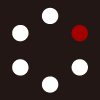
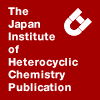
HETEROCYCLES
An International Journal for Reviews and Communications in Heterocyclic ChemistryWeb Edition ISSN: 1881-0942
Published online by The Japan Institute of Heterocyclic Chemistry
e-Journal
Full Text HTML
Received, 30th July, 2008, Accepted, 30th September, 2008, Published online, 2nd October, 2008.
DOI: 10.3987/COM-08-S(F)80
■ Synthesis and Properties of Novel Biologically Interesting Polycyclic 1,3,4-Oxadiazoles Containing Acridine/Acridone Moieties
Zdenka Fröhlichová, Jana Tomascikova, Ján Imrich,* Pavol Kristian, Ivan Danihel, Stanislav Böhm, Danica Sabolová, Mária Kozurková, and Karel D. Klika
Department of Organic Chemistry, P. J. Safárik University, Moyzesova 11, SK-041 67 Kosice, The Slovak Republic
Abstract
A series of polycyclic 1,3,4-oxadiazoles bearing acridine and acridone pharmacophores were synthesized as potential noncovalent DNA-binding and antitumor agents. The synthesis of oxadiazoles with acridone moiety was performed exploring oxidative cyclization of corresponding aldimines via bromine and the acridine derivatives by cyclization of acylthiosemicarbazides with mercuric oxide. The spectroscopic properties of the compounds in the case of acridonoxadiazoles showed an efficient binding activity to DNA (K = 5.3-9.2 x 104 M-1), whereas the acridine analogues are suitable as biomarkers. The structure of compounds were characterized by spectral methods (UV-vis, IR, 1H, 13C, and 2D NMR) and quantum-chemical calculations (DFT, ZINDO).INTRODUCTION
A number of papers have appeared recently on the interesting biological properties of various oxadiazole derivatives. Of note, some polysubstituted pyrazoles comprising oxadiazole rings exhibited antitumor activity and were found to be anti-HCV agents.1 Moreover, attention was paid to oxadiazoles with antimicrobial and anti-inflammatory properties2−4 and other oxadiazoles were also synthesized and found
to possess expected biological activities.5,6 In addition to these properties, oxadiazoles exhibit antifungal, anticonvulsive, pesticidal, hypotensive, hypoglycemic, diuretic, and other activities.5,6 Various methods for the synthesis of 1,3,4-oxadiazoles starting from hydrazides exist, e.g. the reaction of hydrazides with isothiocyanates to provide thiosemicarbazides followed by refluxing with mercuric oxide in absolute ethanol1 or the oxidative cyclization of sugar hydrazones, derived from acylhydrazides and aldopentoses/aldohexoses, with bromine.6 An alternative synthesis of 1,3,4-oxadiazoles from hydrazides is the treatment of acylsemicarbazides with POCl3,7 where the acylsemicarbazides are produced from acylthiosemicarbazides by S→O exchange using mesitylnitrile oxide (MNO). 2,5-Disubstituted-1,3,4- oxadiazoles have also been prepared by microwave-assisted synthesis4 and by the thermolysis of geminal diazides.8
Acridine derivatives are known as excellent intercalating pharmocophores.9 The aim of this work was to synthesize and investigate the properties of novel 1,3,4-oxadiazoles substituted with either an acridine or an acridone moiety as part of our continuing interest in biologically active acridine derivatives. The ensuing compounds were characterized spectroscopically using UV-vis, fluorescence, IR, 1H, 13C (2D NMR) and by quantum-chemical calculations using DFT (B3LYP/6-311+G(d,p)) and ZINDO level of theory. Biological activity was evaluated using calf thymus DNA (ctDNA) to ascertain the binding and bio-interaction of the compounds.
RESULTS AND DISCUSSION
Synthesis
The first set of 1,3,4-oxadiazoles containing an acridone moiety was prepared starting from 2,2'-iminodibenzoic acid (1) (Scheme 1). Acridonoxadiazoles 6 were obtained from 9-oxo-9,10-dihydro-acridine-4-carboxylic acid 2 synthesized according to method10 when polyphosphoric acid (PPA) was used for cyclization of 1. The esterification of 2 to corresponding methyl ester 3 was realized using methyl iodide in DMF/NaH, from which the corresponding hydrazide 4 was prepared by treatment with hydrazine monohydrate. Condensation11 of 4 with a series of aldehydes (RCHO: a, R = phenyl; b, R = 4-bromophenyl; c, R = 4-methoxyphenyl; d, R = 2,4,6-trimethylphenyl; e, R = 3-nitrophenyl), then yielded aldimines 5a−e. Aldimines 5a−e were then cyclized by bromine/acetic acid oxidation6 to 1,3,4-oxadiazoles 6a−e. The second set of 1,3,4-oxadiazoles containing an acridine moiety was then prepared (see Scheme 2) by exploiting acylthiosemicarbazides 7a−d (acyl groups: a, CH3CO; b, PhCO; c, 3-pyridinylCO; d, 4-pyridinylCO) described in a previous work.9b Compounds 7a−d were cyclized to 1,3,4-oxadiazoles 8a−d by refluxing with mercuric oxide in absolute ethanol.1 For comparative purposes, following better yields, 7d was also treated with MNO to yield acylsemicarbazide 9d, which was then cyclized to oxadiazole 8d using POCl3.7 Comparing the yields of the oxadiazole products prepared by other methods,12−14 this attempt however was not profitable because these were practically the same small (12 and 14%), but the latter was more labor and time consuming. There are two main reasons for the low product yields obtained. The first is that the acridine moiety is not very stable and as well as side reactions leading to decomposition products present in the reaction mixture, also a black-brown precipitant was formed. The second is the very poor solubility of the starting thiosemicarbazides 7a−d which only formed a suspension in the ethanol.
