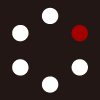
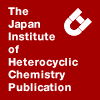
HETEROCYCLES
An International Journal for Reviews and Communications in Heterocyclic ChemistryWeb Edition ISSN: 1881-0942
Published online by The Japan Institute of Heterocyclic Chemistry
e-Journal
Full Text HTML
Received, 20th June, 2008, Accepted, 6th August, 2008, Published online, 11th August, 2008.
DOI: 10.3987/REV-08-637
■ Biologically Active Marine Natural Products
Kazuhiko Nakamura, Makoto Kitamura, and Daisuke Uemura*
Department of Biosciences and Informatics, Faculty of Science and Technology, Keio University, 3-14-1 Hiyoshi, Yokohama 223-8522, Japan
Abstract
The discovery of new biologically active molecules contributes to the development of basic scientific concepts in the field of biological sciences. These compounds often lead to the development of valuable drugs, and to new pharmacological reagents. They also lead to a better understanding of ecological phenomena. Newly discovered substances can even be responsible for the creation of new scientific fields. Due to the radically different habitats of marine organisms, several notable examples of secondary metabolites from marine organisms have been isolated. Two of the most remarkable properties of these compounds are their structural and physiological diversities. Palytoxin is a polyol compound that shows extreme acute toxicity. Halichondrins are remarkable antitumor macrolides from sponge. Pinnatoxins, potent shellfish poisons, cause food poisoning. The compounds controlling the ecological behaviors of coral reveal to us a wonder of nature. And, the compounds produced by symbiotic microorganisms always inspire us by chemical interests. This paper describes bioorganic studies that are helping to develop new research fields.1. INTRODUCTION
Since life on earth first appeared 3.5 billion years ago, organisms have been continuously exposed to harsh environments. According to the central dogma of molecular biology, organisms adapt to their environments by evolving over long periods of time through the transcription of DNA to RNA to protein. And at the same time, the organisms gain control over their vital functions through small molecules that are now called “bioactive substances”. An understanding of the functions of such molecules can directly contribute to the development of basic scientific concepts, and the discovery of novel molecules promotes new biological comprehension. These compounds can also be useful for their effects on humans, thus leading to valuable drug-oriented compounds and possibility to new pharmacological reagents. Progress in these fields often contributes to the betterment of humanity.
Since the last century, much effort has been made to search for biological substances from natural resources. From these efforts, many compounds have been isolated from microorganisms for medical purposes, including antibiotics. The development of this field has been supported by progress in methodologies, including separation techniques and both spectroscopic and X-ray crystallographic analyses. Chemical synthesis methodologies have also achieved notable successes. Natural products chemists have benefited from these developments, enabling them to discover an ever-growing number of new molecular structures.
We have long been attracted to marine natural products because of their complexity.1-8 Their chemical structures are completely different from those of terrestrial products due to the radical differences in their habitats. For example, palytoxin, maitotoxin and okadaic acid fascinate us not only because of their structures but also because of their extraordinary biological activity and interesting ecological phenomena, such as the ecological food chain and symbiosis. The compounds often provide us with the possibility of medicinal resources for the discovery of drug candidates or molecular tools for biological research. Synthetic organic chemists are competing to achieve the total synthesis of such complex natural products, not only as intellectual achievement, but also to provide material for biological evaluation. Pharmacologists have found that some marine natural products are useful tools for clarifying biological molecular mechanisms. Thus marine natural products promise a new field of study. In this paper, we describe our latest work on bioactive marine natural products along with other topics.
