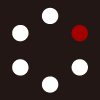
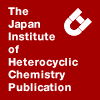
HETEROCYCLES
An International Journal for Reviews and Communications in Heterocyclic ChemistryWeb Edition ISSN: 1881-0942
Published online by The Japan Institute of Heterocyclic Chemistry
e-Journal
Full Text HTML
Received, 7th August, 2008, Accepted, 27th November, 2008, Published online, 1st December, 2008.
DOI: 10.3987/REV-08-SR(F)6
■ The Lycopodium Alkaloids
Yusuke Hirasawa, Jun'ichi Kobayashi, and Hiroshi Morita*
Faculty of Pharmaceutical Sciences, Hoshi University, 2-4-41 Ebara, Shinagawa-ku, Tokyo 142-8501, Japan
Abstract
Lycopodium alkaloids are unique heterocyclic alkaloids having C11N, C15N2, C16N, C16N2, C22N2, and C27N3 types from genus Lycopodium and have attracted great interest from biogenetic and biological points of view as well as providing challenging targets for total synthesis. This review covered the structure elucidation and biological activity of new Lycopodium alkaloids and total synthesis of some Lycopodium alkaloids reported in the literature from 2004 to July in 2008.INTRODUCTION
Lycopodium species produce a number of structurally diverse alkaloids, which often possess unusual skeletons, and many of them continue to be of interest from the biogenetic and biological points of view, as well as providing challenging targets for total synthesis. There are over 500 species in the genus Lycopodium (family Lycopodiaceae), but the alkaloid content has been studied about 50 species.1-7 Most of the species are low, evergreen, coarsely moss-like plants, which are commonly known as club mosses. They are non-flowering plants which reproduce by means of spores rather than seeds. In many species, the spore-bearing bodies, known as strobili, appear as club-shaped growths at the tips of the moss like branches, hence the name club mosses. The taxonomy of the genus and the family is still in a state of flux. Some botanists have subdivided the genus into four genera (Lycopodium, Diphasiastrum, Lycopodiella, and Huperzia), and some have placed Huperzia in a separate family.8,9 We prefer to retain the single genus name, Lycopodium that can be easily recognized as being closely related.
In 1986, Liu and co-workers isolated two new Lycopodium alkaloids, huperzines A (1) and B from Huperzia serrata (Lycopodium serratum), which is a Chinese traditional medicine.10 Huperzine A (1) has been shown to be a potent, reversible inhibitor of acetylcholinesterase and shows promise in the treatment of Alzheimer’s disease and myasthenia gravis.10-13 The inherent inhibition of acetylcholinesterase has promoted the pursuit of the total synthesis14-19 and SAR11,12 studies of huperzine A. Since the inhibition of acetylcholinesterase activity of huperzine A was detected, clinical trials with Lycopodium alkaloids have been carried out. Many reports on clinical trials of huperzine A and its analogue have been published in recent years.
The biosynthesis of the alkaloids is still not completely understood, and only limited biosynthetic studies have been reported.20-33 Plants of the genus Lycopodium have not been cultivated, and labeling experiments must be carried out in the field. Because the club mosses often are not easily accessible, very few feeding studies have been conducted.34-36 This is an area where plant tissue culture may prove extremely useful in future biosynthetic studies.
Ther are some reviews of the chemistry of Lycopodium alkaloids,1-7 and of the biology and chemistry of huperzine A (1).10-13 Since the last review by us in Volume 617 of The Alkaloids, a number of new Lycopodium alkaloids have been discovered. As a result, the number of known Lycopodium alkaloids has grown markedly in recent years to a present count of ca. 250. These alkaloids, isolated chiefly by Manske and Ayer et al., are classified into different frameworks of C16N, C16N2, and C27N3 types.1-4 These unusual ring systems have attracted great interest as challenging targets for total synthesis or biosynthetic studies.
This review covers the reports on Lycopodium alkaloids that have been published between 2004 to July in 2008, and provides an update of the previous our review7 in 2005. The natural Lycopodium alkaloids published between 2004 to July 2008 (compounds 2-51) are listed in Table 1.
This review describes the recent studies on Lycopodium alkaloids isolated from the genus Lycopodium and Huperzia, and the syntheses of Lycopodium alkaloids.
A. LYCOPODINE RELATED ALKALOIDS
Six new lycopodine related alkaloids were isolated from some Lycopodium species. These alkaloids possess fused tetra-6-membered rings system, so-called lycopodane skeleton.
12-Epilycodoline-N-oxide (2), 7-hydroxylycopodine (3), and 4,6α-dihydroxylycopodine (4) were isolated from the basic material of the whole plant of Huperzia serrata (Thunb.).37 Their structures were elucidated spectroscopically, especially by means of 1D and 2D NMR.
A new alkaloid, sauroine (5) was isolated from the aerial parts of Lycopodium saururus.38 The relative stereochemistry of sauroine was 7α,8-endo-dihydroxylycopodine. Sauroine did not inhibit acetylcholinesterase with 10 µg/mL.
With an aim to isolate structurally interesting alkaloids and key intermediates to clarify the biogenetic pathway, purification of extracts of L. obscurum led to isolate two new alkaloids, obscurumines A (6) and B (7).39 The structure and stereochemistry of 6 and 7 were assigned as N-oxide form of lycodoline58 and 8-dehydro form of fawcettiine59 respectively from combination of 2D NMR spectra. The absolute configuration of obscurumine A (6) was assigned by chemical transformation of lycodoline with m-CPBA.
B. LYCOPODATINES
Further investigation on extracts of L. inundatum resulted in the isolation of three new C16N type alkaloids, lycopodatines A-C (8-10),40 as well as known related alkaloids, inundatine,60,61 debenzoylalopecurine, 60,61 and anhydrolycodoline.62
Lycopodatine A (8) had a molecular formula of C17H28NO2 by HRESIMS [m/z 278.2117, (M)+, Δ −0.3 mmu]. The IR spectrum was indicative of the presence of a hydroxy group (3440 cm−1). 1H and 13C NMR data of 8 were analogous to those of debenzoylalopecurine, although the three carbons C-1 (δC 67.7; δH 3.25, 3.82), C-9 (δC 72.1; δH 3.04, 4.66), and C-13 (δC 80.7) were remarkably shifted to lower field as compared to those of debenzoylalopecurine. Furthermore, a methyl signal (δC 3.00; δH 45.4) was observed in the 1H and 13C NMR spectra of 8. The molecular structure of 8 was deduced from extensive analyses of the two-dimensional NMR data, including the 1H−1H COSY, HOHAHA, HMQC, and HMBC spectra in CD3OD (Figure 1). The 1H-1H COSY and HOHAHA spectra revealed connectivities of two partial structures a (C-1−C3) and b (C-5−C-8, C-9−C-12, and C-14−C-16), as shown in Figure 1. Connectivities of C-17 to C-1, C-9, and C-13 through a nitrogen atom were implied by HMBC correlations for H3-17 to C-1, C-9, and C-13. HMBC correlations were observed for H-2, H-5, and H-9a to C-4 (δC 54.6), suggesting that C-3, C-5, and C-10 were connected through C-4. HMBC cross-peaks for H-14 to C-4, C-12, and C-13 indicated that C-4, C-12, and C-14 were connected through C-13. Thus, the molecular structure of lycopodatine A was elucidated to be 8, possessing an alopecurane skeleton with two hydroxy groups at C-2 and C-5 and an N-methyl group. The relative configuration of 8 was elucidated by NOESY correlations and 3JH−H couplings as depicted in the computer-generated three-dimensional drawing (Figure 1). The chair conformation of the cyclohexane ring (C-7−C-8, C-12−C-15) was deduced from NOESY correlations, as shown in Figure 1. The methyl group at C-15 was assigned to be equatorial from the large 3J coupling (12.4 Hz) between H-14a and H-15. The NOESY correlation of H-2/H-14b and H-5/H-10 indicated that the hydroxy groups at C-2 and C-5 were α- and β-orientated, respectively.
Treatment of debenzoylalopecurine with methyl iodide afforded an N-methyl derivative, whose spectroscopic data and specific rotation were identical with lycopodatine A (8). Thus, the absolute configuration of lycopodatine A was assigned as 8.
Lycopodatine B (9) had a molecular formula of C17H26NO by HRESIMS [m/z 260.2037, (M)+, Δ +2.3 mmu]. The IR spectrum indicated the presence of a carbonyl group (1770 cm−1). 1H and 13C NMR data of 9 were similar to those of anhydrolycodoline, although the three carbons C-1 (δC 61.8; δH 3.40, 3.79), C-9 (δC 54.9; δH 3.23, 4.29), and C-13 (δC 72.5) were remarkably shifted to lower field. Furthermore, a methyl signal (δH 3.16; δC 48.9) was observed in the 1H and 13C NMR of 9. Two-dimensional NMR data, including the 1H−1H COSY, HOHAHA, HMQC, HMBC, and NOESY spectra in CD3OD (Figure 2), supported the structure of 9. Thus, the structure of lycopodatine B was assigned as the N-methylammonium form of anhydrolycodoline.
