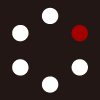
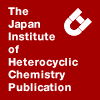
HETEROCYCLES
An International Journal for Reviews and Communications in Heterocyclic ChemistryWeb Edition ISSN: 1881-0942
Published online by The Japan Institute of Heterocyclic Chemistry
e-Journal
Full Text HTML
Received, 9th April, 2008, Accepted, 18th August, 2008, Published online, 21st August, 2008.
DOI: 10.3987/COM-08-S(F)9
■ Benzothiazines in Organic Synthesis. An Approach to the Synthesis of seco-Pseudopteroxazole
Michael Harmata* and Pinguan Zheng
Room 125, Department of Chemistry, University of Missouri-Columbia, 601 South College Avenue, Columbia, Missouri 65211, U.S.A.
Abstract
As part of our approach to the synthesis of seco-pseudopteroxazole, we have succeeded in establishing the stereochemistry of the methyl-bearing stereocenter (C-11) with good diastereoselectivity. Though seemingly straightforward, the process was not simple and revealed some interesting aspects of benzothiazine chemistry.INTRODUCTION
We recently reported the synthesis of the antitubercular agent pseudopteroxazole (1)1 using benzothiazine chemistry developed in our laboratories.2 One difficulty in that process was establishing the configuration
of the C-11stereocenter in 1. The reaction of enantiomerically pure 3 with LDA afforded 4 as a 10:1 mixture of diastereomers, unfortunately in the direction opposite to that desired (Scheme 1).
While steps could be taken to amerliorate this situation so that 1 could be synthesized, we wanted to develop one or more routes that would avoid this complication. We chose to do that in the context of the synthesis of the natural product seco-pseudopteroxazole (2).
Seco-pseudopteroxazole (2) was isolated by Rodriguez and coworkers from Pseudopterogorgia elisabethae.3 Like pseudopteroxazole, it showed antituberculosis activity, though its potency was less than that of pseudopteroxazole. No synthesis of seco-pseudopteroxazole has been reported, and this target appeared to be one through which we could demonstrate new strategies for establishing the common stereochemical centers at C-4 and C-11 of both pseudopteroxazole and seco-pseudopteroxazole.
While several approaches can be conceived for the establishment of the appropriate C-4/ C-11 relationship, we chose to simply reverse the roles of the substituents on the α,β-unsaturated ester of the benzothiazine precursor, relative to how those were used in the synthesis of pseudopteroxazole.
RESULTS AND DISCUSSION
We began with aldehyde 5, which is readily prepared from 3,5-dimethylanisole in a 4-step sequence.4 A Horner-Emmons reaction with known phosphonate 65 using the modification introduced by Roush and
Masamune6 afforded the ester 7 in 83% yield along with a 10% yield of the corresponding Z isomer. Coupling of this compound with enantiomerically pure sulfoximine 8 under standard conditions led to sulfoximine 9 in 99% yield (Scheme 2).7 We were thus prepared to develop an intramolecular conjugate addition/protonation sequence that would be highly diastereoselective.
Based on earlier results,1 we knew that the key to selectivity would be the protic quench itself. A selection of quenching procedures attempted are illustrated in Table 1.
Initial attempts utilizing cold methanol as quenching reagent afforded the desired product in good yield, but with only fair diastereoselectivity. This might result from the rapid epimerization at C-11 position during the protonation of enolate, due to the formation of methoxide. A stronger proton donor was necessary to achieve better stereoselectivity. When the reaction was quenched by rapid addition of cold 1N HCl in methanol solution at –78 oC, the product was obtained in quantitative yield with a diastereomeric ratio of up to 9.2:1.0 (entry 4). Interestingly, a sterically-hindered proton donor (diisopropylamine-HCl) afforded product with only moderate diastereoselectivity (entry 6). Further, the reaction utilizing LDA as a base generally gave lower diastereoselectivity than LiHMDS, which might suggest that the role of free amines (HMDS and DIPA) in the reaction mixture should not be overlooked (entries 3 and 5). The stereochemical outcome of the reaction could be rationalized by a model analogous to one developed by Kocienski (11, Figure 2).8 Protonation on the Re face of 11 avoids an untoward interaction with the hydrogen on the aryl ring (Figure 2) and is thus favored.