2. PALYTOXIN
Palytoxin is a deadly poison that is found in the coelenterate Palythoa tuberculosa. It was first described in Science by Scheuer et al. in 1971.9 Palytoxin was characterized as having a molecular weight of 3300 and a molecular structure with no repetition. In 1980, using plasma desorption mass spectrometry with californium (252Cf), we reported that the toxin’s exact molecular weight was 2680.10 In Japan in 1969, at about the time that palytoxin was found in biota in Hawaii, Hashimoto et al. reported that a species of Alutera scripta in Okinawa contained an extremely strong poison called aluterin.11 When these reports were made, it was not suspected that both of these poisons were from coelenterates. Thus, the question arose as to who should be credited with the discovery. In fact, Banner et al., who studied ciguatera in the 1960s at the University of Hawaii, should be credited with the discovery. Based on local legends, the researchers learned that Limu-make-o-Hana, a deadly poisonous alga, could be found in the Hana District. They wanted to identify the exact origin of this toxin, as it was suspected of having an association with ciguatera. However, they discovered that the poison actually came not from the alga but from a species of Palythoa toxicus.12 The extremely strong toxicity of this species was reported in C&E News.12
Not long after, Scheuer et al. reported the chromophore of palytoxin.14 In 1974, at the time of these reports, we started our work.
Hashimoto had already reported that aluterin extracted from A. scripta actually originated in P. tuberculosa (vide post). Thus, in 1974, we believed that the islands of Okinawa and Ishigaki were the two best places for conducting our research. First, we tried to collect P. tuberculosa on the main island of Okinawa but failed to find any specimens that exhibited potent toxicity. We then collected specimens from Ishigaki Island. At low tide, the coral reef around the edge of the island is exposed above the water, and P. tuberculosa can be easily obtained. Each time of field works, 100 kg of coelenterates was collected, and we froze the living specimens in dry ice and sent them to Nagoya. This process was repeated several times. P. tuberculosa is a very beautiful creature that spreads its polyp to form a colony 1 to 1.5 cm deep. Between April and June, the coelenterates produce eggs and have the strongest toxicity. We used specimens collected during this first season to purify palytoxin. The planar structure of 1 was eventually clarified in 1981,15 and its stereochemical structure was determined in 1982.16 There was a competition between a group at the University of Hawaii and our group to determine the complete structure. The two competing groups proposed different structures for the same 13C-NMR spectrum; our group suggested a hemiacetal structure, while the Hawaii group proposed an anhydro structure. However, no other researchers supported the presence of an anhydro structure. Finally, we clarified the true stereochemical structure of palytoxin by an overall degradation reaction and chemical synthesis in collaboration with Yoshito Kishi at Harvard University.
We next sought to determine the functions responsible for the extremely strong toxicity of palytoxin and the shape of the molecules. The true molecular shapes of such polyol compounds have yet to be clarified. Therefore, we tried to determine what these molecules looked like using the small-angle X-ray scattering method, which is commonly used with proteins and high-molecular-weight substances. Because palytoxin is much smaller than proteins, we needed to use a strong radiation light with small-angle X-ray scattering. We used the radiation light facility of SPring-8 (RIKEN Harima Institute). First, we obtained the scattering curve for an aqueous solution of palytoxin. Then, we computed a stable conformation using model calculations and determined whether or not the conformation matched the scattering curve. The maximum length was calculated with a radial distribution function and the Guinier approximation. We discovered that palytoxin has a length of 50 Å, while N-acetyl palytoxin, which has an acetyl group at its N-terminus, has a length of 35 Å.17 We also calculated the molecular weight to be 5700 for palytoxin and 2600 for N-acetyl palytoxin. In an aqueous solution, palytoxin was found to exist as a dimer, while N-acetyl palytoxin exists as a monomer. Using a low-resolution model simulation, we found that in an aqueous solution a molecule of N-acetyl palytoxin has a U-shape, while the dimer of palytoxin consists of two U-shaped molecules overlapping each other to form a figure-eight. Even though we currently have a fairly good understanding of the molecule's shape, we feel that an even more detailed analysis is required.
Low resolution models of palytoxin (left) and N-acetylpalytoxin (right) in solution based on dummy-residues modeling from X-ray small-angle scattering (SAXS) profiles.
An early study determined that palytoxin targets Na+, K+-ATPase. Na+, K+-ATPase is essential in neural transmission, as it draws out three Na+ ions from within a nerve cell and exchanges them with two K+ ions from outside the cell. We discovered that palytoxin creates a channel-like structure within Na+, K+-ATPase.18,19 A channel is made within the ATPase, into which Na+ ions enter from the outside. This activity is antagonized by ouabain. Recent research has determined that palytoxin and ouabain bind to different positions. The Na+ channel that is created by palytoxin has a larger diameter than the usual Na+ channels. Thus, palytoxin has become well known as a physiological reagent that fixates an ion channel in the common ATPase.
