diminished by using pivaloyl protecting groups, however, we could not improve D-manno- pyranulonidations with disarmed donors such as the benzoyl- or pivaloyl-protected D-manno-pyranuronate imidate.24 Even though isolation yields for 19 and 20 were moderate, these products could be isolated simply by column chromatography. In addition, 8a, 8b, 7a, and 7b could be synthesized a multi-gram quantity without difficulty. Thus, further reaction optimization was not undertaken for this program. E2 elimination reactions of 19 and 20 with a stoichiometric amount of DBU furnished 21 and 22 in 65~85% yields. (2,6-Dichloro-4-methoxyphenyl)(2,4-dichlorophenyl)methyl ester and TBS groups were simultaneously deprotected with 50% TFA to provide the acid-alcohol and trifluoroacetic acid ester of 10.25 The resulting crude mixture was coupled with (2S)-aminocaprolactam (9) under a condition of HOAt, EDCI, and NMM to yield the ligation product in quantitative yield. The acetyl-protected decarboxyamide-capuramycin analogs were hydrolyzed by using LiOH in aq. THF to provide 3a, 3b, 5a, and 5b in 90~95% yields.26 Thus, we demonstrated a flexible synthesis of capuramycin analogs in which the 2’,3’-positions in ribose, 4-deoxy-4-hexenuronic acid, and amide moieties can be selectively functionalized or be replaced with a wide range of physicochemically interesting building blocks. It is worthwhile mentioning that rac-(2,6-dichloro-4-methoxyphenyl)(2,4-dichlorophenyl)methanol (10) is one of very few protecting groups which 1) are stable to Brønstead bases and Lewis acids, 2) can be cleaved by a volatile acid such as TFA, and 3) are reusable. Introduction of a protecting group possessing such chemical properties in the synthesis of capuramycin analogs enable us to synthesize desired analogs with a minimum number of protecting group manipulations. We will 1) functionalize the 2’- and 3’-positions of Segment A (Figure 2) with a wide range of alkyl groups, 2) introduce the other pyranuronate and furanonate in place of D-manno-pyranuronate (Segment B), and 3) diversify the structure of molecules with a wide variety of primary and secondary amines (Segment C). Detailed structure antimycobactericidal activity relationship of library molecules based on capuramycin will be reported elsewhere.
ACKNOWLEDGEMENTS
We thank the National Institutes of Health and Colorado State University for generous financial support. Mass spectra were obtained on instruments supported by the NIH Shared Instrumentation Grant GM49631.
Dedicated to Professor Emeritus Keiichiro Fukumoto on the occasion of his 75th birthday.
References
1. A. M. Rouhi, Chem. Eng. News, 1999, 77, 52.
2. J. Cohen, Science, 2004, 306, 1872. CrossRef
3. E. E. Telzak, K. D. Chirgwin, E. T. Nelson, J. P. Matts, K. A. Sepkowitz, C. A. Benson, D. C. Perlman, and W. M. El-Sadr, Int. J. Tuberc. Lung. Dis., 1999, 3, 337.
4. P. Nunn, R. Brindle, L. Carpenter, J. Odhiambo, K. Wasunna, R. Newnham, W. Githui, S. Gathua, M. Omwega, and K. Mcadam, American Review of Respiratory Disease, 1992, 146, 849.
5. a) J. van Heijenoort, Cell. Mol. Life Sci., 1998, 54, 300; CrossRef b) J. van Heijenoort, Glycobiology, 2001, 11, 25R. CrossRef
