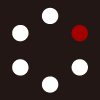
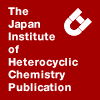
HETEROCYCLES
An International Journal for Reviews and Communications in Heterocyclic ChemistryWeb Edition ISSN: 1881-0942
Published online by The Japan Institute of Heterocyclic Chemistry
e-Journal
Full Text HTML
Received, 6th August, 2008, Accepted, 3rd October, 2008, Published online, 6th October, 2008.
DOI: 10.3987/COM-08-S(F)99
■ Synthesis and Properties of 2’-Deoxy-trans-3’,4’-BNA with S-Type Sugar Puckering
Tetsuya Kodama, Kensaku Sugaya, Yasuki Harada, Yasunori Mitsuoka, Takeshi Imanishi, and Satoshi Obika*
Graduate School of Pharmaceutical Science, Osaka University, 1-6 Yamadaoka, Suita, Osaka 560-0871, Japan
Abstract
2’-Deoxy-trans-3’,4’-BNA monomer, which was previous synthesized as part of a study on bridged nucleic acids with S-type sugar puckering, was introduced into an oligodeoxynucleotide using phosphoramidite chemistry, and the hybridization ability of the 2’-deoxy-trans-3’,4’-BNA-modified oligonucleotide with complementary DNA and RNA strands was evaluated. The 2’-deoxy-trans-3’,4’-BNA-modified oligonucleotide showed greatly improved hybridization ability compared to its 2’-methoxy congener (2’-methoxy-trans-3’,4’-BNA), which suggested that the sugar-restriction of nucleic acids to S-type conformation with appropriate design may be useful for development of DNA mimics.INTRODUCTION
Chemical modification of nucleic acid sugar moieties is an important approach in the development of oligonucleotides that interact strongly with complementary DNA and/or RNA. Numerous sugar-modified nucleic acid analogues have been synthesized and characterized. A series of 2’-O,4’-C-bridged nucleic acids (2’,4’-BNAs1–4/LNA5), in which the sugar moiety is tightly restricted to an N-type conformation, are attractive nucleic acid analogues because they confer high binding affinity towards complementary RNA strands and show superior resistance to enzymatic digestion, and they have been synthesized for use in various fields, including antisense strategy6 and microRNA silencing technology.7
Oligonucleotides in which sugar puckering is restricted to S-type are expected to act as DNA mimics and to form more stable duplexes than natural DNA, and are also fascinating materials due to their potential applications in such technologies as DNA chips and decoy strategy. Several nucleoside analogues with
S-type sugar puckering have been prepared, and the hybridizing abilities of their oligonucleotide derivatives have been evaluated.8-17 However, most oligonucleotides containing a fixed S-type sugar moiety show depressed hybridizing abilities, probably due to unfavorable restriction of the nucleobase (torsion angle around glycoside bond χ) and of the 5’-hydoxyl group (torsion angle γ), and/or steric repulsion between the additional structural components and neighboring nucleotide residues in B-type duplexes.
We recently synthesized several nucleic acid analogues with S-type sugar puckering, trans-3’,4’-BNA,8-10 which have an additional trans-fused ring between C3’ and C4’ (Figure 1). Crystallographic analysis and preliminary computer modeling studies showed that the conformation of the sugar moiety was fixed to S-type (pseudorotation phase angle18 P = 194°, C3’-exo) and that the additional bridged moiety was located outside the B-type duplex.10,19 Based on molecular dynamics studies, Venkateswarlu and Ferguson discussed the possibility that oligonucleotides containing sugar moieties that ware restricted to a large pseudorotation phase angle would favor B-type duplex formation rather than A-type.20 Preliminary conformational analysis and molecular modeling implied that trans-3’,4’-BNAs would form stable B-type duplexes with the complementary DNA strands. In previous work, 2’-methoxy-trans-3’,4’-BNA (2) bearing an arabino-type 2’-methoxy group on the sugar moiety was successfully incorporated into oligonucleotides, and their hybridizing abilityies were evaluated.9 However, the binding affinities of oligonucleotides containing 2 with complementary DNA and RNA strands were, contrary to our expectations, much lower than that of the natural oligonucleotides (–12 to –14 °C). Wengel and the co-workers reported that oligonucleotides containing 2’-OCH3-araT (3) showed slightly lower hybridizing ability than natural DNA (–4 to –6 °C),21 and also that the methoxy group did not have a significant influence on the duplex structure as it was positioned in the major groove.20,21 These results, together with our results concerning 2, indicated that the methoxy group of 2 caused steric repulsion between O5’ (or C6) and O2’ and/or an unfavorable χ angle in the B-type duplex, because the methoxy group and the 5’-methylene group occupied pseudo-axial positions due to the tight restriction of S-type sugar puckering. On this basis, it was suggested that the removal of the methoxy group from 2’-methoxy-trans-3’,4’-BNA (2) should reduce its conformational disadvantage for stable duplex formation, allowing the development of a simple S-type-restricted DNA analogue. In this report, we describe the synthesis of a 2’-deoxy-trans-3’,4’-BNA-modified oligonucleotide with no steric repulsion around the 2’-position, and discuss the hybridization ability and conformational properties of the corresponding oligonucleotide based on Tm values and circular dichroism (CD) spectroscopy.