Spectroscopy and structure
The characteristic 1H and 13C NMR chemical shifts of the acridone skeleton for oxadiazoles 6a−e were readily assigned using HSQC and HMBC spectra: the H-10's (ca. 11.5 ppm), C-9's (178 ppm), and the quaternary carbons C-4a' (139 ppm), C-10a' (140 ppm), C-8a' (122 ppm), and C-9a' (122 ppm). The values for these signals are in good agreement with those for the 4-acridone carboxamide imines11 and comparable, except for the carbonyl carbon, with acridine derivatives consisting of a dihydroacridine ring.9b The carbons of the oxadiazole ring resonated at ca. 166 ppm for C-2 and 158 ppm for C-5, which are similar to literature values5,15 and which were able to be unambiguously assigned by HMBC correlations from the ortho protons of the phenyl substituent. The structures of oxadiazoles 8a−d possessing an acridine moiety were confirmed to be in the dihydroacridine tautomeric form and this is consistent with expectations as the N-10' atom of the acridinyl moiety is well known16,9b,f for its propensity to retain a proton, i.e. the H-10' tautomer dominates the tautomeric equilibrium depicted in Scheme 2. Characteristic 1H and 13C NMR chemical shift values, as averaged values for the set, were observed at 118.1 ppm for C-4',5', 140.0 ppm for C-4a',10a', and 12.3 ppm for H-10', though most diagnostic16a,b for the presence of the H-10' tautomer is the shielded position of the C-4',5' signals, in this instance at 118.1 ppm (typically ca. 119 ppm). Uncharacteristically, rotation about the N11'−C9' bond was fast thus rendering the two sides of the acridine moiety equivalent on the NMR timescale in compounds 8a−d. Though the two sides of the acridine moiety are usually observed as distinct in these compounds, the prevailing conditions can nevertheless increase the rate of exchange to render them equivalent,9b though paradoxically the labile H-10' can still be observed for samples of 8a−d measured in this work.
Taking into account the possibility to form hydrogen bonds in acridone compounds, the concentration dependence of N-H intensity bands in range 3200−3600 cm−1 measured in carbon disulfide for derivatives 6a, 6d, and 6e was performed (see Experimental). The intensity of the absorption N-H bands with dilution decreased and at dilution 1:100 two new bands occurred (Figure 1) confirming the presence of intramolecular bonding in addition to the intermolecular bonding typical for an acridone skeleton.11 These two bands could be ascribed to N10-H….O(oxadiazole) and N10-H…N (oxadiazole) hydrogen bondings (Figure 1). Two characteristic regions revealed in UV-vis spectra of oxadiazoles 6a−e and 8a−d. The first with higher intensity in the region 230−300 nm and the second absorption band (Figure 2) in region at 370−420 nm was registered, which could be atributed to the π→π* transitions connected with electron transfer due to the conjugation of oxadiazol heterocycles with acridone/acridine skeleton.
To obtain more details, the semiempirical ZINDO method was used to interpret UV-vis spectra of derivatives 6a and 8b. The theoretical and experimental spectra of 6a are similar but shifted (scaling factor = 1.188). From analysis of electronic transitions followed that all significant transitions are of π→π* type. The most shifted maximum (λ = 420 nm, oscillator strength f = 0.61) corresponds to HOMO→LUMO transition with both two π-orbitals HOMO and LUMO spreading over whole molecular system including phenyl substituent (Figure 3).
The situation is similar at HOMO-1→LUMO (λ = 292 nm, f = 0.79) as well as the other transitions and leads to conclusion, that the molecule 6a is presented at excitation as one complex chromophore system. For derivative 8b, the most shifted maximum (λ = 419 nm, f = 0.21) is HOMO→LUMO transition again with HOMO orbital spreading over the whole molecular system. LUMO orbital however is limited really to acridine part of the molecule. Similar findings were assigned for other excitations, so we can conclude that in this case two chromophore systems are present.
Electron density can be reduced in the acridine moiety due to conjugation extending over the oxadiazole ring with the R group leading to diminished relative fluorescence. The changes in the fluorescence of compounds 8a−d (see Table 1) due to the changing R substituent can be rationalized on the basis of electron withdrawal by the R group from the acridine segment via the oxadiazole ring. The more effective the electron withdrawal by the R group, the greater the reduction in fluorescence that can be expected. Electron withdrawal from the acridine segment is best facilitated by delocalization effected by conjugation and this process has been used previously to explain the fluorescence results observed in 9-iminoacridines.17 Thus, 8a has the highest fluorescence of the set due to an electron-donating methyl group, but the fluorescence is reduced when the methyl is substituted by an electron withdrawing phenyl (b) or 3-pyridinyl (c) group. In the case of d, mesomeric structures can be drawn with a negative charge localized on nitrogen rather than on carbon, thus explaining the even further diminished relative fluorescence for this compound. Thus, 8a−d follow an anticipated order: b and c have comparable fluorescence and are reduced with respect to a, whilst d has the least fluorescence of the set. In the case of 6a−e, using 6a (R = phenyl) as a base value, 6b−d are all accordingly relatively more fluorescent since they all possess para/ortho directing groups in para/ortho positions on the attached phenyl group and
thus inhibit the additional flow of electrons from the acridone ring into the phenyl ring by conjugation, thereby leading to greater fluorescence. Inexplicably though, 6e with a nitro group on the meta position of the attendant phenyl ring is the most fluorescent of the set and thus factors other than simple electron re-direction are in play in this case.
Molecular Modeling
Oxadiazoles 6a−e were examined by quantum-chemical calculations using DFT at the B3LYP/6-311+G(d,p) level of theory and two low-energy rotamers for rotation about the C2−C4' bond were found as optimized structures (see Figure 4). Interestingly, the structural segments in both rotamers A and B are all coplanar, driven no doubt by a desire for extended delocalization, but also by the availability
for intramolecular H-bonding between H-10' and the heteroatoms of the oxadiazole ring (O-1 or N-3). Similar coplanarity was also found for 2-oxadiazo-5-yl aniline18 thus enabling efficient conjugation of the structural segments in that system and it is clear that the same stabilizing forces also drive this system here. Despite some predilection for steric hindrance due to the phenyl groups of the R substituent, in each case, the B rotamers, where N-3 is located close to H-10', were found to be lower in energy, ca. 6.5 kcal mol−1, compared to structures A where O-1 is spatial to H-10'. This result may simply be just a reflection of the H-bonding preferences (sp3-hybridized O vs. sp2-hybridized N) as it does not appear that steric hindrance plays a role since d, with a sterically congestable 2,4,6-trimethylphenyl group, is not dissimilar to the other four members of the set. The calculated intramolecular distances rO1,H10' and rN3,H10' for A and B are very similar (1.974 and 1.934 Å, respectively). However, it is conceivable that the electron lone pairs of the oxygen in 6a−e are weakened by conjugation with the ring nitrogen atoms. This is evident by the rather surprisingly low negative charge on the oxygen atom, particularly in comparison to the nitrogen N-3 atom (Table 2). Moreover, in comparison between structures A and B, the charge on O-1 is little changed, since it is either H-bonding to H-10' (in structure A) or donating electrons to N-3 when it is not (structure B).