6. V. Jarlier, L. Gutmann, and H. Nikaido, Antimicrob. Agents Chemother., 1991, 35, 1937.
7. K. A. L. De Smet, K. E. Kempsell, A. Gallagher, K. Duncan, and D. B. Young, Microbiology, 1999, 145, 3177.
8. W. E. Sanders and C. C. Sanders, Ann. Rev. Pharmacol. Toxicol., 1979, 19, 53. CrossRef
9. S. S. Eveland, D. L. Pompliano, and M. S. Anderson, Biochemistry, 1997, 36, 6223.. CrossRef
10. a) A. Bryskier and A. C. Dini, Antimicrobial Agents: Antibacterials and Antifungals, 2005, 377; b) C. Dini, N. Drochon, S. Feteanu, J. C. Guillot, C. Peixoto, and J. Aszodi, Bioorg. Med. Chem. Lett., 2001, 11, 529; CrossRef c) H. Hu, Q. Zhang, B. Zhu, B. Gong, and B. K. Ochi, Zhongguo Kangshengsu Zazhi, 2003, 28, 53; d) A. Yamashita, E. Norton, P. J. Petersen, B. A. Rasmussen, G. Singh, Y. Yang, T. S. Mansour, and D. M. Ho, Bioorg. Med. Chem. Lett., 2003, 13, 3345. CrossRef
11. M. Kurosu, P. Narayanasamy, and D. C. Crick, Heterocycles, 2007, 72, 339. CrossRef
12. C. G. Boojamra, R. C. Lemoine, J. C. Lee, R. Léger, K. A. Stein, N. G. Vernier, A. Magon, O. Lomovskaya, P. K. Martin, S. Chamberland, M. D. Lee, S. J. Hecker, and V. J. Lee, J. Am. Chem. Soc., 2001, 123, 870. CrossRef
13. H. Seto, N. Otake, S. Sato, H. Yamaguchi, K. Takada, M. Ito, H. S. M. Lu, and J. Clardy, Tetrahedron Lett., 1988, 29, 2343. CrossRef
14. Y. Muramatsu, A. Muramatsu, T. Ohnuki, M. I. Miyazawa, M. Kizuka, R. Enokita, S. Tsutsumi, M. Arai, Y. Ogawa, T. Suzuki, T. Takatsu, M. and Inukai, J. Antibiot., 2003, 56, 243.
15. S. Knapp and S. R. Nandan, J. Org. Chem., 1994, 59, 281. CrossRef
16. a) H. Hotoda, M. Furukawa, M. Daigo, K. Murayama, M. Kaneko, Y. Muramatsu, M. M. Ishii, S. Miyakoshi, T. Takatsu, M. Inukai, M. Kakuta, T. Abe, T. Harasaki, T. Fukuoka, Y. Utsui, and S. Ohya, Bioorg. Med. Chem. Lett. 2003, 13, 2829; CrossRef b) T. Koga, T. Fukuoka, N. Doi, T. Harasaki, H. Inoue, H. Hotoda, M. Katsuta, Y. Muramatsu, N. Yamamura, M. Hoshi, T. Hirota, J. Antimicrob. Chemother., 2004, 54, 755. CrossRef
17. M. Kurosu and K. Li, unpublished data.
18. a) R. R. Schmidt and G. Grundler, Synthesis, 1981, 11, 885; CrossRef b) G. Blatter and J-C. Jacquinet, Carbohydr. Res., 1996, 288, 109; c) J. R. Ferguson, J. R. Harding, K. W. Lumbard, F. Scheinmann, and A. V. Stachulski, Tetrahedron Lett., 2000, 41, 389; CrossRef d) J. Tamura, H. Urashima, K. Tsuchida, H. Kitagawa, and K. Sugahara, Carbohydr. Res., 2001, 332, 41; CrossRef e) N. Hada, T. Ogino, H. Yamada, and T. Takeda, Carbohydr. Res., 2001, 334, 7; CrossRef f) H. A. Orgueira, A. Bartolozzi, and P. H. Schell, Chem. Eur. J., 2003, 9, 140; CrossRef g) N. Pitt, R. M. Duane, A. O‘Brien, W. Helena, S. J. Wilson, K. M. O‘Brien, and P. V. Murphy, Carbohydr. Res., 2004, 339, 1873; CrossRef h) Y. Jin, N. Hada, J. Oka, O. Kanie, S. Daikoku, Y. Kanie, H. Yamada, and T. Takeda, Chem. Pharm. Bull., 2006, 54, 485; CrossRef i) J. L. de Paz, C. Noti, and P. H. Seeberger, J. Am. Chem. Soc., 2006, 128, 2766. CrossRef
19. a) M. Kurosu, K. Biswas, and D. C. Crick, Org. Lett., 2007, 9, 1141; CrossRef b) M. Kurosu, K. Biswas, P. Narayanasamy, and D. C. Crick, Synthesis 2007 16, 2513; CrossRef c) M. Kurosu, P. Narayanasamy, and D. C. Crick, Heterocycles, 2007, 73, 169. CrossRef
20. a) M. Krecmerova, H. Hrebabecky, and H. Antonin, Collect. Czech. Chem. Commun., 1996, 61, 645; CrossRef b) S. Ichikawa, S. Shuto, N. Minazawa, and A. Matsuda, J. Org. Chem., 1997, 62, 1368. CrossRef
21. Because 13b was isolated exclusively in the conversion of 12 to 13b it was concluded that the C3-TBS silyl group did not migrate to the C2-position. Thus, no rapid equivilation of the silyl group was taking place.