RESULTS AND DISCUSSION
The 2’-deoxy-trans-3’,4’-BNA monomer 1 was synthesized from thymidine according to previously reported methods.10 To incorporate the monomer into oligonucleotides, 2’-deoxy-trans-3’,4’-BNA (1) was converted to the corresponding phosphoramidite 5 (Scheme 1). Treatment of 1 with 4,4’-dimethoxytrityl triflate22 afforded 5’-O-selective protection, and compound 4 was obtained in quantitative yield. Phosphitylation of 4 using 2-cyanoethyl N,N,N’,N’-tetraisopropylphosphorodiamidite and dicyanoimidazole at 50 °C afforded the corresponding phosphoramidite 5. Because the usual protocol for DNA synthesis did not give the desired oligonucleotide, oligonucleotide III containing 2’-deoxy-trans-3’,4’-BNA was synthesized as follows. First, a 5’-unprotected oligonucleotide 5’-TTTGCT-3’ (6) attached to CPG (controlled pore glass) at the 3’-hydroxy position was prepared on
column by an automated DNA synthesizer using conventional protocols. Incorporation of 5 into the oligonucleotide was achieved by mixing the CPG-supported oligonucleotide 6, phosphoramidite 5 and 5-ethylthio-1H-tetrazole using a vortex mixer at 50 °C for 24 hours. After replacement on the automated DNA synthesizer, the CPG-supported oligonucleotides was treated with acetic anhydride to cap the unreacted hydroxyl group of 6, and the phosphorous group was oxidized with tert-butyl hydroperoxide instead of iodine to avoid oligonucleotide strand break,23,24 giving 7. The upstream sequence was synthesized by conventional DNA synthesis protocols using 4,5-dicyanoimidazole as an activator and tert-butyl hydroperoxide as an oxidizer. The resulting DMTr-protected oligonucleotide was cleaved from the CPG solid support and protecting groups were removed by treatment with concentrated aqueous ammonia in the usual manner. The resulting crude oligonucleotide was roughly purified, the DMTr group was removed with aqueous trifluoroacetic acid on reverse phase column cartridge, and the product was further purified by HPLC. The sequence composition of 2’-deoxy-trans-3’,4’-BNA-modified oligonucleotide III was verified by MALDI-TOF mass spectroscopy.
The hybridization abilities of various oligonucleotides towards their complementary strands were evaluated by means of UV melting experiments. The melting temperatures (Tm) of the duplex oligonucleotides are shown in Table 1. As reported previously,9 2’-methoxy-trans-3’,4’-BNA modification of oligonucleotide II led to significant destabilization of its duplex DNA (–15 °C) and RNA (–13 °C) compared to those of the natural oligonucleotide I. In contrast, the 2’-deoxy-trans-3’,4’-BNA-modified oligonucleotide III formed duplexes with complementary RNA and DNA that were as stable as those of the natural oligonucleotide I, with Tm value of 49 °C (DNA) and 44 °C (RNA). Thus, the hybridization abilities of III were remarkably improved compared with II. These results confirm that the methoxy moiety of 2’-methoxy-trans-3’,4’-BNA (2) interferes with stable DNA/DNA and DNA/RNA duplex formation, although the mechanism of methoxy-group-induced destabilization is still unclear.