Lycopodatine C (10) had a molecular formula of C16H23NO by HRESIMS [m/z 246.1855 (M + H)+, Δ −0.3 mmu]. IR absorptions implied the presence of a carbonyl (1700 cm−1) group. 1H−1H COSY, HOHAHA, HMQC, HMBC and NOESY spectra suggested that 3 was the 4-epi form of anhydrolycodoline (Figure 3).
C. FAWCETTIMINE RELATED ALKLAOIDS
Five new Lycopodium alkaloids, 11α-hydroxyfawcettidine (11), 2α,11α-dihydroxyfawcettidine (12), 8α,11α-dihydroxyfawcettidine (13), 2β-hydroxylycothunine (14), and 8α-hydroxylycothunine (15) having the fawcettimine skeleton were isolated from L. serratum by Takayama and co-workers.41 The structures including absolute configuration of the alkaloids were elucidated on the basis of spectroscopic analysis.
D. LYCOVATINE A
A new C16N-type quaternary alkaloid, lycovatine A, was isolated from the club moss Lycopodium clavatum var. robustum.42 The relative stereochemistry of 16 was deduced from detailed analyses of 2D NMR data of 16 (Figure 4). The absolute configuration of C-5 in lycovatine A was deduced to be R configuration by applying the modified Mosher’s method.63
Effects of lycovatine A on neurotrophic factor biosynthesis in 1321N1 human astrocytoma cell were examined by a semiquantitative RT-PCR method39,64 to find that the mRNA expressions for NGF were enhanced by 16. Lycovatine A did not show cytotoxicity against murine leukemia L1210 cells and human epidermoid carcinoma KB cells (IC50 > 10 µg/mL). Lycovatine A exhibited antimicrobial activity against Cryptococcus neoformans (MIC, 0.52 µg/mL) and Aspergillus niger (MIC, 2.05 µg/mL).
E. LANNOTINIDINES
Seven new Lycopodium alkaloids, lannotinidines A−G (17-23), have been isolated from the club moss Lycopodium annotinum and L. annotinum var. acrifolium.43 Lannotinidine A (17) is structural isomer of annopodine.65,66 Lannotinidine B (18) has similar stereostructure with lyconesidine A.67 Lannotinidine C (19) and D (20) are new alkaloids consisting of deacetylfawcettiine59,68 or lycofoline59,69 with ferulic acid ester at C-5, respectively. Treatment of annotinine70,71 with sulfuric acid gave a dihydroxy derivative, whose spectral data and [α]D value were identical with those of lannotinidine E (21). Lannotinidine F {22, [α]D24 −22° (c 1.0, MeOH)} was revealed to have the molecular formula, C16H19NO3, by HRFABMS [m/z 274.1432 (M+H)+, Δ −1.1 mmu]. IR absorptions implied the presence of carbonyl (1770 and 1680 cm−1) groups. 1H and 13C NMR data suggested the presence of two ketones, two sp2 methines, five sp3 methylenes, four sp3 methines, one methyl, and two sp3 quaternary carbon. Among them, one sp2 methine (δC 153.6), one methylene (δC 49.9), and one quaternary carbon (δC 66.6) were ascribed to those bearing a nitrogen. The 1H−1H COSY and HOHAHA spectra of 22 revealed four structural units as shown in Figure 5. The presence of 2,3-dihydro-4-pyridinone and γ-lactone moieties was suggested by HMBC correlations for H-9 of C-11 and C-13, H-5 and H-6 of C-8, respectively. Connectivities among C-1, C-9, and C-13 through a nitrogen were elucidated by HMBC cross-peaks for H-1 of C-9 and C-13. HMBC correlations for H-1, H-5, and H-14 of C-13, H-7, H-14, and H-16 of C-12, H-7 and H-16 of C-11, and H-4 of C-14 gave rise to connectivities among the four units through a nitrogen atom, C-11, C-12, and C-13. Thus, the gross structure of lannotinidine F was elucidated to be 22.
The relative stereochemistry of 22 was deduced from cross-peaks observed in the phase sensitive NOESY spectrum as shown in computer-generated 3D drawing (Figure 5).
Lannotinidine G (23) was revealed to have the molecular formula, C16H21NO2, by HRFABMS [m/z 260.1653 (M+H)+, Δ +0.3 mmu]. IR absorptions implied the presence of carbonyl (1685 cm−1) group. 1H and 13C NMR data suggested the presence of one ketone, three sp2 methines, one sp2 quaternary carbon, six sp3 methylenes, three sp3 methines, one methyl, and one sp3 quaternary carbon. Among them, signals due to three nitrogen-bearing carbons at δC 49.6, 49.8, and 62.0, and an oxygen-bearing carbon at δC 75.2 appeared.
The structure of 23 was elucidated by 2D NMR (1H–1H COSY, HOHAHA, HMQC, and HMBC) data (Figure 10). The 1H−1H COSY and HOHAHA spectra revealed connectivities of C-1−C-7, C-9−C-11, and C-14−C-16. These three partial units were connected to one another on the basis of HMBC correlations as shown in Figure 6. Lannotinidine G (23) was identical with a compound produced by hydrolysis of lyconnotine with KOH/MeOH followed by acidification. The relative stereochemistry of 23 was deduced from NOESY correlations (Figure 6).
F. LYCOPLADINES
Five new Lycopodium alkaloids lycopladine A−E (24-28) were isolated from the club moss Lycopodium complanatum,43 together with lyconadin A.72
Lycopladine A (24) showed the pseudomolecular ion peak at m/z 260 (M+H)+ in the ESIMS, and the molecular formula, C16H21NO2, was established by HRESIMS [m/z 260.1653, (M+H)+, Δ +0.2 mmu]. IR absorptions implied the presence of hydroxyl group (3380 cm−1) and ketone carbonyl (1700 cm−1). 13C NMR data of 24 revealed 16 carbon signals due to one carbonyl carbon, two sp2 quaternary carbons, three sp2 methines, one sp3 quaternary carbon, two sp3 methines, six sp3 methylenes, and one methyl group. Among them, two olefinic carbons [δC 148.8 (d), 164.3 (s)] assignable to nitrogen-bearing carbons were elucidated to form a disubstituted pyridine ring together with the remaining three olefinic carbons [δC 123.0 (d), 136.1 (d), 140.0 (s)]. The UV absorption [270 nm (ε 2800)] also supported the presence of the pyridine ring. Since five out of seven unsaturations were accounted for, 24 was inferred to possess two more rings.
The gross structure of 24 was elucidated by analyses of 2D NMR data including 1H−1H COSY, HOHAHA, HMQC, and HMBC spectra in CD3OD. The 1H–1H COSY and HOHAHA spectra of 24 revealed three structural units a (C-1–C-3), b (C-6−C-8, C-8−C-15, and C-14−C-16), and c (C-9−C-11) (Figure 7). It was elucidated that unit a constituted a 2,3-disubstituted pyridine ring by HMBC correlations of H-1 and H-3 (δH 8.30, 7.67, respectively) to C-5, and H-2 (δH 7.24) to C-4. HMBC correlations of H-11a (δH 2.06) to C-4, C-7, and C-12 revealed the connectivities from C-11 to C-4 and C-7 through C-12. The HMBC correlations of H2-11 and H2-14 (δH 2.29) to C-13 indicated the connectivities from C-11 to C-14 through C-12 and C-13. HMBC cross-peaks of H2-6 (δH 3.09, 2.83) to C-5 suggested the connectivity from C-6 to C-5. The remaining C-9 (δH 3.53, δC 62.8) was elucidated to be connected with a hydroxyl group. Thus, the gross structure of lycopladine A was assigned as 24.
The NOESY spectrum of 24 showed cross-peaks as shown in computer-generated 3D drawing. The relative configurations of C-7, C-12, and C-15 in the cyclohexanone ring (C-7, C-8, and C-12 to C-15) were deduced from NOESY correlations of H-3/H2-11, H-6b/H-15, H-7/H2-11, H-8b/H2-11, and H2-14/H2-11. Thus, the relative configurations of lycopladine A (24) were elucidated as shown in Figure 7.
Lycopladine A (24) showed weak cytotoxicity against murine lymphoma L1210 cells (IC50, 7 µg/mL) in vitro, while 24 did not show such activity against human epidermoid carcinoma KB cells (IC50 > 10 µg/mL).
Lycopladine B (25) showed the pseudomolecular ion peak at m/z 290 (M+H)+ in the ESI-MS, and the molecular formula, C17H23NO3, was established by HRESIMS [m/z 290.1759, (M+H)+, Δ +0.3 mmu]. IR absorptions implied the presence of hydroxy (3380 cm−1) and ester carbonyl (1710 cm−1) functionalities. 13C NMR data of 25 revealed 17 carbon signals due to one carbonyl carbon, two sp2 quaternary carbons, two sp2 methines, one sp3 quaternary carbon, three sp3 methines, seven sp3 methylenes, and one O-methyl group.