The charge on N-10' changes only modestly between the two structures, since H-bonding is present in both and it is only the donor atom that changes. For N-3, a sizeable change is observed between the two structures and this accounted for by greater electron donation from O-1 when it is not involved in H-bonding which renders it much more negative in structure B. Experimental findings from IR spectra (Figure 1) pointing out the presence both rotamers [N10-H…O (oxadiazol) and N10-H…N3 (oxadiazol)] are in accord with theoretical analysis.
Oxadiazoles 8a−d were also evaluated by quantum-chemical calculations at the same level of theory for two tautomers, H-11' and H-10'. The optimized tautomer structures are depicted in Figure 5 where the H-10' tautomers are seen to be able to maintain all three structural segments co-planar for conjugation despite obvious steric hindrance between the oxadiazole ring and H-8'. In the H-11' tautomers, this steric congestion is relieved by bond rotation about the N11'−C9' bond as conjugation is less amenable through the sp3-hybridized N-11' thereby diminishing stabilization via this process.
The H-10' tautomers (D), in concert with the spectroscopic results, were found to be preferred over their H-11' tautomers (C) in each case, but moreover, there was a clear trend across the series (for a (CH3), 1.8; b (phenyl), 2.1; c (3-pyridinyl), 2.6; and d (4-pyridinyl), 3.7 kcal mol−1) indicating that the more effective the R substituent is in electron withdrawal, the more stable the H-10' tautomer is with respect to its counterpart H-11' tautomer. Only for the H-10' tautomer where N-11' is sp2-hybridized and able to conjugate can electron withdrawal by the R substituent proficiently stabilize the acridine ring. The importance of electron withdrawal as the stabilizing factor is clearly seen in the comparison of where the para positioning of the ring nitrogen in d is better able to effect withdrawal (by supporting a negative charge in one mesomeric structure) in comparison to the meta positioned c: the H-10' tautomer of d is 1.25 kcal mol−1 more stable than the H-10' tautomer of c but the H-11' tautomer of d is only 0.12 kcal mol−1 more stable than the H-11' tautomer of c. Hence virtually all of the additional tautomeric stabilization of d emanates from its H-10' tautomer. Of significance, the tautomeric stability order of a < b < c < d reflects the afore described fluorescence order well with only b and c interchanged within the order. This is considered a correlation of some note and could be useful for adequately evaluating appropriate systems rapidly with regards to their potential applications, e.g. as fluorescence probes. Finally, calculation of the 13C NMR chemical shifts using the GIAO method at the DFT B3LYP/6-311++G(2d,2p) level of theory yielded a significant linear correlation when plotted against the experimental values for compounds 6a-e (linear equation: δexp = 0.95 × δcalc + 1.24, correlation coefficient r = 0.986) and 8a−d (linear equation: δexp = 0.98 × δcalc – 1.98, correlation coefficient r = 0.987), thereby substantiating the correctness of the assigned structures.
DNA binding properties
To evaluate the potential applications of 6c−e as biological probes and/or potential drug therapeutic agents based on their intercalating properties, we investigated their binding efficiency and chromophore
selective interactions with ctDNA using spectroscopic techniques. The UV-vis spectra of compounds 6c−e exhibited broad absorption bands in the region 375−475 nm, typical for transitions between the π-electronic energy levels of the acridine skeleton. Upon the addition of ctDNA to the solutions of 6c−e, a reduction in the absorbance by 28–33% from its initial value was observed which was dependent on the concentration of ctDNA. Figure 6 depicts these changes in the absorption spectra of 6c upon increasing concentrations of ctDNA.
The DNA binding constants K of 6c−e (Table 3) were determined using UV-vis titration according to the method of McGhee and von Hippel.19 Binding constants were found to be in the range 5.3−9.2 × 104 M−1
and the binding affinity values of compounds 6c−e with ctDNA were found to be similar to values for proflavine−dithiazolidinone derivatives which have been reported recently.20 The binding isotherms in each case were non-linear and concave upward, suggesting the involvement of more than one binding site. With multiple site binding (n lies in the range 2.1−3.4), the occurrence of one binding event is able to influence the interaction at neighboring sites. Binding constants and neighbor exclusion parameters clearly indicate a direct dependence between the intercalation capability and resulting structural changes in DNA binding. Upon the addition of ctDNA, the fluorescence properties of compounds 6c−e was also affected with a resulting decrease in their fluorescence. Similar biological testing of oxadiazoles 8a−d was precluded due to the decomposition of these compounds under Tris buffer conditions.