CD spectra of duplexes involving 2’-deoxy-trans-3’,4’-BNA were measured at 10 °C (Figure 2). As shown in Figure 2a, the CD profile of the 2’-deoxy-trans-3’,4’-BNA-modified oligonucleotide III/natural DNA IV-dna duplex implied a B-type helix structure, as evidenced by a positive Cotton effect around 280 nm and a negative Cotton effect around 250 nm; this is very similar to the spectrum of natural DNA. Furthermore, the III/natural RNA IV-rna duplex showed a structure that was intermediate between A-type and B-type; this was supported by a relatively strong Cotton effect around 270 nm in its CD spectrum, which, again, was almost the same as that of natural DNA/RNA (Figure 2b). These results provide evidence that 2’-deoxy-trans-3’,4’-BNA mimics the structure of DNA not only in DNA/DNA duplex but also in the DNA/RNA duplex with no significant change in the helical structure.
Despite the finding that the bridge moiety between 3’- and 4’-position of trans-3’,4’-BNA did not affect the helix structure of DNA/DNA and DNA/RNA duplexes, the conformational restriction of the sugar moiety seems not to have worked effectively in term of thermal stabilization. It is likely that the entropic advantage of conformational fixing during duplex formation was canceled out by unfavorable interactions, e.g. disturbance of hydration network due to the additional hydrophobic bridge structure. It is notable that the 2’-deoxy-trans-3’,4’-BNA-modified oligonucleotide, which is comparable to tricyclo-DNA14, showed high binding ability with complementary DNA among artificial nucleic acids with S-type sugar puckering. This implies the possibility of creating superior artificial S-type nucleic acids by fixing the sugar conformation to S-type but employing a more appropriate bridge structure.
CONCLUSION
We successfully synthesized 2’-deoxy-trans-3’,4’-BNA with S-type sugar puckering. The binding affinity of 2’-deoxy-trans-3’,4’-BNA-modified oligonucleotide towards complementary DNA was significantly improved compared with that of the 2’-methoxy-trans-3’,4’-BNA-modified oligonucleotide, indicating that the removal of the 2’-methoxy group from 2’-methoxy-trans-3’,4’-BNA was advantageous. All of the analytical data supported 2’-deoxy-trans-3’,4’-BNA (1) was an effective mimic of the DNA component. Furthermore, this work revealed the possibility of creating artificial S-type nucleic acids by fixing the sugar conformation to S-type but employing a more appropriate bridge structure. The sugar-restriction of nucleic acids to an S-type conformation should prove useful for designing DNA mimics.
EXPERIMENTAL
Unless otherwise mentioned, all chemicals from commercial sources were used without further purification. Acetonitrile (MeCN), dichloromethane (CH2Cl2), pyridine and tetrahydrofuran (THF) used in reactions were distilled from calcium hydride just before use. All reactions were performed under an atmosphere of nitrogen. Melting points were measured on a Yanagimoto micro melting points apparatus and are uncorrected. 1H, 13C, and 31P NMR spectra were recorded on JEOL EX-270 (1H, 270 MHz; 13C, 67.8 MHz), GX-500 (1H, 500 MHz; 31P 202 MHz) instruments. Values for δ are in ppm relative to tetramethylsilane or deuterated solvent as internal standard. 31P NMR spectra were recorded with 85% H3PO4 as an external standard. IR spectra were recorded on a JASCO FT/IR-200 spectrometer. FAB-Mass spectra were measured on a JEOL JMS-600 or JMS-700 mass spectrometer. Column chromatography was carried out using Fuji Silysia BW-127ZH, BW-300 and FL-100D silica gel. DNA synthesis was performed using an Applied Biosystems ExpediteTM 8909 instrument. MALDI-TOF-MS spectra were recorded in negative ion mode on Brucker Daltonics Autoflex II TOF/TOF instrument. UV melting experiments were carried out using a Shimadz UV-1650PC instrument.