The gross structure of 25 was elucidated by analyses of 2D NMR data including 1H–1H COSY, HOHAHA, HMQC, and HMBC spectra in CD3OD (Figure 8). 1H–1H COSY and HOHAHA spectra of 25 revealed two structural units a (C-1 to C-8) and b (C-9 to C-11). In unit a, C-5 was deduced to be connected to a hydroxy group from the 1H and 13C NMR chemical shifts (δH 3.59 and δC 80.8). HMBC correlations of H2-6 (δH 2.08 and 1.96) and H-8 (δH 6.51) to C-12 (δC 48.2), and H-7 (δH 2.76) to C-4 (δC 59.9) suggested a connection of C-7 to C-4 through C-12, indicating the presence of a cyclopentane ring (C-4 to C-7 and C-12). HMBC cross-peaks of H-1b (δH 3.09) to C-9 (δC 53.5) and H-1a to C-13 revealed connections of C-1 to C-9, C-1 to C-13, and C-9 to C-13 through a nitrogen atom. While HMBC cross-peaks of H-10b (δH 1.61) and H-14 (δH 6.22) to C-12 (δC 48.2) and H-11a (δH 2.02) to C-13 (δC 147.9) indicated a connection of C-11 to C-14 through C-12 and C-13. HMBC cross-peaks of H-8 (δH 6.51) to C-14 and C-16 (δC 114.9 and 167.1, respectively) and H-7 (δH 2.76) to C-15 (δC 127.6) suggested that C-8 was connected to C-14 and C-16 through C-15. The presence of a methyl ester group was elucidated by the HMBC correlation of H-17 (δH 3.74) to C-16 (δC 167.1). Thus, the gross structure of lycopladine B was elucidated to be 25.
The NOESY spectrum of 25 showed cross-peaks as shown in computer-generated 3D drawing (Figure 8). The relative stereochemistry of H-5 was deduced to be a β-configuration from NOESY correlations of H-1b/H-3b, H-1b/H-14, and H-3b/H-5. While the relative stereochemistry of H-4 was deduced to be an α-configuration from the NOESY correlation of H-4/H-11a. A chair-like conformation of the piperidine ring (N-1 and C-9 to C-13) and an α-configuration of H-7 were suggested by NOESY correlations of H-7/H2-11.
The absolute configuration of C-5 in lycopladine B (25) was elucidated by the modified Mosher’s method for the MTPA esters of 25. The values of Δδ[δ(S-MTPA ester)−δ(R-MTPA ester)] for H2-6, H-7, and H-8 were negative, while the values of Δδ for H2-1, H2-2, H2-3, H-4, H2-9, H2-10, and H2-11 were positive. These data suggested that the absolute configuration at C-5 was S. Thus, the absolute stereochemistry of 25 was assigned as shown.
Lycopladine C (26) showed the pseudomolecular ion peak at m/z 332 (M+H)+ in the ESI-MS, and the molecular formula, C19H25NO4, was established by HRESIMS [m/z 332.1858, (M+H)+, Δ −0.4 mmu]. IR absorptions implied the presence of ester carbonyl (1735 cm−1) functionality. Comparison of the 1H and 13C NMR spectra of 26 with those of 25 suggested that they were almost identical with each other except for the presence of signals for an acetate group [δH 2.04 (s, 3H) and δC 170.8 and 21.2] and low field shift of signal of H-5 (δH 4.67) in 26. The spectral data of the product which was obtained by acetylation of 25 were identical with those of 26. Thus, lycopladine C (26) was assigned as 5-O-acetyl form of lycopladine B (25).
Lycopladine D (27) showed the pseudomolecular ion peak at m/z 278 (M+H)+ in the ESI-MS, and the molecular formula, C16H23NO3, was established by HRESIMS [m/z 278.1773, (M+H)+, Δ +1.7 mmu]. IR absorptions implied the presence of hydroxy (3380 cm−1) and lactone carbonyl (1780 cm−1) functionalities. 13C NMR data of 27 revealed 16 carbon signals due to one ester carbonyl carbon, two sp3 quaternary carbons, four sp3 methines, and nine sp3 methylenes.
The gross structure of 27 was elucidated by analyses of 2D NMR data including the 1H–1H COSY, HOHAHA, HMQC, and HMBC spectra in CD3OD (Figure 9). The 1H–1H COSY and HOHAHA spectra of 3 revealed two structural units a (C-1 to C-8, C-14 to C-15, and C-8 to C-15) and b (C-9 to C-10). In unit a, C-5 was deduced to be connected to a hydroxy group from the 1H and 13C NMR data (δH 3.43, δC 74.0). HMBC cross-peaks of H-1a (δH 3.46) to C-9 (δC 46.6), and H-1b (δH 2.62) and H-9b (δH 3.05) to C-13 (δC 101.3) suggested connections of C-1 to C-9, C-1 to C-13, and C-9 to C-13 through a nitrogen atom. The δC value (101.3 ppm) suggested that C-13 was ascribed to aminoacetal carbon. HMBC correlations of H-8a (δH 2.09) and H-14a (δH 2.62) to C-16 (δC 179.0) revealed that the ester carbonyl group was connected to C-15. The connection of C-10 to C-11 was suggested by the HMBC cross-peak of H-11a (δH 1.91) to C-10 (δC 21.2). HMBC correlations of H-7 (δH 1.70) to C-11 (δC 34.1) and C-12 (δC 46.1) indicated that C-7 was connected to C-11 through C-12. HMBC cross-peaks of H-4 (δH 2.19) to C-12 (δC 46.1) and C-13 (δC 101.3), and H-14b (δH 2.00) to C-13 (δC 101.3) revealed that C-4 was connected to C-14 through C-12 and C-13. Thus, the gross structure of lycopladine D was elucidated to be 27.
The NOESY spectrum of 27 showed cross-peaks as shown in computer-generated 3D drawing (Figure 9). The relative stereochemistry of H-4 was deduced to be a β-configuration from NOESY correlations of H-1b/H-4, H-4/H-6b, and H-6b/H-8b, while the relative stereochemistry of H-5 and H-7 was deduced to be both β-configurations from NOESY correlations of H-3b/H-5, H-5/H-6a, H-5/H-7, and H-5/H-11a. A chair-like conformation of the piperidine ring (N-1 and C-9 to C-13) and the cyclohexane ring (C-7 to C-8 and C-12 to C-15) and relative stereochemistry of H-15 were suggested by NOESY correlations of H-8b/H-6b H-8a/H-14a, H-9a/H-11a, and H-9a/H2-14. Thus, the relative stereochemistry of lycopladine
D (27) was elucidated as shown in Figure 9.
The absolute configuration of lycopladine D (27) was elucidated by the modified Mosher’s method for the MTPA ester of 27. The values of Δδ[δ(S-MTPA ester)–δ(R-MTPA ester)] for H2-6, H2-8, and H-15 were positive, while the values of Δδ for H2-1, H2-2, H2-3, H-4, H2-9, H2-10, and H-14a were negative, suggesting that the absolute configuration at C-5 was R. Thus, the absolute configuration of 27 was assigned as shown.
Lycopladine E (28) was a new C16N-type alkaloid having a lycopodane-skeleton with N-oxide and acetoxy group at C-5. The relative and absolute stereochemistry were confirmed by 2D NMR technique and chemical correlation.
G. HUPERZININE RELATED ALKALOIDS
Two new Lycopodium alkaloids, huperzinine N-oxide (29) and 8,15-dihydrohuperzinine (30) were isolated from Lycopodium casuarinoides.47 Their relative and absolute stereochemistries were analyzed by spectroscopic data including CD spectra and chemical correlations.
In our search for bioactive Lycopodium alkaloids, lycoparins A−C (31-33), new alkaloids were isolated from the club moss L. casuarinoides.48
The club moss L. casuarinoides was extracted with MeOH, and the extract was partitioned between EtOAc and 3% tartaric acid. Water-soluble materials, which were adjusted to pH 10 with sat. Na2CO3, were extracted with CHCl3, and then n-BuOH. The aqueous layer was subjected to an HP-20 column (H2O/MeOH, 1:0 → 0:1), and then an ODS column (10% MeOH → MeOH), in which a fraction eluted with 30% MeOH was purified by an amino silica gel column (CHCl3/MeOH, 10:1) and then an ODS HPLC (13% CH3CN/0.1% TFA) to afford lycoparins A (31, 3.0 mg, 0.0002% yield), B (32, 3.0 mg, 0.0002% yield), and C (33, 7.6 mg, 0.0005%) together with huperzines B13, C73, and D73.
Lycoparin A {31, [α]D27 +1° (c 1.0, MeOH)} was revealed to have the molecular formula C17H20N2O3, by HRESITOFMS [m/z 301.1541 (M+H)+, Δ −1.2 mmu]. UV absorptions at 230 nm and 310 nm indicated the presence of α-pyridone ring. IR absorption implied the presence of α,β-unsaturated ketone (1660 cm−1) and a hydroxyl (3440 cm−1) group.