EXPERIMENTAL
Melting points were determined on a Boetius block and are uncorrected. NMR spectra were obtained at room temperature in hexadeuteriodimethyl sulfoxide (DMSO-d6) using a Varian Mercury Plus NMR spectrometer operating at 400 MHz for 1H and 100 MHz for 13C. Tetramethylsilane was used as an internal standard for both 1H and 13C nuclei (δTMS = 0.00 ppm for both). Heteronuclear HSQC and HMBC 2D experiments were optimized to 145 Hz (one-bond) and 8 Hz (long-range) for JH,C couplings. Elemental analyses were performed using a Perkin−Elmer CHN 2400 analyzer. IR spectra were recorded on a Nicolet 6700 FT IR instrument (samples in CS2 solution, due to consistency with previous azomethines11). UV-vis spectra were measured on a Varian Cary 100 UV-vis spectrophotometer in methanol whilst the UV-vis titrations were conducted in 10 mm Tris buffer at pH 7.3. The concentration of compounds 6a–e was 2.60 × 10−5 m and 8a–d was 2.17 × 10−5 m. Fluorescence measurements were made using a Varian Cary Eclipse spectrofluorometer with a slit width of 10 nm for the excitation and emission beams in methanol. The concentration of 6a–e was 1.25 × 10−5 m and that of 8a–d was 1.08 × 10−5 m. Fluorescence intensities are expressed in arbitrary units. The concentration of ctDNA (purchased from Sigma Chemical Co.) was varied over the range 0−80 µm. All measurements were performed at 24 °C. The absorption data were used to construct binding plots according to the method of McGhee and von Hippel using data points from a Scatchard plot.20,21 The binding data were fitted using GNU Octave 2.1.73 software. DFT quantum-chemical calculations were performed using the Gaussian 03 program at the B3LYP/6-311+G(d,p) level of theory.22,23 The GIAO method24 was used to obtain 13C NMR chemical shifts with the 6-311++G(2d,2p) basis set. UV-vis spectral analysis was carried out using ZINDO method.25
General procedure for the preparation of hydrazide 4
To an ethanolic solution of hydrazine monohydrate (0.82 mL, 15.85 mmol) an ethanolic solution of ester 3 (1 g, 3.95 mmol) was added. The reaction mixture was refluxed until the formation of a precipitant (ca. 4 h). The reaction mixture was then cooled and the precipitant filtered off and dried. Yield 80%; mp 314−316 °C; 1H NMR (400 MHz, DMSO-d6) δ: 4.72 (bs, 2H, NH2), 7.30−7.35 (m, 2H, H-2,7), 7.71−7.79 (m, 2H, H-5,6), 8.16 (dd, 1H, H-3, J = 8.4, 1.4 Hz), 8.24 (dd, 1H, H-8, J = 8.4, 1.2 Hz), 8.43 (dd, 1H, H-1, J = 9.6, 1.4 Hz), 10.26 (s, 1H, NH), 12.28 (s, 1H, H-10); 13C NMR (100 MHz, DMSO-d6) δ: 117.8 (C-4), 118.2 (C-5), 120.0 (C-2), 120.3 (C-9a), 121.42 (C-8a), 121.8 (C-7), 125.8 (C-8), 129.7 (C-1), 132.3 (C-3), 133.9 (C-6), 139.9 (C-4a), 139.9 (C-10a), 166.6 (C=O), 176.45 (C-9); Anal. Calcd for C14H11N3O2 (253.26): C, 66.40; H, 4.38; N, 16.59. Found: C, 66.95; H, 4.17; N, 16.23%.
Aldimines 5a−e were prepared according to the procedure described previously.11
General procedure for the preparation of oxadiazoles 6a−e
To a mixture of aldimine (0.26 mmol) and anhydrous sodium acetate (0.26 mmol) in glacial acetic acid bromine in glacial acetic acid (0.26 mmol) was added. The reaction mixture was stirred at rt for 2 h followed by the addition of acetic anhydride (1 mL) after which the reaction mixture was left to stir for 24 h at 100 °C. The reaction mixture was then dried under reduced pressure and extracted into CHCl3 following which the solvent was removed and the crude product recrystallized from MeOH.
4-(5-Phenyl[1,3,4]oxadiazol-2-yl)-10H-acridin-9-one (6a). Yield 65%; mp 227−230 °C; IR (CS2, hydrogen bondings) 3441.0 and 3443.2 cm−1; UV-vis λ = 411 nm (log ε = 3.67), λ = 299 nm (log ε = 3.79); 1H NMR (CDCl3) δ: 7.30 (t, 1H, H-7', J = 8.0 Hz), 7.36 (t, 1H, H-2', J = 7.6 Hz), 7.50 (d, 1H, H-5', J = 8.4 Hz), 7.58−7.62 (m, 3H, H-3'',4'',5''), 7.69 (m, 1H, H-6'), 8.17 (dd, 2H, H-2'',6'', J = 7.6, 1.6 Hz), 8.34 (d, 1H, H-3', J = 7.2 Hz), 8.44 (d, 1H, H-8', J = 7.6 Hz), 8.67 (d, 1H, H-1', J = 7.6 Hz), 11.81 (s, 1H, H-10'); 13C NMR (CDCl3) δ: 109.1 (C-4'), 117.7 (C-5'), 120.4 (C-2'), 121.6 (C-8a'), 122.4 (C-9a'), 122.6 (C-7'), 123.1 (C-1''), 127.0 (C-8'), 127.1 (C-3'',5''), 129.3 (2'',6''), 131.9 (C-1'), 132.3 (C-3'), 132.3 (C-4''), 134.1 (C-6'), 138.7 (C-4a'), 140.3 (C-10a'), 163.4 (C-2), 163.6 (C-5), 177.8 (C-9'). Anal. Calcd for C21H13N3O2 (339.36): C, 74.33; H, 3.86; N, 12.38. Found: C, 74.12; H, 3.78; N, 12.01%.
4-[5-(4-Bromophenyl)-[1,3,4]oxadiazol-2-yl]-10H-acridin-9-one (6b). Yield 37%; mp 229−232 °C; UV-vis λ = 413 nm (log ε = 4.18), λ = 390 nm (log ε = 3.99), λ = 320 nm (log ε = 4.23), λ = 298 nm (log ε = 4.21); 1H NMR (CDCl3) δ: 7.35−7.30 (m, 2H, H-2',7'), 7.42 (d, 2H, H-2'',6'', J = 8.4 Hz), 7.57 (d, 2H, H-3'',5'', J = 8.8 Hz), 7.78−7.70 (m, 2H, H-5',6'), 8.16 (d, 1H, H-3', J = 7.6 Hz), 8.47 (d, 1H, H-8', J = 8.4 Hz), 8.67 (d, 1H, H-1', J = 8.0 Hz), 11.04 (s, 1H, H-10'); 13C NMR (CDCl3) δ: 109.2 (C-4'), 117.2 (C-5'), 120.5 (C-2'), 121.7 (C-9a'), 122.1 (C-8a'), 122.8 (C-4''), 122.8 (C-7'), 124.6 (C-1''), 127.3 (C-8'), 128.3 (C-2'',6''), 132.1 (C-1'), 132.2 (C-3'',5''), 133.5 (C-3'), 134.1 (C-6'), 138.7 (C-4a'), 139.9 (C-10a'), 155.1 (C-5), 167.3 (C-2), 177.7 (C-9'). Anal. Calcd for C21H12BrN3O2 (418.25): C, 60.31; H, 2.89; N, 10.05. Found: C, 60.48; H, 2.85; N, 9.97%.