After successfully establishing the stereocenters at carbons 4 and 11 (2 numbering), we set out to convert the methyl ester in 10 to a methyl group. One step procedures were not successful.9 LAH reduction gave only moderate yields (43-57%) of the corresponding alcohol after silica gel column chromatography, despite the fact that relatively clean conversion was achieved on a 200 mg scale (entry 1, Table 2). The low yield of this reaction might be attributed to the lability of alcohol 12, which was prone to undergo decomposition under the reaction conditions and during silica gel chromatography.
Compound 10 afforded a complex mixture of products upon with the treatment of Red-Al (Table 2, entry 2). Attempted reduction with DIBAL afforded product in 46% yield along with a small amount of starting material (Table 2, entry 3). LiEt3BH10 was found to work perfectly to give a quantitative yield of alcohol, which was directly converted to iodide 16 in 80-89% yield (Table 2, entries 4 and 5).11 Other substrates (13-15), bearing different sulfonate functionalities, were also prepared from 12 in good yields.
Substrates 13-16 were subjected to different conditions of reductive cleavage of the C-X bond (Table 3). When 13 was first exposed to 3.0 equivalents of LAH at –25 oC, compound 17 was obtained in 45% yield (60% based on recorvered starting material). A 15% yield of overreduction product 18 was isolated (entry 1). Compound 13 reacted very slowly upon treatment of LiEt3BH or LiAlH(OMe)3 combined with CuI12 (entries 2 and 3). An unidentified complex mixture was obtained when 13 was treated with lithium dimethylaminoborohydride and 20% Et3B13 (entry 4). NaBH4 dissolved in DMSO14 was also applied to the deoxygenation of 13. A 71% yield of 17 was obtained in a small-scale reaction, but the yield dropped precipitously on a larger scale. When sulfonate 1415 was treated with either LAH or LiBHEt3, only decomposition of starting material was observed (entries 6 and 7). When tosylate 15 was subjected to reaction with LAH at 0 oC, significant amounts of over-reduction products 18 and 19 were isolated (entry 8).16 A lower temperature (-25 oC) and lower loading of LAH suppressed the side reactions leading to these compounds (entries 9 and 10), but the yield of 17 was still not satisfactory. Decomposition of 15 was observed when it was reacted with LiEt3BH (entry 11).
Alkyl iodide 16 was first subjected to catalytic hydrogenolysis.17 Only a 38% conversion to product was observed through 1H NMR analysis of crude reaction mixtures (entry 12). Heating 16 in the presence of
NaBH4 in DMSO resulted in the decomposition of starting material (entry 13). However, treatment with 3.0 equivalents of LiEt3BH at 0 oC for 2 h afforded 17 in up to 91% yield, together with 5-12% of sulfinamide 20.18 This reaction was generally very clean and was easily scaled up to gram-scale.
A possible mechanism for the formation of byproducts 18 and 19 is shown in Scheme 3. Based on proposed mechanisms for the LAH reduction of sulfones and sulfoxides,19 we suggest that reduction might occur through initial S-N bond cleavage to afford 22. Tosylate reduction followed by sulfoxide reduction would afford 18. If displacement of the leaving group at the nascent C-11 methyl group has not yet occurred, cyclization is possible and subsequent sulfoxide reduction would give 19. The exact timing of tosylate reduction is open to question, but formation of an anilide must presumably precede reduction during the formation of 19.
If reagents and reaction conditions could be manipulated to selectively produce 18 or 19, a powerful methodology for the synthesis of such compounds would be available.
CONCLUSION
We have developed a reliable 6-step sequence to benzothiazine 17, a key intermediate in our projected total synthesis of seco-pseudopteroxazole (2), featuring our completely stereoselective benzothiazine chemistry. Enolate protonation provided high levels of diastereoselectivity in setting the C-11 stereochemistry of 2. The total synthesis of seco-pseudopteroxazole is still in progress and the results will be reported in due course.
