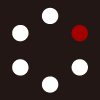
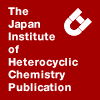
HETEROCYCLES
An International Journal for Reviews and Communications in Heterocyclic ChemistryWeb Edition ISSN: 1881-0942
Published online by The Japan Institute of Heterocyclic Chemistry
e-Journal
Full Text HTML
Received, 31st July, 2008, Accepted, 23rd October, 2008, Published online, 27th October, 2008.
DOI: 10.3987/COM-08-S(F)84
■ Preparation of 1,4-Bis[2-{2-(1-methyl-1H-imidazol-5-yl)ethynyl}-3-thienyl]benzene and Related Compounds
Kozo Toyota,* Kazuyuki Okada, Hiroshi Katsuta, Yasutomo Tsuji, and Noboru Morita
Department of Chemistry, Graduate School of Science, Tohoku University, Aoba-ku, Sendai 980-8578, Japan
Abstract
1,4-Bis[2-{2-(1-methyl-1H-imidazol-5-yl)ethynyl}-3-thienyl]benzene, 1,4-bis[2-(2-ferrocenylethynyl)-3-thienyl]benzene, and some related compounds were prepared and their UV-visible spectra were studied.INTRODUCTION
Transition metal complexes of ligands containing multiple coordination sites have been the focus of considerable research interest for many decades.1 Recently, artificial molecular architecture has also attracted considerable interest.2 Toyota and coworkers previously designed and prepared a double bonded phosphorus ligand, DPCBT (Chart 1),3 and related polymers4 and metal complexes,3c,4,5 containing thiophene moieties, which connect the various substituents. The key to this research is that the coordination sites are separated by appropriate spacer units. In the case of DPCBT polymers,4 the thiophene moieties perform this role by connecting the diphosphinidenecyclobutene ligand units. During this earlier study, the author was inspired by metalloprotein structures, and designed ETB (Chart 1, n = 1) and ETAr (n ≥ 2) spacers,6 which contain thiophene rings bearing side chains. The expectation was that the ETB/ETAr spacers could be used to connect various substituents, such as pyridyl, imidazolyl, cyclopentadienyl, and diphenylphosphino units, at the sp-hybridized carbons. Based on this idea, we have prepared several compounds such as 1–4 and 5a,6 containing the ETB or ETAr spacer. The ETB/ETAr spacers enable the easy and selective introduction of substituents at the terminal sp-carbon and/or the 5-position of the thiophene rings and, therefore, provide a promising potential building block for the construction of sophisticated ligands, Chart 1.
Our linked ETAr system concept, Chart 1, is very much bio-inspired. Proteins (especially, transmembrane proteins) normally have both a hydrophobic and a hydrophilic domain, and exhibit special functionality such as catalytic (or enzymatic) activity. Our synthetic strategy involves connecting ETAr units to hydrophobic and/or hydrophilic chains, to enable the preparation of large assembled molecules in water. As part of the fundamental research building towards that goal, we report here the preparation of a novel ligand and an organometallic compound of the ETB type, containing either a 1-methyl-1H-imidazol-5-yl group or a ferrocenyl group.
RESULTS AND DISCUSSION
1,4-Bis[2-{2-(1-methyl-1H-imidazol-5-yl)ethynyl}-3-thienyl]benzene (8) was prepared by a Sonogashira coupling of 1,4-bis(2-iodo-3-thienyl)benzene (7) with 5-ethynyl-1-methyl-1H-imidazole in 70% yield, Scheme 1. A (3-thienyl)ethynyl-substituted derivative, 5b, (Chart 2) and a ferrocenylethynyl-substituted derivative, 11, were prepared under similar conditions in 77% and 22% yield, respectively. In addition, a mono-substituted derivative was also prepared. 1,4-Di(3-thienyl)benzene (6, 1 molar amount) was reacted with N-iodosuccinimide (NIS, ca. 0.5 molar amount) to afford a mixture of 6, 7, and 9 (ca. 1:2:8 ratio). A Sonogashira coupling reaction of 9 (crude) with 5-ethynyl-1-methyl-1H-imizazole yielded compound 10.