The gross structure of lycoparin A was deduced from detailed analyses of 2D NMR spectra of 31 (Figure 10). And the relative stereochemistry of 31 was elucidated by NOESY correlations as shown in computer-generated 3D drawing (Figure 10). The vinyl group at C-12 was elucidated to be α-configuration by the NOESY correlation of H-6a/H-11. Thus, the relative stereochemistry of 31 was assigned as shown in Figure 10. The absolute structure was deduced to be in Figure 11 by use of Flack parameter74 of the X-ray crystal structure of lycoparin A (31) TFA salt.
The relative stereochemistry of lycoparin B (32) was assigned to be an N-demethyl form of lycoparin A (31) by detailed analyses of 2D NMR data.
Lycoparin C (33), colorless amorphous solid, [α]D27 −12 (c 1.0, MeOH), was shown to have the molecular formula of C15H18N2O2 by HRESITOFMS [m/z 259.1429, (M+H)+, Δ −1.7 mmu], which was smaller than that of huperzine D by 28 mmu. 1H and 13C NMR data of 33 were analogous to those of huperzine D, although two N-methyl signals lacking for 33 was observed for huperzine D. The gross structure of 33 was elucidated by 2D NMR (1H−1H COSY, HMQC, HMBC) data, and relative stereochemistry of 33 was assigned as des-N-methyl form of huperzine D by NOESY correlation of H-6b and H-11 (Figure 12).
Lycoparin C (33) inhibited acetylcholinesterase (from bovine erythrocyte) with IC50 25 µM, whereas lycoparins A (31) and B (32) possessing a carboxylic acid at C-15 and one or two N-methyl groups did not show such activity (IC50>200 µM).75
H. CARINATUMINS
Three new Lycopodium alkaloids, carinatumins A−C (34-36), have isolated from the club moss Lycopodium carinatum.49 Relative stereochemistry of carinatumins A (34) and B (35) were assigned as 10-hydroxyhuperzine A and 11-hydroxyhuperzine B, respectively. On the other hands, carinatumin C (36) was the same structure as lycoposerramine-X, isolated from Lycopodium serratum.51 Carinatumins A (34) and B (35) inhibited acetylcholinesterase with IC50 4.6 and 7.0 µM, respectively, whereas carinatumin C (36) did not show such activity (IC50 > 100 µM).
I. LYCOPOSERRAMINES
Takayama and co-workers are energetically studying Lycopodium alklaoids and many fawcettimane-type and fawcettidane-type alkaloids, a series of lycoposerramines were isolated from L. serratum in Japan.76-80
Recently, they isolated five new alkaloids, lycoposeramines-V (37), -W (38), -X (39), -Y (40), and -Z (41), having phlegmarine-related structures, from the club moss Lycopodium serratum and their structures were elucidated on the basis of spectroscopic analysis.50,51 Among them, the absolute configuration of lycoposerramines-V (37) and -W (38) were established by asymmetric total synthesis by themselves.50
J. NANKAKURINES
New Lycopodium alkaloids, nankakurine A (42) and B (43) were isolated from the club moss Lycopodium hamiltonii.52,53 Nankakurine A {42, [α]21D +16° (c 0.4, MeOH)} showed the pseudomolecular ion peak at m/z 263 (M + H)+ in the FABMS spectrum, and the molecular formula, C17H30N2, was established by HRFABMS [m/z 263.2497, (M + H)+, Δ +1.0 mmu].
The gross structure of 42 was deduced from extensive analyses of the two-dimensional NMR data, including the 1H−1H COSY, HOHAHA, HMQC, and HMBC spectra in CD3OD (Figure 13). The 1H-1H COSY and HOHAHA spectra in CD3OD revealed connectivities of two partial structures a (C-1−C-4) and b (C-6 to C-8, C-9−C-16, C-7 to C-12, and C-8 to C-15) as shown in Figure 13. HMBC correlations were observed for H-1 and H-4 to C-5 (δC 56.1), suggesting that C-1 and C-4 were connected to each other through C-5 and a nitrogen atom to form a piperidine ring. The connectivities of C-17 to C-9 and C-13 through a nitrogen atom were implied by HMBC correlations for H3-17 to C-9 (δC 58.5) and C-13 (δC 65.1). HMBC cross-peaks for H-9b and H-6a to C-5 (δC 56.1) indicated that a 3-aza-bicyclo[3.3.1]nonane ring was connected to a piperidine ring through a spiro carbon (C-5). 1H-1H correlations observed in the 1H-1H COSY and HOHAHA spectra indicated that a cyclohexane ring with a methyl at C-15 was connected to the 3-aza-bicyclo[3.3.1]nonane ring at C-7, C-12, and C-13. Thus, the gross structure of nankakurine A was elucidated to be 42 possessing an unprecedented caged skeleton consisting of a cyclohexane ring (C-7−C-8 and C-12−C-15) with a methyl group at C-15 and a 3-aza-bicyclo[3.3.1]nonane ring (C-5−C-7, C-9−C-13, and N-2) with a N-methyl group (C-17) connected to a piperidine ring (N-1 and C-1−C-5) through a spiro carbon at C-5.
Nankakurine B {43, [α]D19 +12° (c 1.0, MeOH)} showed the pseudomolecular ion peak at m/z 277 (M+H)+ in the FABMS, and the molecularformula, C18H32N2, was established by HRFABMS [m/z 277.2653 (M+H)+, Δ +0.9 mmu]. IR absorptions implied the presence of an amine (3300 cm−1) functionality. 13C NMR data resembled that of nankakurine A except for the excess of a signal for an N-methyl group. The gross structure of 43 was assigned as N-methyl form of nankakurine A by 2D NMR data.
The relative stereochemistry of 43 was elucidated by NOESY correlations and 3JH−H couplings as shown in gomputer-generated 3D drawing (Figure 14). Conformations of the piperidine ring (N-1, C-1−C-5), the bicyclo[3.3.1]nonane ring (C-5−C-7, C-9−C-13, and N-2), and the cyclohexane ring (C-7, C-8, and C-12−C-15), in which all of the 6-membered rings took chair forms, were deduced from NOESY correlations such as H3-18/H-2b and H-4a, H-11a/H-9a and H-13, and H-12/H-8a and H-14a as shown in Figure 14. The 13C high field shift at C-2 (δC 17.9) by its γ-gauche effect indicated that the N-methyl at C-18 took an axial orientation. Stereochemistry of the spiro carbon at C-5 was elucidated to be R* by the clear NOESY correlations of H-1b/H-6b, and H3-18/H-9b. Thus, the relative stereochemistry of 43 was assigned as shown in Figure 14. Treatment of nankakurine A (42) with HCOOH and HCHO, nankakurine B (43) was obtained, so nankakurine A is demethyl form of nankakurine B.
There are two possible conformational states for the piperidine ring of 42 and 43 in solution as shown in Figure 15. The conformational space was searched using MMFF force field81 implemented in the Macromodel program.82 Each of the lowest energy conformers belonging to two separate clusters are represented as 42a, 42b, 43a, and 43b. Each conformer possessed a chair conformation in the piperidine part, while the bicyclo[3.3.1]nonane ring and the cyclohexane ring adopted the same chair conformation. In the case of nankakurine B, 43b was abundant from the populations calculated for these two clusters (43a and 43b). On the other hand, 42a and 42b took a similar energy. These results of the simulations were consistent with the relative stereochemistry of 43 and the equilibrium to the more stable conformer 43b in CD3OD inferred on basis of the NMR data.
K. LYCONADIN B
Lyconadin B (44) isolated from L. complanatum,45 was assigned as 2,3-dihydro form of lyconadin A.72 Lyconadin B elevated NGF mRNA expression in 1321N1 human astrocytoma cells.
L. SENEPODINE F
A new class of C22N2-type Lycopodium alkaloids consisting of a decahydroquinoline and a quinolizidine ring, senepodine F (45, 0.01%) was isolated from the club moss Lycopodium chinense,54 together with known related alkaloids, senepodines A83 and E.84 The relative stereochemistry of 45 was determined by NOESY correlations for a deacetylated derivative.
M. LYCOPERINE A AND CRYPTADINES
Three novel C27N3-type pentacyclic Lycopodium alkaloids, lycoperine A (46) and cryptadines A (47) and B (48) consisting of two octahydroquinoline rings and a piperidine ring were isolated from the club moss Lycopodium hamiltonii55 and L. cryptomerinum,56 respectively.
Lycoperine A (46) was shown to have the molecular formula C31H49N3O2 by HRFABMS [m/z 496.3907, (M + H)+, Δ +0.4 mmu]. The IR spectrum was indicative of amide carbonyl (1629 cm−1) and amine (3440 cm−1) functionalities. 1H and 13C NMR spectra showed broad signals because of rotation of its N-acetyl moiety. Treatment of 46 with LiAlH4 afforded tetrahydrodeoxylycoperine A (52), which provided sharp signals on the 1H and 13C NMR spectra.