3. HALICHONDRIN B
Halichondrins were isolated from the marine sponge Halichondria okadai Kadota.20,21 The invertebrate animal is widely distributed on the rocky Pacific shores of Japan. Generally, marine sponges are ecologically interesting because they frequently harbor epibionts and/or symbionts such as bacteria and blue-green algae. Since sponges may be thought of as equivalent to colonies of marine microorganisms, they are valuable sources of extremely minor bioactive constituents among very large amounts of other compounds. We found that crude extracts of H. okadai significantly inhibited cancer cell growth in vitro. All chromatography proceeded by a reversed-phase column to obtain eight compounds, named halichondrins. The component that we named halichondrin B was the most active compound, so we selected this compound for in vivo tests against a variety of tumor cell lines. Halichondrin B exhibited significant effects at a concentrations as low as 5.0 µg/kg, and showed a high life-prolongation effect of more than 244% with low toxicity. In addition, administration was effective not only intraperitoneally but also intravenously.
Halichonrin B’s structure was successfully revealed mainly by X-ray analysis and detailed spectroscopic analysis such as NMR spectral data by our group,20 in competition with Professor Munro of the University of Canterbury, Professor Pettit of Arizona State University, and others.22 Halichondrin B consists of a very long carbon chain that could be depicted as a single long brush stroke from the carbon at the end of the molecule; this is similar to the structure of palytoxin. Kishi et al. also synthesized this molecule23 and determined that the right half of the molecule is the pharmacophore of its potent antitumor activity.24,25 A derivative of the pharmacophore (E7389 or named “eribulin masylate”) was selected for a drug candidate. Today, the Eisai Research Institute of Boston is aggressively developing an effective synthesis. The right half of the molecule is effective against cancers, especially breast cancer, which is resistant to taxol. One advantage of the synthesis of the right half of the molecule is that 2 kg of pure synthesized substance can be supplied annually in 40-50 steps. Once this compound finds its way into actual clinical use as an antitumor medicine, we believe it will greatly encourage the work of many organic synthetic chemists.
The mode of anticancer action was also elucidated through the pharmacological studies of E7389.26 Like its mother compound halichondrin B, E7389 is classified into a tubulin depolymerizer. It acts to disrupt the polymerization of the microtubules necessary to mitosis. This profile is also characterized in some other naturally occurring anticancer drugs, such as Vinca alkaloids, dolastatins, cryptophycin, and so on. However, its tubulin interactions appear to be unique, and it was found to have great activity against lung and breast tumors, especially in the case of drug-resistant cancers.
The search for the true natural producer(s) of halichondrins is closely connected with this biogenetic hypothesis. H. okadai itself is unlikely to biosynthesize halichondrins; bacteria or blue-green algae are more probable candidates, as in the case of eicosapentaenoic acid. The quantity of halichondrins isolated depends on the locations of the collected sponges. Halichondrin themselves are only slowly extractable from pulverized animals, so a sufficiently long period is needed to obtain a desired amount of it. This may mean that unicellular microorganisms are not completely broken down. A few species of bacteria have been isolated from animals and cultured in marine agar medium. Unfortunately, however, the true biological origin of halichondrins remains unknown.20
Halichondrin B is classified into super-carbon-chain compounds. From biogenetic standpoint, these compounds are likely to be biosynthesized via the polyketide pathway, and a divergent and latent acetate-propionate route must be existent. If the carbon framework fringed with oxygen atoms and methyl groups can be produced, it might be recognized that the structure of halichondrin B is biogenetically constructed. An attack by water acts as a trigger, and simultaneous removal of coenzyme A results in lactonization. After six- and five-membered ether rings form in this lactone, two types of domino cyclization are independently initiated. The reason why the right half of this precursor contains only trans-epoxide and trans-double bonds, while the left half includes only cis-epoxide, may lead to an interesting biosynthetic story.20