4-[5-(4-Methoxyphenyl)-[1,3,4]oxadiazol-2-yl]-10H-acridin-9-one (6c). Yield 40%; mp 226−228 °C; UV-vis λ = 412 nm (log ε = 4.28), λ = 392 nm (log ε = 4.14), λ = 322 nm (log ε = 4.31), λ = 311 nm (log ε = 4.37); 1H NMR (CDCl3) δ: 3.92 (s, 3H, OCH3), 7.07 (m, 2H, H-3'',5''), 7.32 (t, 1H, H-7', J = 7.4 Hz), 7.38 (t, 1H, H-2', J = 7.6 Hz), 7.52 (d, 1H, H-5', J = 8.4 Hz), 7.71 (t, 1H, H-6', J = 7.8 Hz), 8.11 (d, 2H, H-2'',6'', J = 9.2 Hz), 8.35 (dd, 1H, H-3', J = 7.2, 1.6 Hz), 8.46 (dd, 1H, H-8', J = 8.4, 1.6 Hz), 8.69 (dd, 1H, H-1', J = 8.4, 1.6 Hz), 11.87 (s, 1H, H-10'); 13C NMR (CDCl3) δ: 55.6 (OCH3), 109.4 (C-4'), 114.7 (C-3'',5''), 115.5 (C-1''), 117.8 (C-5'), 120.4 (C-2'), 121.7 (C-8a'), 122.4 (C-9a'), 122.6 (C-7'), 127.1 (C-8'), 128.9 (C-2'',6''), 131.7 (C-1'), 132.2 (C-3'), 134.1 (C-6'), 138.7 (C-4a'), 140.4 (C-10a'), 162.9 (C-4''), 163.0 (C-2), 163.7 (C-5), 177.9 (C-9'). Anal. Calcd for C22H15N3O3 (369.38): C, 71.54; H, 4.09; N, 11.38. Found: C, 71.08; H, 3.96; N, 11.02%.
4-[5-(2,4,6-Trimethylphenyl)-[1,3,4]oxadiazol-2-yl]-10H-acridin-9-one (6d). Yield 72%; mp 216−218 °C; IR (CS2, hydrogen bondings) 3414.7 and 3444.7 cm−1; UV-vis λ = 411 nm (log ε = 4.07), λ = 391 nm (log ε = 3.93), λ = 326 nm (log ε = 3.20), λ = 305 nm (log ε = 4.02); 1H NMR (CDCl3) δ: 2.38 (s, 3H, H-4'''), 2.39 (s, 6H, H-2''',6'''), 7.04 (s, 2H, H-3'',5''), 7.32−7.37 (m, 2H, H-2',7'), 7.56 (d, 1H, H-5', J = 8.0 Hz), 7.73 (m, 1H, H-6'), 8.30 (dd, 1H, H-3', J = 7.2, 1.4 Hz), 8.47 (dd, 1H, H-8', J = 8.0, 1.6 Hz), 8.70 (dd, 1H, H-1', J = 7.6, 1.4 Hz), 11.93 (s, 1H, H-10'); 13C NMR (CDCl3) δ: 20.7 (C-2''',6'''), 21.4 (C-4'''), 109.4 (C-4'), 117.8 (C-5'), 120.2 (C-1''), 120.5 (C-2'), 121.7 (C-8a'), 122.4 (C-9a'), 122.6 (C-7'), 127.1 (C-8'), 129.2 (C-3'',5''), 131.9 (C-1'), 132.4 (C-3'), 134.1 (C-6'), 138.8 (C-4a'), 138.9 (C-2'',6''), 140.4 (C-10a'), 141.7 (C-4''), 163.2 (C-5), 163.8 (C-2), 177.9 (C-9'). Anal. Calcd for C24H19N3O2 (381.44): C, 75.57; H, 5.02; N, 11.02. Found: C, 75.16; H, 4.91; N, 10.86%.
4-[5-(3-Nitrophenyl)-[1,3,4]oxadiazol-2-yl]-10H-acridin-9-one (6e). Yield 68%; mp 267−269 °C; IR (CS2, hydrogen bondings) 3413.6 and 3443.3 cm−1; UV-vis λ = 412 nm (log ε = 4.23), λ = 390 nm (log ε = 4.07), λ = 297 nm (log ε = 4.17); 1H NMR (CDCl3) δ: 7.23 (s, 1H, H-2''), 7.31−7.34 (m, 2H, H-2',7'), 7.66 (t, 1H, H-5'', J = 8.4 Hz), 7.73 (t, 1H, H-6', J = 7.6 Hz), 7.94 (d, 1H, H-4'', J = 8.4 Hz), 8.17 (dd, 1H, H-3', J = 7.2, 1.2 Hz), 8.32 (m, 1H, H-6''), 8.40 (m, 1H, H-5'), 8.48 (dd, 1H, H-8', J = 8.0, 1.2 Hz), 8.69 (dd, 1H, H-1', J = 7.2, 1.2 Hz), 10.98 (s, 1H, H-10'); 13C NMR (CDCl3) 108.9 (C-4'), 117.2 (C-2''), 120.6 (C-2'), 121.0 (C-1''), 121.7 (C-9a'), 121.8 (C-5'), 122.2 (C-8a'), 122.9 (C-7'), 125.2 (C-6''), 127.4 (C-8'), 130.2 (C-5''), 132.4 (C-1'), 132.9 (C-4''), 133.5 (C-3'), 134.2 (C-6'), 137.7 (C-3''), 138.8 (C-4a'), 139.9 (C-10a'), 155.1 (C-5), 167.7 (C-2), 177.7 (C-9'). Anal. Calcd for C21H12N4O4 (384.35): C, 65.63; H, 3.15; N, 14.58. Found: C, 65.21; H, 3.07; N, 14.25%.