Tetrahydrodeoxylycoperine A (52) showed the pseudomolecular ion peak at m/z 468 (M + H)+. Analysis of the 1H and 13C NMR data and the HMQC spectrum of 52 revealed the presence of six sp3 methines, seventeen sp3 methylenes, four sp2 quaternary carbons, and four methyl groups. Among them, four sp3 methylenes (δC 52.5, δH 2.17 and 2.91; δC 52.4, δH 2.13 and 2.85; δC 47.3, δH 2.42 and 2.83; δC 47.2, δH 2.43 and 2.78) and four sp3 methines (δC 61.7, δH 2.84; δC 61.6, δH 2.75; δC 56.6, δH 2.70; δC 55.6, δH 2.69) were ascribed to those bearing a nitrogen atom.
The gross structure of 52 was deduced from extensive analyses of the 2D NMR data, including the 1H−1H COSY, HOHAHA, HMQC, and HMBC spectra in C6D6 (Figure 16). The 1H−1H COSY and HOHAHA spectra in C6D6 revealed connectivities of seven partial structures, a (C-2−C-4), b (C-6−C-9, C-12), c (C-11, C-13−C-17, C-11'), d (C-2'−C-4'), e (C-6'−C-9', C-12'), f (C-19−C-20), and g (C-13'−C-14'), as shown in Figure 16.
In the octahydroquinoline moiety, the connectivity of partial structures a and b revealed by the 1H−1H COSY and HOHAHA spectra was analyzed by the HMBC spectrum. HMBC correlations from H2-19 to C-2 (δC 52.5) and C-6 (δC 61.7) established the connection among C-2, C-6, and C-19 through a nitrogen atom. HMBC cross-peaks of H-4, H-7, and H-11 to C-5, of H-4 and H-11 to C-10, and of H-11 to C-9 indicated the connection among partial structures a, b, c, and four-substituted olefinic carbons assigned to C-5 and C-10, constructing the octahydroquinoline ring (C-2−C-10 and N-1) with a methyl group (C-12) at C-8. Another octahydroquinoline moiety (C-2'−C-10' and N-1') was analyzed in the same way as that metioned above. Thus, the gross structure of tetrahydrodeoxylycoperine A was assigned as 52.
The relative stereochemistry of 52 was elucidated by NOESY correlations and by comparison of chemical shifts with known piperidine derivatives. The NOESY correlation of H-6 to H-8 suggested that H-6 and H-8 were oriented to the same side (Figure 17). A similar incident was observed for another octahydroquinoline moiety (C-2'−C-10' and N-1'). The relative stereochemistry of the piperidine moiety (C-13−C-17 and N-18) was deduced from comparison of chemical shifts with andrachamine (53)85 and its analogue (54).85
The 1H signals assigned to the piperidine ring of 52 were observed at δ 2.70 (H-13) and δ 2.69 (H-17), and the 13C signals were observed at δ 56.6 (C-13), δ 25.6 (C-15), and δ 55.6 (C-17). The 13C signals of the trans-substituted piperidine analogue (54) of andrachamine were observed at higher fields than those of 52 and 53 (see Figure 18). On the other hand, andrachamine (53) with a cis-substituted piperidine ring showed 1H and 13C chemical shifts similar to those of 52. Thus, the piperidine ring (C-13−C-17 and N-18) of tetrahydrodeoxylycoperine A (52) was assigned as cis configuration. The gross relative stereochemistry of 52 was deduced from the 13C NMR spectrum. Because 52 is not a symmetrical structure (26 signals were observed in the 13C NMR spectrum of 52), the absolute configurations of the two octahydroquinoline moieties were elucidated to be the same. Consequently, the relative stereochemistry of lycoperine A consisting of the two octahydroquinoline rings with the same absolute stereochemistry and a piperdine ring was assigned as 46.
Relative stereochemistry for cryptadines A (47) and B (48) from L. cryptomerinum was elucidated on the basis of spectroscopic data, chemical transformations, and computational methods.
Lycoperine A (46) and cryptadines A (47) and B (48) exhibited an inhibitory activity against acetylcholinesterase at IC50 60.9, 106.3, and 18.5 µM, respectively.
N. COMPLANADINES
In 2000, we isolated a first dimeric alkaloid containing a lycodine-type C16N2 skeleton,86 complanadine A from Lycopodium complanatum.87 Further investigation of the extracts of L. complanatum resulted in the isolation of three new dimeric alkaloids, complanadines B (49),39 C (50),57 and D (51).57
Complanadine B (49), [α]D23 −13° (c 0.5, MeOH) showed the pseudomolecular ion peak at m/z 497 (M+H)+ in the FABMS, and the molecular formula, C32H40N4O, was established by HRFABMS (m/z 497.3259, [M+H]+, Δ −2.1 mmu). IR absorptions implied the presence of amine and conjugated ketone (3322 and 1697 cm−1, respectively) functionalities. 13C NMR data of 49 was analogous to those of complanadine A, although a carbonyl carbon instead of one sp3 methylene was observed for complanadine A. The gross structure of 49 was assigned as a new alkaloid consisting of lycodine86 and 6-oxolycodine units, in which C-1 in 6-oxolycodine was connected to C-2’ in lycodine.
The phase sensitive NOESY spectrum of 49 showed cross-peaks as shown in computer-generated 3D drawing (Figure 19). The relative configurations at C-7, C-12, C-13, and C-15 in part A were based on NOESY correlations of H-12/Hb-10, H-3/Hb-14, Ha-8/H-15, and Hb-8/H-12, while the piperidine and cyclohexane (C-7, C-8, and C-12−C-15) rings adopted both chair conformations. On the other hand, a NOESY correlation of H’-15/H’a-8 was also observed in addition of the corresponding NOESY correlations for part B. Thus, the relative stereostructure of complanadine B (49) was assigned as shown in Figure 19. The CD spectrum [λmax 230 (θ −9200), 260 (4000), 290 (3300), 315 (2300), and 350 (−2000) nm] of 49 in MeOH was similar to those [complanadine A: λmax 260 (θ 4500), 285 (1500), 295 (2000), and 315 (3000) nm; lycodine: λmax 250 (θ 5000), 280 (3000), and 325 (3000) nm] of complanadine A and lycodine except for a negative CD curve at 350 nm. According to the anti-octant sector rule88 applied to aromatic conjugated ketones, absolute configurations of the 6-oxolycodine part were assigned as 7R, 12R, 13R, and 15R.
Complanadine D (51) showed the pseudomolecular ion peak at m/z 487 (M+H)+ in the ESIMS, and the molecular formula, C32H46N4, was established by HRESIMS [m/z 487.3801, (M+H)+, Δ +1.6 mmu]. Most of 1H and 13C NMR signals of 51 seemed to be due to each half moiety [units C (C-1−C-16) and D (C-1’−C-16’)] of a dimeric compound, of which the 1H and 13C NMR spectra were similar to those of complanadine A, except for lacking NMR signals for one of two trisubstituted pyridine rings in complanadine A. The 1H−1H COSY and HOHAHA spectra of 51 revealed the connection of C-1−C-3 and HMBC correlations of H-1 (δH 4.44) and H2-3 (δH 1.96 and 1.57) to C-5 (δC 140.0), and H2-6 (δH 2.50 and 1.77) to C-4 (δC 98.6) and C-5, indicated the existence of a trisubstituted tetrahydropyridine ring in unit C. The connection between the tetrahydropyridine ring in unit C and a pyridine ring in unit D was provided by HMBC correlations of H2-2 (δH 2.14 and 1.96) to C-2’ (δC 140.2), and H-1’ (δH 8.24) and H-3’ (δH 7.89) to C-1 (δC 52.9) (Figure 20). Thus, the gross structure of complanadine D (51) was assigned as N-1,1,2,3-tetrahydro form of complanadine A.
The NOESY spectrum of 51 was similar to that of complanadine A, suggesting that the relative stereochemistry was the same as that of complanadine A except for the tetrahydropyridine ring in unit C. Analysis of the NOESY spectrum of 51 revealed a pseudochair form of the tetrahydropyridine ring (N-1 and C-1−C-5) and an α-configuration of H-1 (Figure 21).
Complanadine C (50) showed the pseudomolecular ion peak at m/z 503 (M+H)+ in the ESIMS, and the molecular formula, C32H42N2O3, was established by HRESIMS [m/z 503.3270, (M+H)+, Δ −0.4 mmu].