It should be noted that we previously prepared the mono-ethynyl compound 12 based on a Suzuki-Miyaura coupling of 3-thiopheneboronic acid with the 1-bromo-4-(2-ethynyl-3-thienyl)benzene derivative.6c As described above, selective mono-iodination of 6 turned out to be difficult. Thus, for the purpose of preparing mono-ethynyl derivatives, the previous strategy using 1-bromo-4-(2-ethynyl-3-thienyl)benzene derivative seems to be more advantageous than using 9.
Figure 1 contains the UV-visible spectra of 8 and the related 2, 3a, 5a,b compounds in CH2Cl2. Of the five compounds, the phenylethynyl derivative 2 exhibits the highest ε value in the 250–370 nm region, while those of 8 tend to be the smallest (similar results were obtained in the case of 10, see below). As expected, there is a bathochromic shift of the spectrum of (2-thienyl)ethynyl derivative 5a, compared to that of 5b.
Figure 2 contains the UV-visible spectra of the mono-ethynyl substituted derivatives 10 and 12 in CH2Cl2, along with those of 8 and 13.6b The diethynyl derivatives 8 and 13 exhibit bathochromic shifts (ca. 15 nm shift) and larger ε values, relative to 10 and 12 due to significant π-conjugation. The molar extinction coefficients of the imidazolyl compounds are smaller than those of the corresponding p-butylphenyl and p-hexylphenyl derivatives. This is probably due to a lower degree of coplanarity between the N-methylimidazole ring and the ethynylthiophene moiety in the case of 8 and 10, due to the steric congestion between the methyl group and the central benzene ring upon rotation around the imidazole–ethynyl bond. Additional evidence for this steric congestion is provided by the 1H and 13C NMR spectra. This is probably due to hindered rotation at the imidazole–ethynyl bond. It should be noted, however, that there is no significant signal broadening of the benzene moiety.
Figure 3 contains the absorption spectra of 8, 11, and 13 in CH2Cl2. Derivative 11 primarily absorbs between 400–550 nm due to the d-d transition of the ferrocene moiety, as has been observed previously in the case of 2,5-bis(ferrocenylethynyl)thiophene [λmax (log ε) in CH2Cl2: 340 (4.43) and 445 (3.53)].7
In summary, we have prepared several ETB derivatives, containing imidazolyl or cyclopentadienyl ligand moieties. These derivatives may find practical application as functional units particularly in the context of catalysis. Further studies on the construction of linked ETAr systems are in progress.
EXPERIMENTAL
Melting points were measured on a Yanagimoto MP-J3 micro melting point apparatus and are uncorrected. NMR spectra were recorded on a Bruker Avance-400 or AM-600 spectrometer. UV-visible spectra were measured on a Hitachi U-3210 spectrometer, while a Horiba FT-300 or a Shimadzu FTIR-8100M spectrometer was used to obtain the IR spectra. A Hitachi M-2500S spectrometer was used to obtain MS data. FT-ICR-MS spectra were measured on a Bruker APEX III spectrometer.
1,4-Bis[2-{2-(3-thienyl)ethynyl}-3-thienyl]benzene (5b). A mixture of 7 (522 mg, 1.06 mmol), 3-ethynylthiophene (294 mg, 2.72 mmol), dichlorobis(triphenylphosphine)palladium(II) (106 mg, 0.150 mmol), copper(I) iodide (14.4 mg, 0.0756 mmol), and diisopropylamine (1.2 mL) in THF (16 mL) was stirred at 50 °C for 40 h. CHCl3 and water were added to the resulting mixture. The organic phase was separated, dried over MgSO4 and the solvent was removed under reduced pressure. The residue was treated on a silica-gel column (eluent = hexane-EtOAc 1:0 to 10:1) to give 370 mg (0.813 mmol, 77% yield) of 5b and 47.1 mg (9% recovery) of the starting material, 7. 5b: Pale yellow powder, mp 165–170 °C (decomp); Rf = 0.45 (SiO2-CCl4); 1H NMR (400 MHz, CDCl3) δ = 7.13 (2H, dd, J = 5.2 and 0.8 Hz, 4’-thienyl), 7.24–7.26 (2H+2H, m, 4- and 5’-thienyl), 7.30 (2H, d, J = 5.2 Hz, 5-thienyl), 7.45 (2H, dd, J = 2.8 Hz and 0.8 Hz, 2’-thienyl), and 7.90 (4H, s, phenyl); 13C{1H} NMR (100 MHz, CDCl3) δ = 82.7 (C≡C), 90.6 (C≡C), 118.2 (thienyl), 122.1 (thienyl), 125.5 (thienyl), 126.5 (thienyl), 127.9 (thienyl), 127.9 (phenyl), 128.7 (thienyl), 129.6 (thienyl), 134.6 (1- and 4-phenyl), and 144.1 (3-thienyl); UV (CH2Cl2) 297 (log ε 4.56), 319 (sh, 4.50), and 337 nm (sh, 4.44); IR (KBr) 3106, 2924, 2203 (C≡C), 1495, 1458, 1354, 1287, 1194, 1075, 930, 870, 845, 779, 731, 691, 648, 623, 538, and 519 cm–1. FT-ICR-MS Calcd for C26H14NaS4: M++Na, 476.9871. Found: m/z 476.9869. Anal. Calcd for C26H14S4: C, 68.69; H, 3.10%. Found: C, 68.29; H, 3.30%.