General procedure for the synthesis of 1,3,4-oxadiazoles 8a−d
Method A: To a boiling solution of acylthiosemicarbazides 7a−d9b (0.4 mmol) in absolute EtOH (4 mL), finely powdered mercuric oxide (0.9 mmol) was added portion-wise over a period of 30 min. The resulting suspension was heated under reflux for 2 h and then filtered whilst hot. The black precipitate was washed with boiling EtOH (3 × 3 mL) and the combined filtrate and washings were concentrated to a small volume and set aside overnight at rt. The separated product was filtered, dried and recrystallized from MeOH.
Method B: A mixture of acylsemicarbazide (0.27 mmol) and POCl3 (0.95 mL) was heated on an oil bath until the reaction was complete (monitored by TLC, CHCl3–MeOH, 9:1). The reaction mixture was cooled to rt, diluted with CH2Cl2 (8 mL) and then poured into 25 mL of an ice–water mixture with stirring. After cooling to rt and extraction with a mixture of CH2Cl2−MeOH (1:1), the combined organic extracts were washed with H2O, brine, and then dried over MgSO4. The solvent was evaporated in vacuo and the residue purified by flash chromatography (silica gel, CHCl3−MeOH).
(10H-Acridin-9-ylidene)-(5-methyl[1,3,4]oxadiazol-2-yl)amine (8a). Yield 13%; mp 222−224 °C; UV-vis λ = 423 nm (log ε = 4.13), λ = 394 nm (log ε = 3.89), λ = 265 nm (log ε = 4.45); 1H NMR (DMSO-d6) δ: 2.42 (s, 3H, CH3), 7.22 (dd, 2H, H-2',7', J = 8.0, 7.0 Hz), 7.58 (d, 2H, H-4',5', J = 8.4 Hz), 7.73 (dd, 2H, H-3',6', J = 8.4, 7.0 Hz), 7.90 (d, 2H, H-1',8', J = 8.0 Hz), 12.09 (s, 1H, NH); 13C NMR (DMSO-d6) δ: 11.0 (CH3), 117.1 (C-8a',9a'), 117.7 (C-4',5'), 121.7 (C-2',7'), 126.4 (C-1',8'), 133.4 (C-3',6'), 139.7 (C-4a',10a'), 159.7 (C-5), 159.8 (C-9'), 166.1 (C-2). Anal. Calcd for C16H12N4O (276.30): C, 69.55; H, 4.38; N, 20.28. Found: C, 69.28; H, 4.17; N, 20.03%.
(10H-Acridin-9-ylidene)-(5-phenyl[1,3,4]oxadiazol-2-yl)amine (8b). Yield 12%; mp 297−300 °C; UV-vis λ = 419 nm (log ε = 4.04), λ = 389 nm (log ε = 3.86); 1H NMR (DMSO-d6) δ: 7.25 (ddd, 2H, H-2',7', J = 8.2, 7.0, 1.2 Hz), 7.50−7.60 (m, 3H, H-2'',4'',6''), 7.63 (dd, 2H, H-4',5', J = 8.2, 1.2 Hz), 7.77 (ddd, 2H, H-3',6', J = 8.2, 7.0, 1.2 Hz), 7.85−7.95 (m, 2H, H-3'',5''), 8.01 (dd, 2H, H-1',8', J = 8.2, 1.2 Hz), 12.28 (s, 1H, NH); 13C NMR (DMSO-d6) δ: 117.6 (C-8a',9a'), 118.3 (C-4',5'), 122.4 (C-2',7'), 124.8 (C-1''), 126.0 (C-3'',5''), 126.9 (C-1',8'), 129.8 (C-2'',6''), 131.4 (C-4''), 134.1 (C-3',6'), 140.2 (C-4a',10a'), 160.7, 160.8 (C-5, C-9'), 166.6 (C-2); Anal. Calcd for C21H14N4O (338.37): C, 74.54; H, 4.17; N, 16.56. Found: C, 74.26; H, 3.98; N, 16.37%.
(10H-Acridin-9-ylidene)-(5-pyridin-3-yl[1,3,4]oxadiazol-2-yl)amine (8c). Yield 15%; mp 292−294 °C; UV-vis λ = 427 nm (log ε = 3.99), λ = 400 nm (log ε = 3.78), λ = 269 nm (log ε = 4.33); 1H NMR (DMSO-d6) δ: 7.26 (dd, 2H, H-2',7', J = 8.0, 7.2 Hz), 7.59 (dd, 1H, H-5'', J = 8.0, 4.8 Hz), 7.65 (d, 2H, H-4',5', J = 8.0 Hz), 7.78 (ddd, 2H, H-3',6', J = 8.0, 7.2, 1.2 Hz), 8.05 (dd, 2H, H-1',8', J = 8.0, 1.2 Hz), 8.27 (ddd, 1H, H-4'', J = 8.0, 2.0, 1.6 Hz), 8.74 (dd, 1H, H-6'', J = 4.8, 1.6 Hz), 9.09 (d, 1H, H-2'', J = 2.0 Hz), 12.35 (s, 1H, NH); 13C NMR (DMSO-d6) δ: 117.0 (C-8a',9a'), 117.8 (C-4',5'), 120.8 (C-3''), 121.9 (C-2',7'), 124.2 (C-5''), 126.4 (C-1',8'), 132.9 (C-4''), 133.6 (C-3',6'), 139.7 (C-4a',10a'), 146.2 (C-2''), 151.3 (C-6''), 158.3 (C-5), 160.4 (C-9'), 166.4 (C-2); Anal. Calcd for C20H13N5O (339.36): C, 70.79; H, 3.86; N, 20.64. Found: C, 70.54; H, 3.69; N, 20.41%.