EXPERIMENTAL
General. All reactions were carried out under an atmosphere of nitrogen or argon in flamed-dried glassware. All commercial grade reagents and solvents were used as supplied with the following exceptions. Ethyl ether (Et2O), tetrahydrofuran (THF) and toluene were freshly distilled from sodium-benzophenone before use. Triethylamine, dichloromethane and acetonitrile were distilled from calcium hydride. Chromatographic separations were carried out using Silicycle ultra pure silica gel (230-400 mesh). Analytical thin layer chromatography was performed on EM reagent 0.25 nm silica gel 60-F plates with F-254 indicator. Melting points were measured with a Fisher-Johns melting point apparatus. Infrared spectra were recorded on a Thermo Nicolet NEXUS 670 FT-IR spectrometer. Optical rotations were measured on a Jasco DIP-370 digital polarimeter with a sodium lamp and are reported as follows: [α]25D (c g/100 mL, solvent). High-resolution mass was measured on a Bruker 12 Tesla FRICR-MS with an Apollo II ion source in Old Dominion University. Elemental analysis was performed by the MHW laboratories, Phoenix, AZ. 1H and 13C-NMR spectra were recorded on a Bruker ARX-250 (250 MHz), DRX-300 (300 MHz), DRX-500 (500 MHz) spectrometer. Chemical shifts were reported in ppm using tetramethylsilane (TMS: δ 0.0 ppm) as an internal standard. Data are reported as follows: chemical shift, multiplicity (s = singlet, d = doublet, t = triplet, q = quartet, m = multiplet), coupling constants (Hz) and integration.
Preparation of (E)-methyl 2-(2-bromo-3-methoxy-5-methylbenzylidene)-6-methylhept-5-enoate 7
To a stirred suspension of LiCl (2.21g, 52.3 mmol) in dry MeCN (170 mL) under N2 atmosphere at rt, were sequentially added phosphonate 6 (13.8 g, 52.3 mmol), DBU (6.5 mL, 43.6 mmol) and aldehyde 5 (10 g, 43.6 mmol). The resulting mixture was stirred for overnight (12 h) before it was diluted with EtO2C. The organic solution was washed with water and brine, then dried over MgSO4. Concentration of the solvent in vacuo afforded the crude product. NMR analysis of crude reaction mixture showed the ratio of E: Z isomer is 10: 1.0. Flash column chromatography (5% EtOAc/hexanes) gave 13.3 g (83%) of E-7 and 1.6 g (10%) of an E/Z mixture. E-7: Yellow liquid; IR: ν 2945, 2851, 1715, 1572, 1425, 1323, 1258, 1090, 1029, 833 cm-1; 1H-NMR (250 MHz, CDCl3): δ 7.60 (s, 1H), 6.68 (s, 1H), 6.64 (s, 1H), 5.03 (t, J = 7.5 Hz, 1H), 3.84 (d, J = 1.9 Hz, 3H), 3.79 (d, J = 1.6 Hz, 3H), 2.32-2.39 (m, 2H), 2.29 (s, 3H), 2.10-2.16 (m, 2H), 1.61 (s, 3H), 1.51 (s, 3H); 13C-NMR (62.5 MHz, CDCl3): δ 168.1, 155.7, 139.0, 137.7, 137.5, 134.0, 132.1, 123.4, 122.4, 112.0, 109.6, 56.1, 51.8, 27.7, 27.4, 25.5, 21.3, 17.4; HRMS caclcd for C18H23BrO3Na+ [M+ Na]+ 389.0722, found 389.0716.
Procedure for Buchwald-Hartwig coupling reaction
To a 500 mL round bottomed flask equipped with a reflux condenser under N2 atmosphere were sequentially added bromoester 7 (10 g, 27.2 mmol), R-sulfoximine 8 (5.0 g, 32.6 mmol), Pd(OAc)2 (312 mg, 1.36 mmol), rac-BINAP (1.31 g, 2.04 mmol), Cs2CO3 (12.4 g, 38.0 mmol) and then toluene (270 mL). The reaction mixture was heated in an oil bath at 110-115 oC for 50 hr. The solution was cooled to rt and diluted with CH2Cl2. The organic solution was filtered through a pad of Celite. Concentration of solvent in vacuo afforded the crude product as brown oil. Purification of the product was carried out by flash chromatography (30% EtOAc/hexane) afforded 9 as a pale yellow semi-solid 12.0 g (99%). IR: ν 2929, 1703, 1462, 1335, 1258, 1204, 1160, 1090, 739 cm-1; 1H-NMR (250 MHz, CDCl3): δ 8.06 (s, 1H), 8.00-8.04 (m, 2H), 7.47-7.57 (m, 3H), 6.77 (s, 1H), 6.60 (d, J = 1.3 Hz, 1H), 5.17 (tt, J = 7.3, 1.3 Hz, 1H), 3.82 (s, 3H), 3.58 (s, 3H), 3.12 (s, 3H), 2.46-2.52 (m, 2H), 2.28 (s, 3H), 2.22-2.25 (m, 2H), 1.68 (s, 3H), 1.62 (s, 3H); 13C-NMR (62.5 MHz, CDCl3): δ 169.0, 151.9, 142.6, 139.1, 132.3, 131.9, 131.8, 131.5, 130.0, 128.8, 127.4, 123.8, 122.0, 112.9, 55.4, 51.6, 46.0, 28.0, 27.8, 25.6, 21.2, 17.6; HRMS caclcd for C25H31NO4SNa+ [M+ Na]+ 464.1849, found 464.1849; [α]25D +15.66 (c 1.66, acetone).