5’-O-(4,4’-Dimethoxytrityl)-3’-C,4’-C-(3-oxabutylene)thymidine (4). To a stirring mixture of AgOTf (480 mg, 1.87 mmol) in CH2Cl2 (2.0 mL) was added a solution of 4,4’-dimethoxytrityl chloride (633 mg, 1.87 mmol) in CH2Cl2 (3.0 mL) at rt. Part of the resulting solution layer (1.00 mL, 0.374 mmol) was added to a solution of compound 110 in pyridine-CH2Cl2 (1:1, 7.4 mL) at rt, and the mixture was stirred at the same temperature for 5 h. After addition of saturated aqueous NaHCO3 to the reaction mixture, the contents were extracted with AcOEt. The organic layer was washed with H2O and brine, dried over anhydrous Na2SO4, and evaporated. The resulting crude product was purified by silica gel column chromatography (CHCl3:MeOH = 30:1) to afford 4 (99 mg, 84%) as a colorless amorphous solid. [α]D23 −63.0 (c 1.00, CHCl3). IR νmax (KBr): 1034, 1059, 1102, 1177, 1252, 1275, 1325, 1466, 1509, 1608, 1661, 1688, 2836, 2954, 3461 cm−1. 1H-NMR (CDCl3) δ 1.46 (3H, d, J = ca. 0 Hz), 1.55 (1H, dd, J = 6, 12 Hz), 2.01 (1H, dd, J = 8, 13 Hz), 2.81-2.88 (2H, m), 3.61 (1H, dd, J = 5, 11 Hz), 3.78 (6H, s), 3.85-3.90 (1H, m), 4.09 (2H, dd, J = 10, 13 Hz), 5.94 (1H, dd, J = 5, 7 Hz), 6.79 (4H, dd, J = 3, 9 Hz), 7.18-7.40 (9H, m), 7.93 (1H, s), 9.41 (1H, brs). 13C-NMR (CDCl3) δC 12.1, 33.0, 44.3, 55.2, 61.5, 64.3, 64.8, 76.3, 84.9, 87.0, 88.1, 109.7, 112.9, 113.0, 127.1, 127.8, 128.2, 130.1, 130.2, 135.3, 135.5, 137.0, 144.4, 150.5, 158.6, 158.6, 164.1. MS (FAB): m/z 601 (MH+). High-resolution MS (FAB): Calcd for C34H36N2O8 (MH+): 601.2532. Found: 601.2550.
1-[3’-O-(N,N-Diisopropylamino-β-cyanoethoxyphosphino)-5’-O-(4,4’-dimethoxytrityl)-3’-C,4’-C-(3-oxabutylene)thymidine (5). To a mixed solution of compound 4 (97 mg, 0.16 mmol) in MeCN and THF (3:1, v/v, 3.2 ml) was added 2-cyanoethyl N,N,N’,N’-tetraisopropylphosphorodiamidite (0.16 mL, 0.49 mmol) and 4,5-dicyanoimidazole (1.6 mg, 0.40 mmol) at rt. After stirring at 40 °C for 46 h, saturated aqueous NaHCO3 was added to the mixture, which was then extracted with AcOEt. The organic layer was washed with H2O and brine, dried over anhydrous Na2SO4, and evaporated. The resulting crude mixture was purified by silica gel column chromatography (n-hexane:EtOAc = 3:2) followed by triturated from n-hexane to afford 5 (54 mg, 42%) as a white solid. mp 111–112°C. 31P-NMR (CDCl3) δP 140.1, 141.7. MS (FAB): m/z 801 (MH+). High-resolution MS (FAB): Calcd. For C43H54N4O9P (MH+): 801.3628. Found: 801.3632.
Synthesis of oligodeoxynucleotides. Synthesis of oligodeoxynucleotides III was performed on a 0.2 μmol scale using commercially available 2-cyanoethyl phosphoramidite, 4,5-dicyanoimidazole as an activator, and tert-butyl hydroperoxide (1 M in toluene) as the oxidizer for natural bases with automated DNA synthesizer. First, 5’-unprotected oligodeoxynucleotide 6 was prepared on CPG solid support in a column using conventional protocols for DNA synthesis, as described above. To introduce 2’-deoxy-trans-3’,4’-BNA into oligonucleotide 6, a solution of 5 (8.3 mg/50 μL in MeCN) was combined by hand with a solution of 5-ethylthio-1H-tetrazole (0.25 M in MeCN, 50 μL) in the column containing CPG-solid-supported 6, and vibrated at 50 °C for 24 h with a vortex mixer. After replacement on the automated DNA synthesizer, the solid-supported oligodeoxynucleotides in the reaction column was washed with MeCN and treated with Ac2O, 1-methylimidazole in a mixed solution of THF and pyridine followed by 1 M tert-butyl hydroperoxide in toluene to afford 7. Continuous solid synthesis was accomplished by the above-described conventional protocols. The 5’-O-DMTr-protected oligonucleotides were cleaved from the solid support by treatment with conc. ammonia at rt for 1.5 h, and the protecting groups were removed at 55 °C for 12 h. The obtained 5’-O-DMTr-protected oligonucleotide was detritylated and purified using disposable reverse phase column cartridges (Sep-Pak® Plus C18 Environmental Cartridges, Waters) [washed with 10% MeCN aqueous solution, detritylated with 0.5% TFA aqueous solution, and eluted with 35% MeOH aqueous solution]. The obtained oligonucleotides were again purified by HPLC (Waters XTerra® MS C18 2.5 mm, 50 °C, detected at 254 nm) with linear gradient of MeCN (6% to 11.3%) in 0.1 M TEAA (pH 7) over 20 min, afforded the pure oligonucleotide III. MALDI-TOF mass of oligonucleotides (20 to 50 pmol) using a 1:1 mixture of 3-hydroxypicolinic acid and diammonium hydrogen citrate as the matrix gave the following results: Calcd. 3686.84 (MH–). Found 3686.51.