22. We have not observed separation of the diastereomers caused by rac-10 throughout the syntheses of 21 and 22.
23. J. R. Harding, C. D. King, J. A. Perrie, D. Sinnott, and A. V. Stachulski, Org. Biomol. Chem., 2005, 3, 1501. CrossRef
24. Trace amounts of the desired coupling product were isolated with the pivaloyl- or benzoyl-protected imidate of D-manno-pyranuronate.
25. The TFA ester of 10 was saponified with NH3 in MeOH (for 12 h) to regenerate 10 in quantitative yield.
26. Syntheses of 4a, 4b, 6a, and 6b (Figure1) were accomplished by skipping the elimination step (19 → 22) in Scheme 2. Representative physical data for the molecules listed in Figure 1 were listed below. 3a: [α]20D +14.2 (c 0.1 in MeOH); IR (film) 3378, 1680, 1070 cm-1; 1H NMR (400 MHz, CDCl3) δ 7.90 (d, J = 8.4 Hz, 1H), 5.94 (d, J = 3.2 Hz, 1H), 5.93 (d, J = 3.2 Hz, 1H), 5.72 (d, J = 8.4 Hz, 1H), 5.19 (d, J = 4.8 Hz, 1H), 4.57 (d, J = 10.2 Hz, 1H), 4.35 (t, J = 4.0 Hz, 1H), 4.20 (t, J = 4.2 Hz, 1H), 4.16-4.07 (m, 3H), 3.80 (dd, J = 4.4, 8.8 Hz, 2H), 3.25 (m, 2H), 2.00 (m, 2H), 1.84 (m, 2H), 1.57 (m, 1H), 1.37 (m, 1H); 13C NMR (100 MHz, CDCl3) δ 176.9, 166.2, 162.7, 152.2, 143.5, 142.5, 109.8, 103.3, 103.0, 88.5, 85.0, 82.0, 71.2, 768.3, 67.8, 67.5, 58.9, 53.4, 42.5, 32.5, 29.9, 29.2; HRMS (ESI) Calcd. for C22H30N4NaO11 (M+Na)+: 549.1809; found: 549.1812. 5a: [α]20D +12.0 (c 0.5 in MeOH); IR (film) 3390, 1681, 1463, 1087 cm-1; 1H NMR (300 MHz, CDCl3) δ 7.87 (d, J = 8.1 Hz, 1H), 6.05 (d, J = 3.9 Hz, 1H), 5.99 (d, J = 4.8 Hz, 1H), 5.70 (d, J = 8.4 Hz, 1H), 5.16 (d, J = 4.5 Hz, 1H), 4.56 (d, J = 11.1 Hz, 1H), 4.33 (t, J = 5.1 Hz, 1H), 4.18 (dd, J = 2.1, 11.1 Hz, 1H), 4.12 (t, J = 3.3 Hz, 2H), 4.01 (t, J = 4.8 Hz, 1H), 3.91 (dd, J = 2.7, 11.1 Hz, 1H), 3.79 (t, J = 4.2 Hz, 1H), 3.48 (s, 3H), 3.26 (m, 2H), 1.97 (m, 2H), 1.82 (m, 2H), 1.52-1.34 (m, 2H); 13C NMR (100 MHz, CDCl3) δ 176.8, 166.3, 162.5, 152.4, 143.6, 143.0, 109.7, 103.2, 102.8, 88.4, 84.6, 84.3, 72.0, 70.4, 70.1, 67.5, 58.8, 53.5, 42.6, 32.5, 30.0, 29.2; HRMS (ESI) Calcd. for C22H30N4NaO11 (M+Na)+: 549.1809; found: 549.1815.