1,4-Bis[2-{2-(1-methyl-1H-imidazol-5-yl)ethynyl}-3-thienyl]benzene (8). A mixture of 7 (443 mg, 0.896 mmol), 5-ethynyl-1-methyl-1H-imidazole (247 mg, 2.33 mmol), dichlorobis(triphenylphosphine)palladium(II) (90 mg, 0.13 mmol), copper(I) iodide (13 mg, 0.066 mmol), and diisopropylamine (1.1 mL) in THF (14 mL) was stirred at 50 °C for 24 h. CHCl3 and water were added to the reaction mixture. The organic phase was washed with brine, dried over MgSO4, and the solvent was evaporated under reduced pressure. The residue was treated with an alumina column (eluent = EtOAc:EtOH 1:1) and the solvent of the elute was removed under reduced pressure. Recrystallization of the crude product from CHCl3-EtOH afforded 387 mg (0.625 mmol, 70% yield) of 8: Yellow solid, mp 216–218 °C (decomp); Rf = 0.50 (long tailing) (Al2O3-EtOAc); 1H NMR (400 MHz, CD2Cl2) δ = 3.60 (6H, s, Me), 7.26 (2H, d, J = 5.6 Hz, 4-thienyl), 7.33 (2H, br s, 4-imidazolyl), 7.37 (2H, d, J = 5.6 Hz, 5-thienyl), 7.44 (2H, br s, 2-imidazolyl), and 7.84 (4H, s, phenyl); 13C{1H} NMR (100 MHz, CDCl3) δ = 32.2 (br s, Me), 82.9 (br s, C≡C), 90.0 (C≡C), 116.1 (br s, imidazolyl), 117.5 (2-thienyl), 127.4 (thienyl), 128.0 (thienyl), 128.0 (2-,3-,5-, and 6-phenyl), 134.7 (1- and 4-phenyl), 135.0 (br s, imidazolyl), 138.7 (br s, imidazolyl), and 144.6 (3-thienyl); UV (CH2Cl2) 294 (log ε 4.49) and 337 nm (sh, 4.37); IR (KBr) 3114, 2197 (C≡C), 1653, 1559, 1534, 1489, 1468, 1435, 1416, 1375, 1348, 1291, 1262, 1229, 1119, 1092, 924, 872, 849, 830, 758, 727, 695, 658, 639, and 542 cm–1. FT-ICR-MS Calcd for C26H19N4S2: M++H 451.1046. Found: m/z 451.1047. Anal. Calcd for C26H18N4S2·(H2O)2.5: C, 63.00; H, 4.68; N, 11.30%. Found: C, 63.01; H, 4.03; N, 12.03%.