UV melting experiments. UV melting experiments were carried out in a medium salt buffer containing 10 mM sodium phosphate (pH 7.2), 100 mM NaCl using each 2 μM concentrations of the two complementary sequences. The increase in absorbance at 260 nm as a function of time was recorded while the temperature was raised linearly from 5 to 90 °C at a rate of 0.5 °C min–1. The melting temperature was determined as the local maximum of the first derivatives of the absorbance vs. temperature curve. Melting temperatures (Tm values) given here are the averages of three independent experiments.
CD measurements. CD spectra were recorded at 10 °C in a buffer containing 10 mM sodium phosphate (pH 7.2), 100 mM NaCl using each 4 μM of the two complementary sequences in the quartz cuvette of 1 cm optical path length. The molar ellipticity was calculated from the equation [θ] = θc-1l-1, where θ is the relative intensity, c is the sample concentration and l is the cell path length in centimeters.
ACKNOWLEDGEMENTS
Part of this work was supported by Grant-in-Aid for Science Research (KAKENHI) from the Japan Society for the Promotion of Science (JSPS).
This paper is dedicated to Professor Emeritus, Tohoku University, Keiichiro Fukumoto on the occasion of his 75th birthday.
References
1. T. Imanishi and S. Obika, Chem. Commun., 2002, 1653. CrossRef
2. S. Obika, D. Nanbu, Y. Hari, K. Morio, Y. In, T. Ishida, and T. Imanishi, Tetrahedron Lett., 1997, 38, 8735; CrossRef S. Obika, D. Nanbu, Y. Hari, J. Andoh, K. Morio, T. Doi, and T. Imanishi, Tetrahedron Lett., 1998, 39, 5401. CrossRef
3. Y. Hari, S. Obika, R. Ohnishi, K. Eguchi, T. Osaki, H. Ohishi, and T. Imanishi, Bioorg. Med. Chem., 2006, 14, 1029. CrossRef
4. K. Miyashita, S. M. A. Rahman, S. Seki, S. Obika, and T. Imanishi, Chem. Commun., 2007, 3765; CrossRef S. M. A. Rahman, S. Seki, K. Utsuki, S. Obika, K. Miyashita, and T. Imanishi, Nucleosides Nucleotides Nucleic Acids, 2007, 26, 1625; CrossRef S. M. A. Rahman, S. Seki, S. Obika, S. Haitani, K. Miyashita, and T. Imanishi, Angew. Chem. Int. Ed., 2007, 46, 4306; CrossRef S. M. A. Rahman, S. Seki, S. Obika, H. Yoshikawa, K. Miyashita, and T. Imanishi, J. Am. Chem. Soc., 2008, 130, 4886. CrossRef
5. J. Wengel, Acc. Chem. Res., 1999, 32, 301; CrossRef A. A. Koshkin, P. Nielsen, M. Meldgaard, V. K. Rajwanshi, S. K. Sing, and J. Wengel, J. Am. Chem. Soc., 1998, 120, 13252; CrossRef V. K. Rajwanshi, A. E. Hakansson, M. D. Sørensen, S. Pitsch, S. K. Sing, R, Kumar, P. Nielsen, and J. Wengel, Angew. Chem. Int. Ed., 2000, 39, 1656. CrossRef
6. M. R. Jakobsen, J. Haasnoot, J. Wengel, B. Berkhout, and Jørgen Kjems, Retrovirology, 2007, 4, 29. CrossRef
7. J. Elme’n, M. Lindow, S. Schütz, M. Lawrence, A. Petri, S. Obad, M. Lindholm, M. Hedtjärn, H. F. Hansen, U. Berger, S. Gullans, P. Kearney, P. Sarnow, E. M. Straarup, and S. Kauppinen, Nature, 2008, 452, 896. CrossRef