Procedure for the intramolecular Michael reaction
To a 1 L round bottom flask under N2 atmosphere were added 9 (9.6 g, 21.7 mmol) and THF (430 mL).
The mixture was cooled to –78 oC before it was added dropwise LiHMDS (1.0 M in THF, 43 mL, 43.4 mmol). The stirring was continued for 1 h. The mixture was vigorously stirring with the rapid addition of a cold 1N HCl in MeOH solution, which was precooled to -78 oC. The resulting mixture was warmed to rt and diluted with EtOAc and H2O. The organic layer was separated and the aqueous layer was extracted with EtOAc. The combined organic layer was washed with brine and dried over MgSO4. Concentration of solvent in vacuo afforded crude product (d.r. 9.2: 1.0). Purification was carried out using column chromatography (30% EtOAc/hexane) to afford 9.5 g of 10 (> 99%). 10: Yellow foam; IR: ν 2929, 1732, 1458, 1254, 1159, 1012, 747 cm-1; major isomer: 1H-NMR (250 MHz, CDCl3): δ 8.11 (d, J = 7.8 Hz, 2H), 7.50-7.65 (m, 3H), 6.68 (s, 1H), 6.60 (s, 1H), 4.96 (t, J = 7.0 Hz, 1H), 3.86 (s, 3H), 3.52-3.58 (m, 2H), 3.37 (s, 3H), 3.29-3.37 (m, 1H), 3.11-3.20 (m, 1H), 2.30 (s, 3H), 1.85-1.91 (m, 2H), 1.65 (s, 3H), 1.56-1.62 (m, 1H), 1.52 (s, 3H), 1.45-1.52 (m, 1H); 13C-NMR (62.5 MHz, CDCl3): δ 174.9, 152.7, 139.5, 133.3, 132.6, 131.4, 130.2, 129.1, 129.0, 125.3, 122.7, 120.1, 111.9, 55.9, 52.4, 51.2, 44.3, 38.5, 31.4, 25.6, 21.3, 17.5; HRMS caclcd for C25H31NO4SNa+ [M+ Na]+ 464.1866, found 464.1853.
Procedure for LiEt3BH reduction of ester 10
In a 300 mL flask were placed 10 (3.6 g, 8.15 mmol) and THF (160 mL). The flask was cooled to 0 oC with ice cooling. LiEt3BH (1.0 M in THF, 24.4 mL, 24.4 mmol) was added dropwise in 30 min with vigorous stirring. The stirring was continued for an additional 30 min. The reaction was quenched by sequential addition of MeOH, 1 N NaOH and 30% H2O2. The resulting mixture was extracted with CH2Cl2. The combined organic extract was washed with H2O and brine. The organic extract was dried over MgSO4. Concentration of solvent in vacuo afforded 12 (3.4 g, >99% yield), which was pure enough to carry out next step without further purification. 12: Yellow semi-solid; IR: ν 3440, 2925, 1470, 1249 cm-1; major isomer: 1H-NMR (250 MHz, CDCl3): δ 8.01 (d, J =7.2 Hz, 2H), 7.43-7.59 (m, 3H), 6.64 (s, 1H), 6.59 (s, 1H), 5.03 (t, J = 7.1 Hz, 1H), 3.82 (s, 3H), 3.48-3.54 (m, 1H), 3.30-3.43 (m, 3H), 3.15-3.21 (m, 1H), 2.28 (s, 3H), 2.24-2.33 (m, 2H), 1.94-2.03 (m, 2H), 1.66 (s, 3H), 1.54 (s, 3H), 1.24-1.36 (m, 2H); 13C-NMR (62.5 MHz, CDCl3): δ 152.3, 139.1, 133.4, 132.1, 131.9, 129.6, 129.0, 129.0, 126.1, 123.8, 118.9, 111.1, 61.4, 55.8, 49.9, 38.4, 29.3, 25.6, 21.4, 17.6; HRMS caclcd for C24H32NO3S+ M+ 414.2097, found 414.2097.