(10H-Acridin-9-ylidene)-(5-pyridin-4-yl[1,3,4]oxadiazol-2-yl)amine (8d). Yield 12%; mp 324–326 °C; UV-vis λ = 429 nm (log ε = 3.74), λ = 390 nm (log ε = 3.53); 1H NMR (DMSO-d6) δ: 7.27 (dd, 2H, H-2',7', J = 8.0, 7.2 Hz), 7.66 (d, 2H, H-4',5', J = 8.4 Hz), 7.70−7.90 (m, 4H, H-3',6',3'',5''), 8.04 (d, 2H, H-1',8', J = 8.0 Hz), 8.76 (m, 2H, H-2'',6''), 12.41 (s, 1H, NH); 13C NMR (DMSO-d6) δ: 117.5 (C-8a',9a'), 118.4 (C-4',5'), 119.7 (C-3'',5''), 122.6 (C-2',7'), 126.9 (C-1',8'), 131.7 (C-4''), 134.3 (C-3',6'), 140.2 (C-4a',10a'), 151.2 (C-2'',6''), 159.0 (C-5), 161.1 (C-9'), 167.3 (C-2); Anal. Calcd For C20H13N5O (339.36): C, 70.79; H, 3.86; N, 20.64. Found: C, 70.47; H, 3.62; N, 20.45%.
4-(9,10-Dihydroacridin-9-ylidene)-1-(4-pyridinylcarbonyl)semicarbazide (9d). To a solution of acylthiosemicarbazide 7d (0.40 mmol) in MeCN (2 mL), MNO (0.49 mmol) was added and the reaction mixture stirred at rt until the reactants had been consumed (monitored by TLC, CHCl3–MeOH, 9:1). The precipitate that formed was filtered off, washed with MeCN and dried at rt. The crude product was recrystallized from MeOH. Yield 95%; mp 154–156 °C; 1H NMR (DMSO-d6) δ: 7.17 (dd, 1H, H-2', J = 8.0, 7.4 Hz), 7.43 (d, 1H, H-4', J = 8.0 Hz), 7.64 (dd, 2H, H-3',6', J = 8.0, 7.4 Hz), 7.80−8.00 (m, 3H, H-3'',5'',7'), 8.16 (d, 1H, H-8', J = 8.0 Hz), 8.25 (d, 1H, H-5', J = 8.0 Hz), 8.40 (d, 1H, H-1', J = 8.0 Hz), 8.75−8.85 (m, 2H, H-2'',6''), 9.52 (s, 1H, NH), 10.64 (s, 1H, NH), 11.44 (s, 1H, H-10'); 13C NMR (DMSO-d6) δ: 117.3 (C-4'), 117.6 (C-8a'), 121.4 (C-2'), 121.9 (C-3'',5''), 122.1 (C-9a'), 125.2 (C-5'), 126.0, 133.0 (C-3', C-6'), 128.0 (C-1'), 129.6 (C-8'), 130.8 (C-7'), 140.1, 140.4 (C-4a', C-4''), 149.4 (C-10a'), 150.8 (C-2'',6''), 154.3 (C-9'), 164.3 (C-3), 165.4 (Py−C=O); Anal. Calcd For C20H15N5O2 (357.37): C, 67.22; H, 4.23; N, 19.60. Found: C, 66.97; H, 4.06; N, 19.33%.
ACKNOWLEDGEMENTS
Financial support for this work from the Slovak Grant Agency VEGA (grants no. 1/0476/08, 1/0053/08), the State NMR Program (grant no. 2003SP200280203), and Turun Yliopistosäätiö is gratefully acknowledged.
† Dedicated to Professor Emeritus Keiichiro Fukumoto on the occasion of his 75th birthday.
The author to whom correspondence should be addressed.
References
1. S. A. F. Rostom, M. A. Shalaby, and M. A. El-Demellawy, Eur. J. Med. Chem., 2003, 38, 959. CrossRef
2. S. G. Küçükgüzel, E. E. Oruç, S. Rollas, F. S. Sahin, and A. Özbek, Eur. J. Med. Chem., 2002, 37, 197. CrossRef
3. P. Mishra, H. Rajak, and A. Mehta, J. Gen. Appl. Microbiol., 2005, 51, 133. CrossRef
4. K. M. Khan, Zia-Ullah, M. Rani, S. Perveen, S. M. Haider, M. I. Choudhary, Atta-ur-Rahman, and W. Voelter, Lett. Org. Chem., 2004, 1, 50. CrossRef
5. I. R. Baxendale, S. V. Ley, and M. Martinelli, Tetrahedron, 2005, 61, 5323 and references cited therein. CrossRef
6. M. A. M. Taha and S. M. El-Badry, J. Chin. Chem. Soc., 2006, 53, 1181 and references cited therein.
7. G. S. Poindexter, M. A. Bruce, J. G. Breitenbucher, M. A. Higgins, S.-Y. Sit, J. L. Romine, S. W. Martin, S. A. Ward, R. T. McGovern, W. Clarke, J. Russell, and I. Antal-Zimanyi, Bioorg. Med. Chem., 2004, 12, 507. CrossRef
8. W. Ogilvie and W. Rank, Can. J. Chem., 1987, 65, 166. CrossRef
9. a) D. Sabolová, M. Kozurková, P. Kristian, I. Danihel, D. Podhradsky, and J. Imrich, Int. J. Biol. Macromol., 2006, 38, 94; CrossRef b) J. Tomasciková, J. Imrich, I. Danihel, S. Böhm, P. Kristian, J. Pisarcíková, M. Sabol, and K. D. Klika, Molecules, 2008, 13, 501; CrossRef c) M. Kozurková, D. Sabolová, H. Paulíková, L. Janovec, P. Kristian, M. Bajdichová, J. Busa, D. Podhradsky, and J. Imrich, Int. J. Biol. Macromol., 2007, 41, 415; CrossRef d) M. Kozurková, D. Sabolová, L. Janovec, J. Mikes, J. Koval’, J. Ungvarsky, M. Stefanisinová, P. Fedorocko, P. Kristian, and J. Imrich, Bioorg. Med. Chem., 2008, 16, 3976; CrossRef e) Z. Gazová, A. Bellová, Z. Daxnerová, J. Imrich, P. Kristian, J. Tomasciková, J. Bágel’ová, D. Fedunová, and M. Antalík, Eur. Biophys. J., 2008, 37, 1261; CrossRef f) S. Hamul’aková, P. Kristian, D. Jun, K. Kuca, J. Imrich, I. Danihel, S. Böhm, and K. D. Klika, Heterocycles, 2008, 76, in press. CrossRef
10. V. Sourdon, S. Mazoyer, V. Pique, and J.-P. Galay, Molecules, 2001, 6, 677. CrossRef
11. Z. Fröhlichová, J. Imrich, I. Danihel, P. Kristian, S. Böhm, D. Sabolová, O. Hritzová, B. Horváth, T. Busová and K. D. Klika, submitted to Spectroc. Acta Pt. A, 2008.