1-[2-{2-(1-Methyl-1H-imidazol-5-yl)ethynyl}-3-thienyl]-4-(3-thienyl)benzene (10). A suspension of 6 (504 mg, 2.06 mmol), N-iodosuccinimide (262 mg, 1.16 mmol, 0.57 molar ratio), and acetic acid (13 mL) in CHCl3 (30 mL) was stirred at 50 °C for 3 h. The insoluble material was removed by filtration and the filtrate was treated with saturated aqueous NaHCO3 and then saturated aqueous Na2S2O3 solution. The organic phase was separated, dried over MgSO4, and the solvent was removed under reduced pressure. The residue was treated with a silica-gel column (eluent = CHCl3) to give a mixture (164 mg) of 6, 7, and 9 (ca. 1:2:8 ratio, determined by 1H NMR spectroscopy). Separation of the mixture by silica gel column chromatography is difficult due to the poor solubility and similar Rf values. 9: 1H NMR (400 MHz, CDCl3) δ = 6.99 (1H, d, J = 5.4 Hz, 4-thienyl), 7.41 (1H, dd, J = 5.0 Hz and 2.9 Hz, 5’-thienyl), 7.44 (1H, dd, J = 5.0 Hz and 1.4 Hz, 4’-thienyl), 7.50–7.52 (1H, m, 2’-thienyl), 7.51 (1H, d, J = 5.4 Hz, 5-thienyl), 7.56 (2H, AA’BB’, phenyl), and 7.67 (2H, AA’BB’, phenyl).
5-Ethynyl-1-methyl-1H-imidazole (0.068 mL, 0.67 mmol) was added to a mixture of crude 9, dichlorobis(triphenylphosphine)palladium(II) (48 mg, 0.069 mmol), copper(I) iodide (6 mg, 0.03 mmol), and diisopropylamine (8 mL) in THF (10 mL). The resulting mixture was stirred at 50 °C for 20 h. CHCl3 and water were added to the reaction mixture and the organic phase was washed with brine, separated, dried over MgSO4, and the solvent was evaporated under reduced pressure. The residue was treated with recycling gel permeation liquid chromatography (Japan Analytical Industry, JAIGEL H1+H2 column) to give 48 mg (0.14 mmol) of 10 (7% yield based on 6): Pale yellow oil; Rf = 0.84 (Al2O3-EtOAc); 1H NMR (600 MHz, CDCl3) δ = 3.64 (3H, s, Me), 7.25 (1H, d, J = 5.3 Hz, 4-thienyl), 7.34 (1H, br s, 4-imidazolyl), 7.35 (1H, d, J = 5.3 Hz, 5-thienyl), 7.40 (1H, dd, J = 5.0 Hz and 2.8 Hz, 5’-thienyl), 7.42 (1H, dd, J = 5.0 Hz and 1.5 Hz, 4’-thienyl), 7.47 (1H, br s, 2-imidazolyl), 7.50 (1H, dd, J = 2.8 Hz and 1.5 Hz, 2’-thienyl), 7.65 (2H, AA’BB’, phenyl), and 7.81 (2H, AA’BB’, phenyl); 13C{1H} NMR (150 MHz, CDCl3) δ = 32.3 (br s, Me), 82.9 (br s, C≡C), 90.0 (C≡C), 116.2 (br s, imidazolyl), 117.2 (thienyl), 126.2 (thienyl), 126.4 (thienyl and phenyl), 127.2 (thienyl), 128.0 (thienyl), 128.3 (phenyl), 134.0 (phenyl), 135.0 (br s, imidazolyl), 135.2 (phenyl), 138.6 (br s, imidazolyl), 141.7 (thienyl), and 144.7 (thienyl); UV (CH2Cl2) 279 (log ε 4.42) and 334 nm (sh, 4.26); IR (neat) 3096, 2923, 2851, 2203 (C≡C), 1539, 1493, 117, 924, 870, 845, 785, 739, 658, 633, and 525 cm–1. FT-ICR-MS Calcd for C18H15N2S2: M++H 347.0671. Found: m/z 347.0671.