Procedure for the preparation of sulfonate ester 13, 14 and 15
To the dichloromethane solution (17 mL) of alcohol 12 (360 mg, 0.87 mmol) were successively added sulfonyl chloride (1.30 mmol), DMAP (10.6 mg, 0.09 mmol) and TEA (365 μL, 2.60 mmol). The resulting mixture was stirred at rt for a few hours before it was diluted with water. The organic layer was washed with brine and dried over MgSO4. Flash chromatography (50% EtOAc/hexane) provided the
desired sulfonate ester.
13: Yellow oil, 87% yield; IR: ν 2929, 1462, 1254, 1168 cm-1; major isomer: 1H-NMR (250 MHz, CDCl3): δ 8.11 (d, J = 8.0 Hz, 2H), 7.53-7.69 (m, 3H), 6.70 (s, 1H), 6.59 (s, 1H), 5.04 (t, J = 6.5 Hz, 1H), 4.17-4.21 (m, 1H), 3.85-3.93 (m, 1H), 3.88 (s, 3H), 3.42-3.46 (m, 2H), 3.14 (dd, J = 13.7, 9.5 Hz, 1H), 2.87 (s, 3H), 2.65-2.67 (m, 1H), 2.33 (s, 3H), 2.03-2.10 (2H), 1.70 (s, 3H), 1.44-1.70 (m, 2H), 1.59 (s, 3H); 13C-NMR (62.5 MHz, CDCl3): δ 152.7, 138.8, 133.8, 132.8, 132.0, 130.1, 129.3, 129.1, 124.7, 122.8, 118.7, 111.5, 68.7, 55.9, 49.4, 37.0, 36.4, 36.1, 29.1, 25.6, 25.2, 21.4, 17.7; HRMS caclcd for C25H33NO5S2Na+ [M+Na]+ 514.1692, found 514.1695.
14: Yellow solid, 95% yield, mp 103-4 oC; IR: ν 2929, 1462, 1331, 1254, 1151 cm-1; major isomer: 1H-NMR (300 MHz, CDCl3): δ 8.08-8.11 (m, 2H), 7.54-7.66 (m, 3H), 6.70 (d, J = 1.2 Hz, 1H), 6.59 (s, 1H), 5.04 (td, J = 7.0, 1.5 Hz, 1H), 4.14-4.19 (m, 1H), 3.89-3.92 (m, 1H), 3.89 (s, 3H), 3.41-3.47 (m, 2H), 3.12-3.21 (m, 2H), 2.60-2.70 (m, 1H), 2.33 (s, 3H), 2.02-2.15 (m, 2H), 13C-NMR (75 MHz, CDCl3): δ 152.7, 138.9, 133.8, 132.8, 132.0, 130.1, 129.3, 129.0, 124.9, 122.9, 118.8, 111.5, 67.7, 56.0, 52.0, 49.5, 36.4, 36.2, 29.3, 25.7, 25.3, 21.4, 17.7, 16.5, 16.4; HRMS caclcd for C27H37NO5S2Na+ [M+Na]+ 542.2005, found 524.2004.