The gross structure of 50 was elucidated by analyses of 2D NMR data including the 1H–1H COSY, HOHAHA, HMQC, and HMBC spectra in CD3OD (Figure 22). Most of 1H and 13C NMR signals appeared to be due to each half moiety (units A and B) of a dimeric compound. In unit A (C-1−C-16), 1H-1H COSY and HOHAHA spectra revealed connectivities of C-1−C-4, C-7−C-8, C-7−C-12, C-8−C-15, C-11−C-12, and C-14−C-16. HMBC correlations of H-4 (δH 2.70) to C-5 (δC 209.9) and C-6 (δC 43.3), H-6b (δH 2.29) to C-5, and H-8b (δH 1.38) and H-12 (δH 2.73) to C-6 suggested connections of C-4 to C-6 through C-5 and C-6 to C-8 through C-7. Connections of C-4 to C-12, C-4 to C-14, and C-12 to C-14 through C-13 were deduced from HMBC cross-peaks of H-12 to C-13 (δC 61.7), H-14b (δH 1.16) to C-4 (δC 50.0) and C-13. HMBC cross-peaks of H-1b (δH 3.08) to C-9 (δC 166.5), H-11 (δH 5.96) to C-9, and H-12 (δH 2.73) to C-10 (δC 135.1) indicated connections of C-1 to C-9 through a nitrogen atom and C-9 to C-11 through C-10. In unit B (C-1’−C-16’), the 1H-1H COSY and HOHAHA spectra of 1 revealed three partial structures C-1’−C-4’, C-8’−C-15’ and C-14’−C-16’, and C-11’−C-12’. HMBC correlations of H-4’ (δH 2.62) and H-6’b (δH 2.23) to C-5’ (δC 211.7) suggested the connection of C-4’ to C-6’ through C-5’. Connections of C-6’ to C-12’, C-6’ to C-8’, and C-8’ to C-12’ through C-7’ were deduced from HMBC cross-peaks of H-6’b to C-12’ (δC 40.5), and H-8’b (δH 1.30) to C-6’ (δC 42.4) and C-12’. HMBC cross-peaks of H-4’ to C-13’ (δC 57.3) and H-14’a (δH 2.56) to C-12’ and C-13’ indicated connections of C-4’ to C-12’, C-4’ to C-14’, and C-12’ to C-14’ through C-13’. Connections of C-1’ to C-9’, C-1’ to C-13’, and C-9’ to C-13’ through a nitrogen atom were suggested from HMBC cross-peaks of H-1’b (δH 2.92) to C-9’ (δC 137.2) and C-13’, and H-9’ (δH 7.22) to C-13’. HMBC cross-peaks of H-9’ to C-11’ (δC 25.9) and H-11’a (δH 2.55) to C-10’ (δC 103.4) revealed the connection of C-9’ to C-11’ through C-10’. The connection of units A and B was provided from HMBC correlations of H-11 to C-10’ and H-9’ to C-10. Thus, the gross structure of complanadine C was elucidated to be 50.
The NOESY spectrum of 50 showed cross-peaks as shown in computer-generated 3D drawing (Figure 23). In unit A, a chair-like conformation of a piperidine ring (N-1, C-1−C-4, and C-13) was suggested from NOESY correlations of H-14a to H-1b and H-3b. NOESY cross-peaks and 3JH-14/H-15 (12.6 Hz) indicated a chair conformation of a cyclohexane ring (C-7−C-8 and C-12−C-15). In unit B, NOESY correlations of H-14’a to H-1’ and H-3’b suggested a chair-like conformation of a piperidine ring (N-1’, C-1’−C-4’, and C-13’). A chair conformation of a cyclohexane ring (C-7’−C-8’ and C-12’−C-15’) was deduced from NOESY correlations of H-8’ to H-16’, and H-12’ to H-8’b and H-14’b. Thus, the partial relative stereochemistry of complanadine C (50) was elucidated as shown in Figure 23.
Complanadine C (50) is the first dimeric Lycopodium alkaloid containing a lycopodane-type C16N skeleton, while complanadine B (49) and D (51) are structure analogue of complanadine A. Effects of complanadine D (51) on neurotrophic factor biosynthesis in 1321N1 human astrocytoma cells were examined by a semiquantitative RT-PCR method, and it was found that the mRNA expressions for NGF were enhanced by 51. Complanadine D (51) exhibited cytotoxicity against murine leukemia L1210 cells (IC50, 7 µg/ml) in vitro, while 50 did not show such activity (IC50 > 10 µg/ml). Complanadines C (50) and D (51) showed antimicrobial activity against Cryptococcus neoformans (MIC, 0.52 and 0.26 µg/ml, respectively) and Aspergillus niger (MIC, 2.05 and 4.16 µg/ml, respectively).
TOTAL SYNTHESIS
CERMIZINE C AND SENEPODINE G
Cermizine C (55)89 and senepodine G (56)89 are new class of C11N Lycopodium alkaloids, consisting of a quinolizidine ring, isolated from Lycopodium cernuum and L. chinense, respectively. An efficient, stereospecific synthesis of these alkaloids has been completed by Snider and Grabowski using the conjugate addition of Me2CuLi to α,β-unsaturated lactam 59 to provide lactam 60 to senepodine G (56, 6 steps, 40% overall yield), and NaBH4 reduction of 56 to give cermizine C (55, 7 steps, 40% overall yield).90
(+)-FAWCETTIDINE
Fawcettidine (61)91,92 was isolated by Burnell and co-workers from Lycopodium fawcetti in the late 1950s. Recently, Dake and Kozak reported first total synthesis of this alkaloids by 16 steps in 1% overall yield.93 They used (R)-(+)-pulegone (62) as a starting material and employed platinum(II)-catalyzed annulation and a one-pot Ramberg-Bäcklund reaction for the key steps.
(−)-MAGELLANINE, (+)-MAGELLANINONE, AND (+)-PANICULATINE
Three alkaloids, magellanine (72),94 magellaninone (73),95 and paniculatine (74),96,97 which possess a highly condensed tetracyclic nucleus with five to seven stereogenic cneters. Unique and challenging structures of magellanine skeleton has evoked a great of attention from the synthetic community, and several studies have been directed toward its total synthesis.98-104 Stereoselective total synthesis of (−)-magellanine (72), (+)-magellaninone (73), and (+)-paniculatine (74), from diethyl L-tartrate (72, 43 steps, 1.7% overall yield; 73, 43 steps, 1.9% overall yield; 74, 45 steps, 2.8% overall yield).105 The key reactions in these syntheses involved two intramolecular Pauson-Khand reactions of enynes.
CERNUINE AND CERMIZINE D
The first total syntheses of two cernuane-type lycopodium alkaloids, (−)-cernuine (92)106-110 and (+)-cermizine D (93),89 were accomplished by Takayama and co-workers.111 They used (+)-citronellal (94) as a starting material and achieved syntheses of them via an organocatalytic α-amination and a transter aminoallylation (92, 19 steps, 11.0% overall yield; 93, 18 steps, 13.9% overall yield).
LYCOPOSERRAMINES-V AND -W
Lycoposerramines-V (37) and -W (38) were isolated from Lycopodium serratum by Takayama and co-workers.50 These alkaloids possess partially aromatized phlegmarane skeleton21 and their structures including the absolute configuration were established by asymmetric total synthesis involving Johnson-Claisen rearrangement and ring-closing metathesis or SmI2-mediated stereoselective piperidine ring construction (37, 22 steps, 4.3% overall yield; 38, 23 steps, 1.4% overall yield).50
LYCONADINS A AND B
We isolated two novel alkaloids (+)-lyconadin A (131)72 and (−)-lyconadin B (44)45 from Lycopodium complanatum in 2001 and 2006, respectively. These alkaloids contains unprecedented pentacyclic ring system, with either an α-pyridone or 3,4-dihydro-α-pyridinone ring and attracted great interest from biogenetic, synthetic, and biological points of view. Recently, the first total synthesis of (+)-lyconadin A (131) and (−)-lyconadin B (44) were accomplished by Smith’s group.112 They chose (−)-methyl (R)-3-methylglutarate (132) and (−)-carboxylic acid (136) as starting materials. 142 was obtained from hydrazone 135 and iodide 141 in the presence of nBuLi and HMPA. Intramolecular aldol/conjugate addition of 144 led to tricyclic ketone 147, after removal of Cbz group and reflux in water/methanol/HCl induced epimerization at C-12. Aminoiodination of alkene 152 with N-iodosuccinimide (NIS) furnished crystalline iodide 153, followed by construction of β-ketoester and Michael addition of propiolamide provided 155. Application of novel one-pot protocol involving decarboxylation, mediated by Me4NOAc, olefin isomerization, and cyclocondensation, total synthesis of lyconadin A was achieved (131, 22 steps, 4.3% overall yield). Hydrogenation of 155 followed by a similar one-pot protocol, lyconadin B was generated (44, 22 steps, 4.3% overall yield).
The second total synthesis of lyconadin A was achieved by Sarpong and co-workers.113 They selected readily available picoline derivative 156 and vinylogous ester 158 as a starting materials, and utilized unique proximity-driven oxidative C−N bond-forming reaction for constructing the caged pentacycle 169. The total synthesis of lyconadin A (131) was accomplshed by 18 steps in 10% overall yield.