12. Z. Shang, Synthetic Commun., 2006, 36, 2927. CrossRef
13. M. Dabiri, P. Salehi, M. Baghbanzadeh, and M. Bahramnejad, Tetrahedron Lett., 2006, 47, 6983. CrossRef
14. F. N. Hayes, B. S. Rogers, and D. G. Ott, J. Am. Chem. Soc., 1955, 77, 1850. CrossRef
15. D. R. Romer, R. B. Shankar, and R. G. Pews, PCT/US 1995/013493.
16. a) K. D. Klika, E. Balentová, J. Bernát, J. Imrich, M. Vavrusov&Aaacute;, E. Kleinpeter, K. Pihlaja, and A. Koch, J. Heterocycl. Chem., 2006, 43, 633; CrossRef b) E. Balentová, J. Imrich, J. Bernát, L. Suchá, M. Vilková, N. Prónayová, P. Kristian, K. Pihlaja, and K. D. Klika, J. Heterocycl. Chem., 2006, 43, 645; CrossRef c) K. D. Klika, E. Balentová, J. Bernát, J. Imrich, M. Vavrusová, K. Pihlaja, A. Koch, E. Kleinpeter, A. Kelling, and U. Schilde, ARKIVOC, 2006, (xvi), 93; d) J. Bernát, E. Balentová, P. Kristian, J. Imrich, E. Sedlák, I. Danihel, S. Böhm, N. Prónayová, K. Pihlaja, and K. D. Klika, Coll. Czech. Chem. Commun., 2004, 69, 833; CrossRef e) K. D. Klika, J. Imrich, M. Vilková, J. Bernát, and K. Pihlaja, J. Heterocycl. Chem., 2006, 43, 739; CrossRef f) K. D. Klika, J. Bernát, J. Imrich, I. Chomca, R. Sillanpää, and K. Pihlaja, J. Org. Chem., 2001, 66, 4416; CrossRef g) P. Kristian, E. Balentová, J. Bernát, J. Imrich, E. Sedlák, I. Danihel, S. Böhm, N. Prónayová, K. D. Klika, K. Pihlaja, and J. Baranová, Chem. Pap., 2004, 58, 268; h) B. Adcock, ‘Aminoacridines’ In ‘Acridines’, ed. by R. M. Acheson, (from ‘The Chemistry of Heterocyclic Compounds’ ed. by A. Weissberger and E. C. Taylor), J. Wiley: New York, 1973, p. 109.
17. P. Kristian, J. Bernát, J. Imrich, E. Sedlák, J. Alföldi, and M. Cornanic, Heterocycles, 2001, 55, 279. CrossRef
18. A. Souldozi, K. Slepokura, T. Lis, and A. Ramazani, Z. Naturforsch. (B), 2007, 62, 835.
19. J. McGhee and P. von Hippel, J. Mol. Biol., 1974, 86, 469. CrossRef
20. L. Janovec, D. Sabolová, M. Kozurková, H. Paulíková, P. Kristian, J. Ungvarsky, E. Moravcíková, M. Bajdichová, D. Podhradsky, and J. Imrich, Bioconjug. Chem., 2007, 18, 93. CrossRef
21. T. C. Jenkins, Methods in Molecular Biology, K. R. Fox, Humana Press, Totowa, New Jersey, 1997, 90, 195.
22. A. D. Becke, J. Chem. Phys., 1993, 98, 5648. CrossRef
23. M. J. Frisch, G. W. Trucks, H. B. Schlegel, G. E. Scuseria, M. A. Robb, J. R. Cheeseman, J. A. Montgomery Jr., T. Vreven, K. N. Kudin, J. C. Burant, J. M. Millam, S. S. Iyengar, J. Tomasi, V. Barone, B. Mennucci, M. Cossi, G. Scalmani, N. Rega, A. Petersson, H. Nakatsuji, M. Hada, M. Ehara, K. Toyota, R. Fukuda, J. Hagesawa, M. Ishida, T. Nakajima, Y. Honda, O. Kitao, H. Nakai, M. Klene, X. Li, J. E. Knox, H. P. Hratchian, J. B. Cross, C. Adamo, J. Jaramillo, R. Gomperts, E. Stratmann, O. Yazyev, A. J. Austin, R. Cammi, C. Pomelli, J. W. Ochterski, P. Y. Ayala, K. Morokuma, G. A. Voth, P. Salvador, J. J. Dannenberg, V. G. Zakrzewski, S. Dapprich, A. D. Daniels, M. C. Strain, O. Farkas, D. K. Malick, A. D. Rabuck, K. Raghavachari, J. B. Foresman, J. V. Ortiz, Q. Cui, A. G. Baboul, S. Clifford, J. Cioslowski, B. B. Stefanov, G. Liu, A. Liashenko, P. Piskorz, I. Komaromi, R. L. Martin, D. J. Fox, T. Keith, M. A. Al-Laham, C. Y. Peng, A. Nanayakkara, M. Challacombe, P. M. W. Gill, B. Johnson, W. Chen, M. W. Wong, C. Gonzales, and J. A. Pople, Gaussian 03, Revision B.05, Gaussian, Inc., Pittsburgh PA 2003.
24. K. Wolinski, J. F. Hinton, and P. Pulay, J. Am. Chem. Soc., 1990, 112, 8251. CrossRef
25. L. K. Hanson, J. Fajer, M. A. Thompson, and M. C. Zerner, J. Am. Chem. Soc., 1987, 109, 4728 CrossRef