15: Yellow foam, 87% yield. IR: ν 2913, 1450, 1168, 1086 cm-1; major isomer: 1H-NMR (250 MHz, CDCl3): δ 8.02 (d, J = 7.4 Hz, 2H), 7.48-7.65 (m, 5H), 7.24 (d, J = 8.1 Hz, 2H), 6.65 (s, 1H), 6.46 (s, 1H), 4.91 (t, J = 6.7 Hz, 1H), 3.90 (dd, J = 10.3, 3.0 Hz, 1H), 3.85 (s, 3H), 3.63 (dd, J = 10.0, 4.3 Hz, 1H), 3.28-3.38 (m, 2H), 2.99-3.09 (m, 1H), 2.42-2.50 (m, 1H), 2.39 (s, 3H), 2.26 (s, 3H), 1.83-1.89 (m, 2H), 1.64 (s, 3H), 1.47 (s, 3H), 1.22-1.55 (m, 2H); 13C-NMR (62.5 MHz, CDCl3): δ 152.6, 144.9, 138.9, 133.7, 132.5, 132.2, 131.8, 130.1, 129.8, 129.2, 129.0, 127.6, 124.9, 122.9, 119.0, 111.5, 69.0, 55.9, 49.8, 36.5, 36.0, 28.9, 25.6, 25.1, 21.5, 21.3, 17.6.
Procedure for the preparation of iodide 16
To a stirred, cooled (0 oC) MeCN (30 mL) and Et2O (30 mL) mixture were successively added 12 (3.30 g, 8.15 mmol), triphenylphosphine (4.27 g, 16.3 mmol) and imidazole (1.11 g, 16.3 mmol). Iodine (4.10 g, 16.3 mmol) was slowly added. After the resulting pale yellow suspension was stirred for 5 hr (monitored by TLC), the reaction mixture was diluted with ether and sequentially washed with saturated aqueous Na2S2O3, saturated aqueous CuSO4 and water. The organic layer was dried over MgSO4 and concentrated in vacuo to afford crude iodide. Flash chromatography (30% EtOAc/hexanes) gave 3.79 g of desired iodide 16 (89% over the two steps). Yellow seimi-solid; IR: ν 2921, 1462, 1254, 1151, 1017, 747 cm-1; major isomer: 1H-NMR (250 MHz, CDCl3): δ 8.12 (d, J = 7.9 Hz, 2H), 7.51-7.67 (m, 3H), 6.70 (s, 1H), 6.55 (s, 1H), 4.97 (t, J = 7.0 Hz, 1H), 3.88 (s, 3H), 3.42 (d, J = 5.3 Hz, 2H), 3.09-3.14 (m, 2H), 2.51 (dd, J = 10.4, 5.7 Hz, 1H), 2.33 (s, 3H), 1.80-1.96 (m, 3H), 1.68 (s, 3H), 1.55 (s, 3H), 1.23-1.52 (m, 2H); 13C-NMR (62.5 MHz, CDCl3): δ 152.9, 139.4, 133.7, 132.4, 131.6, 130.5, 129.3, 129.0, 125.7, 123.0, 120.3, 111.7, 56.0, 50.7, 40.6, 36.5, 31.8, 25.7, 25.1, 21.3, 17.7, 11.1; HRMS caclcd for C24H31INO2S+ M+ 524.1114, found 524.1109.
Procedure for the preparation of 17
In a 300 mL flask were placed iodide 16 (4.62 g, 8.82 mmol) and THF (175 mL). The flask was immersed into an ice bath (0 oC). LiEt3BH (1.0 M in THF, 26.3 mL, 26.3 mmol) was added dropwise in 50 mins using syringe pump (0.49 mL/min) with vigorous stirring. After addition was complete, stirring was continued for an additional 1 hr. The reaction was quenched by sequential addition of MeOH, 1 N NaOH and 30% H2O2. The reaction mixture was extracted with CH2Cl2. The combined organic layers were washed with H2O and brine. The combined organic layer was dried over MgSO4. Concentration of solvent in vacuo afforded crude 17. Flash chromatography (30% EtOAc/Hexane) afforded 3.19 g of 17 (91% yield) and 260 mg of 20 (ca. 10% yield). 17: Yellow semi-solid; IR: ν 2913, 1458, 1241, 1012 cm-1; major isomer: 1H-NMR (250 MHz, CDCl3): δ 8.08-8.12 (m, 2H), 7.52-7.67 (m, 3H), 6.68 (s, 2H), 5.12 (tt, J = 7.1, 1.3 Hz, 1H), 3.89 (s, 3H), 3.30-3.44 (m, 2H), 2.85 (t, J = 12.0 Hz, 1H), 2.49-2.56 (m, 1H), 2.34 (s, 3H), 2.03-2.09 (m, 2H), 1.71 (s, 3H), 1.62 (s, 3H), 1.27-1.51 (m, 2H), 0.80 (d, J = 6.7 Hz, 3H); 13C-NMR (62.5 MHz, CDCl3): δ 152.2, 139.2, 133.5, 132.3, 131.9, 129.4, 129.1, 125.7, 123.8, 118.3, 110.9, 55.9, 47.9, 40.2, 38.0, 35.2, 31.4, 25.8, 25.6, 21.4, 17.6, 14.7; HRMS caclcd for C24H31NO2SNa+ [M+Na]+ 420.1967, found 420.1958.