CONCLUSIONS
Studies on the Lycopodium alkaloids from 2004 to July in 2008 have been reviewed, particularly focusing on recent developments in the synthesis of these alkaloids, the structures of the new types of alkaloids, such as the lycopodatines, lycovatine A, lannotinidines, lycopladines, lycoparins, carinatumins, lycoposerramines, nankakurines, lyconadins, senepodines, lycoperine A, cryptadines, and complanadines. There are currently more than 250 Lycopodium alkaloids of known structure. Huperzine A, a representative Lycopodium alkaloid, is a highly specific and potent inhibitor of acetylcholinesterase, and the inherent inhibition of acetylcholinesterase has poromoted the pursuit of the total synthesis and SAR studies of this alkaloid. Further phytochemical investigations will bring increasing structural variation to this alkaloid group. Although the total syntheses of some of the C16N and C16N2 type skeletons have been accomplished, the other skeletal variants remain attractive targets.
ACKNOWLEDGMENTS
Our isolation work, including structure elucidation, was partly supported by a Grant-in-Aid for Scientific Research from the Ministry of Education, Culture, Sports, Science, and Technology of Japan, and a grant from The Open Research Center Project.
†Dedicated to Professor Emeritus Keiichiro Fukumoto on the occasion of his 75th birthday.
References
1. D. B. MacLean, The Alkaloids, ed. by R. H. F. Manske, Academic: New York, 1968,Vol. 10, p. 305.
2. D. B. MacLean, The Alkaloids, ed. by A. Brossi, Academic: New York, 1973,Vol. 14, p. 348.
3. D. B. MacLean, The Alkaloids, ed. by A. Brossi; Academic: New York, 1985; Vol. 26, p. 241.
4. W. A. Ayer, Nat. Prod. Rep., 1991, 8, 455. CrossRef
5. W. A. Ayer and L. S. Trifonov, The Alkaloids, ed. by G. A. Cordell and A. Brossi; Academic: New York, 1994; Vol. 45, p. 233.
6. X. Ma and D. R. Gang, Nat. Prod. Rep., 2004, 21, 752. CrossRef
7. J. Kobayashi and H. Morita, The Alkaloids, ed. by G. A. Cordell; Academic: New York, 2005; Vol. 61, p. 1.
8. N. Wikström and P. Kenrick,Syst. Bot., 2000, 25, 495. CrossRef
9. N. Wikström, Am. Fern J., 2001, 91, 150. CrossRef
10. J. S. Liu, Y. L. Zhu, C. M. Yu, Y. Z. Zhou, Y. Y. Han, Y. Y. Wu, and B. F. Zi, Can. J. Chem., 1986, 64, 837. CrossRef
11. A. P. Kozikowski and W. Tüeckmantel, Acc. Chem. Res., 1999, 32, 641. CrossRef
12. D. L. Bai, X. C. Tang, and X. C. He, Curr. Med. Chem., 2000, 7, 355.
13. C. H. Tan and D. Y. Zhu, Zhongguo Tianran Yaowu, 2003, 1, 1.
14. F. Yamada, A. P. Kozikowski, E. R. Reddy, Y. P. Pang, J. H. Miller, and M. McKinney, J. Am. Chem. Soc., 1991, 113, 4695. CrossRef
15. A. P. Kozikowski, G. Campiani, P. Aagaard, and M. McKinney, J. Chem. Soc., Chem. Commun., 1993, 860. CrossRef
16. G. Campiani, L. Q. Sun, A. P. Kozikowski, P. Aagaard, and M. McKinney, J. Org. Chem., 1993, 58, 7660. CrossRef
17. S. Kaneko, T. Yoshino, T. Katoh, and S. Terashima, Tetrahedron, 1998, 54, 5471. CrossRef
18. S. Kaneko, T. Yoshino, T. Katoh, and S. Terashima, Heterocycles, 1997, 46, 27. CrossRef
19. S. Kaneko, T. Yoshino, T. Katoh, and S. Terashima, Tetrahedron: Asymmetry, 1997, 8, 829. CrossRef
20. W. A. Ayer, N. Masaki, and D. S. Nkunika, Can. J. Chem., 1968, 46, 3631. CrossRef
21. L. Nyembo, A. Goffin, C. Hootelé, and J. C. Braekman, Can. J. Chem., 1978, 56, 851. CrossRef
22. H. Conroy, Tetrahedron Lett., 1960, 1(31), 34. CrossRef
23. Y. Maki, Gifu Yakka Daigaku Kiyo, 1961, 11, 1.
24. R. N. Gupta, Lloydia, 1968, 31, 318.
25. R. N. Gupta, M. Castillo, D. B. MacLean, I. D. Spenser, and J. T. Wrobel, J. Am. Chem. Soc., 1968, 90, 1360. CrossRef
26. M. Castillo, R. N. Gupta, Y. K. Ho, D. B. MacLean, and I. D. Spenser, Can. J. Chem., 1970, 48, 2911. CrossRef
27. M. Castillo, R. N. Gupta, Y. K. Ho, D. B. MacLean, and I. D. Spenser, J. Am. Chem. Soc., 1970, 92, 1074. CrossRef
28. M. Castillo, R. N. Gupta, D. B. MacLean, and I. D. Spenser, Can. J. Chem., 1970, 48, 1893. CrossRef
29. R. N. Gupta, Y. K. Ho, D. B. MacLean, and I. D. Spenser, J. Chem. Soc., Sect. D, 1970, 409.
30. R. B. Herbert, Alkaloids (London), 1971, 1, 1. CrossRef
31. Y. K. Ho, R. N. Gupta, D. B. MacLean, and I. D. Spenser, Can. J. Chem., 1971, 49, 3352. CrossRef
32. J. C. Braekman, R. N. Gupta, D. B. MacLean, and I. D. Spenser, Can. J. Chem., 1972, 50, 2591. CrossRef
33. W. Marshall, T. Nguyen, D. B. MacLean, and I. D. Spenser, Can. J. Chem., 1975, 53, 41. CrossRef
34. T. Hemscheidt and I. D. Spenser, J. Am. Chem. Soc., 1990, 112, 6360. CrossRef
35. T. Hemscheidt and I. D. Spenser, J. Am. Chem. Soc., 1993, 115, 3020. CrossRef
36. T. Hemscheidt and I. D. Spenser, J. Am. Chem. Soc., 1996, 118, 1799. CrossRef
37. C. H. Tan and D. Y. Zhu, Helv. Chim. Acta, 2004, 87, 1963. CrossRef
38. M. G. Ortega, A. M. Agnese, and J. L. Cabrera, Tetrahedron Lett., 2004, 45, 7003. CrossRef
39. H. Morita, K. Ishiuchi, A. Haganuma, T. Hoshino, Y. Obara, N. Nakahata, and J. Kobayashi, Tetrahedron, 2005, 61, 1955. CrossRef
40. H. Morita, Y. Hirasawa, and J. Kobayashi, J. Nat. Prod., 2005, 68, 1809. CrossRef
41. K. Katakawa, A. Nozoe, N. Kogure, M. Kitajima, M. Hosokawa, and H. Takayama, J. Nat. Prod., 2007, 70, 1024. CrossRef
42. T. Kubota, T. Sunaura, H. Morita, Y. Mikami, T. Hoshino, Y. Obara, N. Nakahata, and J. Kobayashi, Heterocycles, 2006, 69, 469. CrossRef
43. K. Koyama, H. Morita, Y. Hirasawa, M. Yoshinaga, T. Hoshino, Y. Obara, N. Nakahata, and J. Kobayashi, Tetrahedron, 2005, 61, 3681. CrossRef
44. K. Ishiuchi, T. Kubota, H. Morita, and J. Kobayashi, Tetrahedron Lett., 2006, 47, 3287. CrossRef
45. K. Ishiuchi, T. Kubota, T. Hoshino, Y. Obara, N. Nakahata, and J. Kobayashi, Bioorg. Med. Chem., 2006, 14, 5995. CrossRef
46. T. Kubota, H. Yahata, K. Ishiuchi, Y. Obara, N. Nakahata, and J. Kobayashi, Heterocycles, 2007, 74, 843. CrossRef
47. S. Yin, C. Q. Fan, X. N. Wang, and J. M. Yue, Helv. Chim. Acta, 2006, 89, 138. CrossRef
48. Y. Hirasawa, E. Kato, J. Kobayashi, N. Kawahara, Y. Goda, M. Shiro, and H. Morita, Bioorg. Med. Chem., 2008, 16, 6167. CrossRef
49. C. Y. Choo, Y. Hirasawa, C. Karimata, K. Koyama, M. Sekiguchi, J. Kobayashi, and H. Morita, Bioorg. Med. Chem., 2007, 15, 1703. CrossRef