1,4-Bis[2-(2-ferrocenylethynyl)-3-thienyl]benzene (11). A mixture of 7 (200 mg, 0.405 mmol), ethynylferrocene (213 mg, 1.01 mmol), dichlorobis(triphenylphosphine)palladium(II) (41 mg, 0.058 mmol), copper(I) iodide (6 mg, 0.03 mmol), and diisopropylamine (0.5 mL) in THF (6.0 mL) was stirred at 50 °C for 24 h. 11 (60 mg, 0.091 mmol, 22% yield) is insoluble and was obtained by filtration and washed with a large amount of CHCl3. 11: Yellow solid, mp 175–180 °C (decomp); 1H NMR (400 MHz, CD2Cl2) δ = 4.21 (10H, s, Fc), 4.25 (4H, s, Fc), 4.48 (4H, s, Fc), 7.27 (2H, d, J = 5.2 Hz, 4-thienyl), 7.29 (2H, d, J = 5.2 Hz, 5-thienyl), and 7.96 (4H, s, phenyl); UV (CH2Cl2) 260 (log ε 4.55), 290 (4.56), 344 (sh, 4.35), and 440 nm (3.32); IR (KBr) 3104, 2923, 2851, 2193 (C≡C), 1684, 1507, 1412, 1375, 1290, 1202, 1107, 1090, 1028, 1001, 916, 868, 845, 820, 727, 648, 534, 492, and 469 cm–1. FT-ICR-MS Calcd for C38H26Fe2NaS2: M++Na 681.0067. Found: m/z 681.0063. Anal. Calcd for C38H26Fe2S2·(H2O)2.5: C, 64.88; H, 4.44%. Found: C, 64.42; H, 4.00%. The 13C NMR spectrum was not measured due to the low solubility.
ACKNOWLEDGEMENTS
This work was supported in part by a Grant-in-Aid for Scientific Research (No. 20550030) from the Ministry of Education, Culture, Sports, Science and Technology.
† Dedicated to Professor Emeritus Keiichiro Fukumoto.
References
1. See, for example: ‘Metal Complexes and Metals in Macromolecules’, ed. by D. Wöhrle and A. D. Pomogailo, Wiley-VCH, Weinheim, 2003.
2. a) A. C. Reed, Acc. Chem. Res., 2005, 38, 215, and papers in this issue of the journal. For recent reports on oligophenylene rods, see for example; CrossRef b) A. L. Sisson, N. Sakai, N. Banerji, A. Fürstenberg, E. Vauthey, and S. Matile, Angew. Chem. Int. Ed., 2008, 47, 3727; CrossRef c) K. Tsubaki, K. Takaishi, D. Sue, K. Matsuda, Y. Kanemitsu, and T. Kawabata, J. Org. Chem., 2008, 73, 4279; CrossRef d) J.-H. Ryu, D.-J. Hong, and M. Lee, Chem. Commun., 2008, 1043, and references cited therein. CrossRef
3. a) K. Toyota, K. Abe, K. Horikawa, and M. Yoshifuji, Bull. Chem. Soc. Jpn., 2004, 77, 1377; CrossRef b) K. Toyota, K. Horikawa, R. S. Jensen, K. Omori, S. Kawasaki, S. Ito, M. Yoshifuji, and N. Morita, Bull. Chem. Soc. Jpn., 2007, 80, 1580; CrossRef c) A. Nakamura, S. Kawasaki, K. Toyota, and M. Yoshifuji, J. Organomet. Chem., 2007, 78, 692.
4. a) K. Toyota, J. Ujita, S. Kawasaki, K. Abe, N. Yamada, and M. Yoshifuji, Tetrahedron Lett., 2004, 45, 7609; CrossRef b) S. Kawasaki, J. Ujita, K. Toyota, and M. Yoshifuji, Chem. Lett., 2005, 34, 724; CrossRef c) N. Yamada, K. Toyota, and M. Yoshifuji, Chem. Lett., 2001, 248. CrossRef
5. a) A. S. Gajare, R. S. Jensen, K. Toyota, M. Yoshifuji, and F. Ozawa, Synlett, 2005, 144; b) K. Toyota, K. Masaki, T. Abe, and M. Yoshifuji, Chem. Lett., 1995, 221, See, also; CrossRef c) K. Toyota, K. Tashiro, and M. Yoshifuji, Chem. Lett., 1991, 2079. CrossRef
6. a) K. Toyota, Y. Goto, K. Okada, and N. Morita, Heterocycles, 2007, 71, 2227; CrossRef b) K. Toyota, K. Okada, H. Katsuta, and N. Morita, Tetrahedron, in press; c) K. Toyota, Y. Tsuji, K. Okada, and N. Morita, Heterocycles, 2009, in press. CrossRef
7. K. R. J. Thomas, J. T. Lin, and Y. S. Wen, Organometallics, 2000, 19, 1008. CrossRef