Byproduct 18: Red oil; d.r.: 3.3: 1.0; IR: ν 3444, 3366, 2913, 1585, 1486, 1290, 1160, 735 cm-1; major isomer: 1H-NMR (500 MHz, CDCl3): δ 7.25-7.32 (m, 4H), 7.16 (t, J = 7.0 Hz, 1H), 6.53 (s, 2H), 5.02 (t, J = 6.8 Hz, 1H), 3.86 (s, 3H), 3.64 (s, 2H, NH2), 3.43 (dd, J = 12.2, 4.2 Hz, 1H), 3.20 (dd, J = 12.5, 10.5 Hz, 1H), 2.89 (ddd, J = 10.6, 6.8, 3.8 Hz, 1H), 2.29 (s, 3H), 1.85-2.0 (m, 5H), 1.68 (s, 3H), 1.56 (s, 3H), 1.38-1.40 (m, 1H), 1.13-1.17 (m, 1H), 1.03 (d, J = 6.5 Hz, 3H); 13C-NMR (75 MHz, CDCl3): δ 147.4, 137.4, 132.0, 131.4, 128.8, 128.7, 127.9, 127.0, 125.5, 124.5, 119.6, 109.0, 55.3, 43.5, 36.5, 36.4, 34.3, 25.6, 25.4, 21.3, 17.5, 16.8; HRMS caclcd for C24H33NOSNa+ [M+Na]+ 406.2175, found 406.2165.
The presence of NH2 group was clearly shown in IR spectrum (doublet: 3444, 3366 cm–1). A peak of doublet at 1.03 ppm with coupling constant of 6.5 Hz indicated that the tosylate group was cleaved. Furthermore, a careful comparison of proton NMR of desired product 17, a lower chemical shift of S-phenyl group (7.15-7.32 ppm in 18, as compared 7.52-8.12 ppm in 17) suggested the more shielding from sulfur atom, and thus low valence of sulfur in the molecule, which was later confirmed to be thiophenyl ether by the high resolution mass spectrum. Thus, the structure of 18 was assigned.
Byproduct 19: Red liquid; single diastereomer; IR: 3423, 2908, 1585, 1507, 1262, 1102, 833, 735, 690 cm-1; 1H-NMR (500 MHz, CDCl3): δ 7.13-7.37 (m, 5H), 6.55 (s, 1H), 6.49 (d, J = 1.4 Hz, 1H), 5.10 (td, J = 7.0, 1.3 Hz, 1H), 4.17 (s, 1H), 3.81 (s, 3H), 3.36 (ddd, J = 11.5, 4.2, 1.2 Hz, 1H), 3.22 (dd, J = 11.8, 10.3 Hz, 1H), 3.21 (dd, J = 12.0, 4.7 Hz, 1H), 3.05-3.09 (m, 1H), 3.02-3.05 (m, 1H), 2.25 (s, 3H), 2.02-2.10 (m, 3H), 1.67 (s, 3H), 1.60 (s, 3H), 1.25-1.55 (m, 2H); 13C-NMR (75 MHz, CDCl3): δ 145.9, 137.3, 131.8, 130.6, 129.0, 128.7, 125.6, 124.2, 124.0, 123.2, 121.6, 109.1, 55.3, 42.9, 39.4, 35.3, 34.9, 29.5, 25.7, 25.6, 20.9, 17.6; HRMS caclcd for C24H31NOSNa+ [M+Na]+ 404.2018, found 404.2014.