8. S. Obika, M. Sekiguchi, T. Osaki, N. Shibata, M. Masaki, Y. Hari, and T. Imanishi, Tetrahedron Lett., 2002, 43, 4365; CrossRef S. Obika, T. Osaki, M. Sekiguch, R. Somjing, Y. Harada, and T. Imanishi, Tetrahedron Lett., 2004, 45, 4801. CrossRef
9. M. Sekiguch, S. Obika, Y. Harada, T. Osaki, R. Somjing, Y. Mitsuoka, N. Shibata, M. Masaki, and T. Imanishi, J. Org. Chem., 2006, 71, 1306. CrossRef
10. K. Sugaya, Y. Harada, Y. Mitsuoka, T. Osaki, S. Obika, T. Kodama, and T. Imanishi, Nucleic Acids Symp. Ser., 2007, 51, 155; CrossRef T. Osaki, S. Obika, Y. Harada, Y. Mitsuoka, K. Sugaya, M. Sekiguchi, S. Roongjang, and T. Imanishi, Tetrahedron, 2007, 63, 8977. CrossRef
11. S. Obika, M. Onoda, K. Morita, J. Andoh, M. Koizumi, and T. Imanishi, Chem. Commun., 2001, 1992; CrossRef S. Obika, O. Nakagawa, A. Hiroto, Y. Hari, and T. Imanishi, Chem. Commun., 2003, 2202; CrossRef S. Obika, M. Sekiguchi, R. Somjing, and T. Imanishi, Angew. Chem. Int. Ed., 2005, 44, 1944. CrossRef
12. T. Kodama, C. Matsuo, H. Ori, S. Obika, K. Miyashita, and T. Imanishi, Nucleic Acids Symp. Ser., 2007, 51, 159. CrossRef
13. M. Tarköy, M. Bolli, B. Schweizer, and C. Leumann, Helv. Chim. Acta, 1993, 76, 481; CrossRef M. Bolli, H. U. Trafelet, and C. Leumann, Nucleic Acids Res. 1996, 24, 4660; CrossRef M. Bolli and C. Leumann, Angew. Chem., Int. Ed. Engl., 1995, 34, 694; CrossRef M. Bolli, J. C. Litten, R. Schütz, and C. J. Leumann, Chem. Biol., 1996, 3, 197. CrossRef
14. R. Steffens and C. Leumann, Helv. Chim Acta, 1997, 80, 2426; CrossRef R. Steffens and C. J. Leumann, J. Am. Chem. Soc., 1997, 119, 11548; CrossRef R. Steffens and C. J. Leumann, J. Am. Chem. Soc., 1999, 121, 3249; CrossRef D. Renneberg and C. J. Leumann, J. Am. Chem. Soc., 2002, 124, 5993. CrossRef
15. K.-H. Altmann, R. Imwinkelried, R. Kesselring, and G. Rihs, Tetrahedron Lett., 1994, 35, 7625; CrossRef M. A. Maier, Y. Choi, H. Gaus, J. J. Jr. Barchi, V. E. Marquez, and M. Manoharan, Nucleic Acids Res., 2004, 32, 3642. CrossRef
16. P. Nielsen, H. M. Pfundheller, C. E. Olsen, and J. Wengel, J. Chem. Soc., Perkin Trans. 1, 1997, 3423. CrossRef
17. J. Ravn, N. Thorup, and P. Nielsen, J. Chem. Soc., Perkin Trans. 1, 2001, 1855. CrossRef
18. C. Altona and M. Sundaralingam, J. Am. Chem. Soc., 1972, 94, 8250. CrossRef
19. W. Saenger, Principles of Nucleic Acid Structure, Springer, New York, 1984.
20. D. Venkateswarlu and D. M. Ferguson, J. Am. Chem. Soc., 1999, 121, 5609. CrossRef
21. C. H. Gotfredsen, J. P. Jacobsen, and J. Wengel, Bioorg. Med. Chem. Lett., 1996, 4, 1217; CrossRef C. H. Gotfredsen, H. P. Spielmann, J. Wengel, and J. P. Jacobsen, Bioconjugate Chem., 1996, 7, 680. CrossRef
22. M. Tarköy, M.Bolli, and C. Leumann, Helv. Chim. Acta, 1994, 77, 716. CrossRef
23. C. Scheuer-Larsen, B. M. Dahl, J. Wengel, and O. Dahl, Tetrahedron Lett., 1998, 39, 8361. CrossRef
24. Y. Hayakawa, M. Uchiyama, and R. Noyori, Tetrahedron Lett., 1986, 27, 4191 CrossRef