50. T. Shigeyama, K. Katakawa, N. Kogure, M. Kitajima, and H. Takayama, Org. Lett., 2007, 9, 4069. CrossRef
51. K. Katakawa, M. Kitajima, K. Yamaguchi, and H. Takayama, Heterocycles, 2006, 69, 223. CrossRef
52. Y. Hirasawa, H. Morita, and J. Kobayashi, Org. Lett., 2004, 6, 3389. CrossRef
53. Y. Hirasawa, J. Kobayashi, Y. Obara, N. Nakahata, N. Kawahara, Y. Goda, and H. Morita, Heterocycles, 2006, 68, 2357.
54. Y. Hirasawa, H. Morita, and J. Kobayashi, Heterocycles, 2004, 64, 515.
55. Y. Hirasawa, J. Kobayashi, and H. Morita, Org. Lett., 2006, 8, 123. CrossRef
56. K. Koyama, Y. Hirasawa, J. Kobayashi, and H. Morita, Bioorg. Med. Chem., 2007, 15, 7803. CrossRef
57. K. Ishiuchi, T. Kubota, Y. Mikami, Y. Obara, N. Nakahata, and J. Kobayashi, Bioorg. Med. Chem., 2007, 15, 413. CrossRef
58. W. A. Ayer and G. G. Iverach, Can. J. Chem., 1964, 42, 2514. CrossRef
59. R. H. Burnell, B. S. Mootoo, and D. R. Taylor, Can. J. Chem., 1960, 38, 1927. CrossRef
60. J. C. Braekman, C. Hootelé, and W. A. Ayer, Bull. Soc. Chim. Belg., 1971, 80, 83.
61. W. A. Ayer, B. Altenkirk, N. Masaki, and S. Valverde-Lopez, Can. J. Chem., 1969, 47, 2449. CrossRef
62. W. A. Ayer, B. Altenkirk, S. Valverde-Lopez, B. Douglas, R. F. Raffauf, and J. A. Weisbach, Can. J. Chem., 1968, 46, 15.
63. I. Ohtani, T. Kusumi, Y. Kashman, and H. Kakisawa, J. Am. Chem. Soc., 1991, 113, 4092. CrossRef
64. Y. Obara, H. Kobayashi, T. Ohta, Y. Ohizumi, and N. Nakahata, Mol. Pharmacol., 2001, 59, 1287.
65. F. A. L. Amet, M. Z. Haq, N. H. Khan, W. A. Ayer, R. Hayatsu, and S. Valverde-Lopez, Tetrahedron Lett., 1964, 5, 751. CrossRef
66. W. A. Ayer, G. G. Iverach, J. K. Jenkins, and N. Masaki, Tetrahedron Lett., 1968, 9, 4597. CrossRef
67. Y. Hirasawa, H. Morita, and J. Kobayashi, Tetrahedron, 2002, 58, 5483. CrossRef
68. H. Burnell and B. S. Mootoo, Can. J. Chem., 1961, 39, 1090. CrossRef
69. R. H. Burnell and D. R. Taylor, Tetrahedron, 1962, 18, 1467. CrossRef
70. R. H. F. Manske and L. Marion, Can. J. Res., 1943, B21, 92.
71. K. Wiesner, J. E. Francis, J. A. Findlay, and Z. Valenta, Tetrahedron Lett., 1961, 2, 187. CrossRef
72. J. Kobayashi, Y. Hirasawa, N. Yoshida, and H. Morita, J. Org. Chem., 2001, 66, 5901. CrossRef
73. J. S. Liu and M. F. Huang, Phytochemistry, 1994, 37, 1759. CrossRef
74. H. D. Flack, Acta Cryst., 1983, A39, 876.
75. G. L. Ellman, K. D. Courtney, V. Anders, and R. M. Featherstone, Biochem. Pharmacol., 1961, 7, 88. CrossRef
76. H. Takayama, K. Katakawa, M. Kitajima, H. Seki, K. Yamaguchi, and N. Aimi, Org. Lett., 2001, 3, 4165. CrossRef
77. H. Takayama, K. Katakawa, M. Kitajima, H. Seki, K. Yamaguchi, and N. Aimi, Org. Lett., 2002, 4, 1243. CrossRef
78. H. Takayama, K. Katakawa, M. Kitajima, K. Yamaguchi, and N. Aimi, Tetrahedron Lett., 2002, 43, 8307. CrossRef
79. H. Takayama, K. Katakawa, M. Kitajima, K. Yamaguchi, and N. Aimi, Chem. Pharm. Bull., 2003, 51, 1163. CrossRef
80. K. Katakawa, M. Kitajima, N. Aimi, H. Seki, K. Yamaguchi, K. Furihata, T. Harayama, and H. Takayama, J. Org. Chem., 2005, 70, 658. CrossRef
81. T. Halgren, J. Am. Chem. Soc., 1990, 112, 4710. CrossRef
82. F. Mohamadi, N. G. J. Richards, W. C. Guida, R. Liskamp, M. Lipton, C. Caufield, G. Chang, T. Hendrickson, and W. C. J. Still, J. Comput. Chem., 1990, 11, 440. CrossRef
83. H. Morita, Y. Hirasawa, N. Yoshida, and J. Kobayashi, Tetrahedron Lett., 2001, 42, 4199. CrossRef
84. Y. Hirasawa, H. Morita, and J. Kobayashi, Tetrahedron, 2003, 59, 3567. CrossRef
85. S. Mill and C. Hootelé, Can. J. Chem., 1996, 74, 2434. CrossRef
86. F. A. L. Anet and C. R. Eves, Can. J. Chem., 1958, 36, 902. CrossRef
87. J. Kobayashi, Y. Hirasawa, N. Yoshida, and H. Morita, Tetrahedron Lett., 2000, 41, 9069. CrossRef
88. J. Barry, H. B. Kagan, and G. Shatzke, Tetrahedron, 1971, 27, 4737. CrossRef
89. H. Morita, Y. Hirasawa, T. Shinzato, and J. Kobayashi, Tetrahedron, 2004, 60, 7015. CrossRef
90. B. B. Snider and J. F. Grabowski, J. Org. Chem., 2007, 72, 1039. CrossRef
91. R. H. Burnell, J. Chem. Soc., 1959, 3091.
92. R. H. Burnell, C. G. Chin, B. S. Mootoo, and D. R. Taylor, Can. J. Chem., 1963, 41, 3091. CrossRef
93. J. A. Kozak and G. R. Dake, Angew. Chem. Int. Ed., 2008, 47, 4221. CrossRef
94. M. Castillo, L. A. Loyola, G. Morales, I. Singh, C. Calvo, H. L. Holland, and D. B. MacLean, Can. J. Chem., 1976, 54, 2893. CrossRef
95. L. A. Loyola, G. Morales and M. Castillo, Phytochemistry, 1979, 18, 1721. CrossRef
96. M. Castillo, G. Morales, L. A. Loyola, I. Singh, C. Calvo, H. L. Holland, and D. B. MacLean, Can. J. Chem., 1975, 53, 2513. CrossRef
97. M. Castillo, G. Morales, L. A. Loyola, I. Singh, C. Calvo, H. L. Holland, and D. B. MacLean, Can. J. Chem., 1976, 54, 2900. CrossRef
98. G. C. Hirst, T. O. Johnson, Jr, and L. E. Overman, J. Am. Chem. Soc., 1993, 115, 2992. CrossRef
99. L. A. Paquette, D. Friedrich, E. Pinard, J. P. Williams, D. St. Laurent, and B. A. Roden, J. Am. Chem. Soc., 1993, 115, 4377. CrossRef
100. J. P. Williams, D. R. St. Laurent, E. Pinard, B. A. Roden, and L. A. Paquette, J. Am. Chem. Soc., 1994, 116, 4689. CrossRef
101. C. F. Yen and C. C. Liao, Angew. Chem. Int. Ed., 2002, 41, 4090. CrossRef
102. M. Ishizaki, Y. Niimi, and O. Hoshino, Tetrahedron Lett., 2003, 44, 6029. CrossRef
103. M. Ishizaki, Y. Niimi, O. Hoshino, H. Hara, and Y. Takahashi, Tetrahedron, 2005, 61, 4053. CrossRef
104. C. K. Sha, F. K. Lee, and C. J. Chang, J. Am. Chem. Soc., 1999, 121, 9875. CrossRef
105. T. Kozaka, N. Miyakoshi, and C. Mukai, J. Org. Chem., 2007, 72, 10147. CrossRef
106. L. Marion and R. H. F. Manske, Can. J. Res., 1948, 26, 1.
107. W. A. Ayer, J. K. Jenkins, and S. Valverde-Lopez, Tetrahedron Lett., 1964, 5, 2201. CrossRef
108. W. A. Ayer, J. K. Jenkins, S. Valverde-Lopez, and R. H. Burnell, Can. J. Chem., 1967, 45, 433. CrossRef
109. W. A. Ayer, J. K. Jenkins, K. Piers, and S. Valverde-Lopez, Can. J. Chem., 1967, 45, 445. CrossRef
110. W. A. Ayer and K. Piers, Can. J. Chem., 1967, 45, 451. CrossRef
111. Y. Nishikawa, M. Kitajima, and H. Takayama, Org. Lett., 2008, 10, 1987. CrossRef
112. D. C. Beshore and A. B. Smith, J. Am. Chem. Soc., 2007, 129, 4148. CrossRef
113. A. Bisai, S. P. West, and R. Sarpong, J. Ame. Chem. Soc., 2008, 130, 7222. CrossRef