The structure of minor byproduct 19 was assigned based on the extensive NMR studies. Coupling constants of H9 and H10 were calculated based on 2D J-resolved NMR spectrum. As shown in 1H-NMR, the sulfoximine moiety was reduced as clearly indicated by the lower chemical shift of S-phenyl group. The absence of tosylate group and no distinct doublet around 1.00 ppm (methyl group), together with the evidence of free NH group, suggested the presence of tetrahydroquinoline moiety in the molecule. The attempts to calculate the coupling constants of H7 based on the 1H-NMR turned out to be fruitless, because H7 overlapped with H10a and the spectrum turned into second-order spectrum (Δδ/J: 1.87). Thus, the stereochemistry of H7 and H8 were assigned based on the stereochemistry of the starting material. The structural assignment was also supported by NOESY experiment (Figure 4).
ACKNOWLEDGEMENTS
We are grateful to the National Institutes of Health (1R01-AI59000-01A1).
This paper is dedicated to Professor Emeritus Keiichiro Fukumoto on the occasion of his 75th birthday.
References
1. (a) M. Harmata, X. Hong, and C. L. Barnes, Org. Lett., 2004, 6, 2201; CrossRef (b) M. Harmata and X. Hong, Org. Lett., 2005, 7, 3581. CrossRef
2. M. Harmata and X. Hong, J. Am. Chem. Soc., 2003, 125, 5754. CrossRef
3. A. D. Rodriguez, C. Ramirez, I. I. Rodriguez, and E. Gonzalez, Org. Lett., 1999, 1, 527. CrossRef
4. G. Qabaja and G. B. Jones, J. Org. Chem., 2000, 65, 7187. CrossRef
5. (a) W. Biernacki and A. Gdula, Synthesis, 1979, 37; CrossRef (b) Z. Liu, W. Z. Li, L. Peng, Y. Li, and Y. Li, J. Chem. Soc., Perkin Trans. 1, 2000, 4250. CrossRef
6. M. A. Blanchette, W. Choy, J. T. Davis, A. P. Essenfeld, S. Masamune, W. R. Roush, and T. Sakai, Tetrahedron Lett., 1984, 25, 2183. CrossRef
7. (a) C. Bolm and J. P. Hildebrand, Tetrahedron Lett., 1998, 39, 5731; CrossRef (b) C. Bolm and J. P. Hildebrand, J. Org. Chem., 2000, 65, 169; CrossRef (c) M. Harmata and N. Pavri, Angew. Chem. Int. Ed., 1999, 38, 2419. CrossRef
8. R. Chow, P. J. Kocienski, A. Kuhl, J.-Y. LeBrazidec, K. Muir, and P. Fish, J. Chem. Soc., Perkin Trans. 1, 2001, 2344. CrossRef
9. V. Gevorgyan, M. Rubin, J. X. Liu, and Y. Yamamoto, J. Org. Chem., 2001, 66, 1672. CrossRef
10. S. Krishnamurthy and H. C. Brown, J. Org. Chem., 1976, 41, 3064. CrossRef
11. J. A. Marshall and D. G. Cleary, J. Org. Chem., 1986, 51, 858. CrossRef
12. S. Masamune, P. A. Rossy, and G. S. Bates, J. Am. Chem. Soc., 1973, 95, 6452. CrossRef
13. S. Thomas, T. Huynh, V. Enriquez-Rios, and B. Singaram, Org. Lett., 2001, 3, 3915. CrossRef
14. H. M. Bell, C. W. Vanderslice, and A. Spehar, J. Org. Chem., 1969, 34, 3923. CrossRef
15. D. H. Hua, G. Sinai-Zingde, and S. Venkataraman, J. Am. Chem. Soc., 1985, 107, 4088. CrossRef
16. The structures of these side products were assigned on the basis of one and two-dimensional NMR data. See experimental section.
17. A. H. G. Siebum, W. S. Woo, and J. Lugtenburg, Eur. J. Org. Chem., 2003, 4664. CrossRef
18. M. Harmata and P. Zheng, Org. Lett., 2007, 9, 5251. CrossRef
19. (a) T. A. Whitney and D. J. Cram, J. Org. Chem., 1970, 35, 3964; CrossRef (b) F. G. Bordwell and W. H. McKellin, J. Am. Chem. Soc., 1951, 73, 2251 CrossRef