4. PINNATOXINS
We isolated pinnatoxins A-D and determined their structures as Ca2+ channel activators from the bivalve Pinna pectinata.27 There have been reports of shellfish poisoning on the coast of the Ariake Sea in Japan. Furthermore, the Chinese government has forbidden the consumption of the viscera of Pinna attenuata. Both of these shellfish contain a large adductor muscle, which is a popular sushi ingredient in Japan. Because it is difficult to collect a large number of P. pectinata, we extracted pinnatoxin A from Pinna muricata, a shellfish that Okinawans never eat because it is considered poisonous. Pinnatoxin A is a water-soluble, amphoteric compound that contains iminium and carboxylate moieties. At first analysis, its structure looks like a carbocycle, but it is actually biosynthesized from a 6,7-membered spirocycle through the formation of a Schiff base followed by a Diels-Alder reaction, as seen in the formation of a cyclohexene ring. Based on this biosynthesis, Kishi et al. synthesized the enantiomers of pinnatoxin A, as well as pinnatoxin itself.28 Recently, Hirama's group succeeded in the formal net synthesis of pinnatoxin,29 and the total synthesis also reported.30 When we examined the particular Diels-Alder reaction associated with this substance, even on mass spectrum fragmentation, one can observe a retro Diels-Alder reaction with charge remote fragmentation. Of all the pinnatoxins, the most toxic are pinnatoxins B and C, each of which has an amino acid structure with two epimers at the α-position.32 These epimers, two pinnatoxins, cannot be separated from each other, and we reported the natural compounds as a 1 : 1 mixture. Recently, however, Kishi et al. succeeded in a complete synthesis of these two enantiomers.32
Once a methodology is established, a researcher can use it to determine the structure of a substance, even if only a very small quantity of the substance is available. We used this approach to study the Okinawan bivalve Pteria penguin.33 Although this shellfish has a well-developed adductor muscle, it is never eaten by Okinawans. In fact, the Okinawans throw the viscera of this shellfish to the moray eel, which also refuses to consume it. This suggests that the shellfish is highly toxic. We discovered that pteriatoxins A, B, and C cause the toxicity in this shellfish. With only 8 µg of pteriatoxins B and C, we established their structures on a nanomole scale. These compounds are thought to be biosynthesized in a way similar to an epoxide, followed by nucleophilic addition of the cysteine moiety. Recently, all eight possible stereoisomers were synthesized, and the stereochemistries of these compounds have been established.34
5. CONTROLLING COMPOUNDS OF CORAL LARVAL METAMORPHOSIS
Crustose coralline red algae (CCA) are plants that deposit a particularly hard and geologically resistant form of calcium carbonate. These algae cement together large quantities of sand, dead coral, and debris to create a stable substrate. CCA play an important role in reef-building processes.35 In addition, their metabolites exhibit interesting biological activities in marine ecological phenomena. For example, some kind of CCA induced the preferential settlement and metamorphosis of abalone larvae.36 Nonpolar substances of another CCA showed growth-inhibitory activity against the dinoflagellates. We are interested in such phenomena induced by bioactive substances from marine organisms; we focused on the relationship between CCA and larvae of invertebrates mediated by their metabolites.
Through our careful observation of marine ecological systems reconstructed in an experimental aquarium, the metamorphosis of coral larvae was induced by neighboring CCA, but they did not metamorphose directly on the CCA surface. This suggested that the relationship between CCA and coral larvae was controlled by the action of settlement-repellent and metamorphosis-inducing substances.
Juvenile polyps of P. tayamai on a fragment of coral rubble with CCA. Coral larvae settled and metamorphosed on the coral rubble, but not on the surface of CCA. Mesenteries, central mouth, and six tentacles were observed with each polyp (arrow). Scale bar = 1 mm.
The settlement-repellent substance of CCA was identified by chromatographic separation guided by toxic activity against the coral larvae (Pseudosiderastrea tayamai). Final HPLC separation of the methanol extract afforded two new brominated aromatic compounds, corallinafuran and corallinaether. These compounds exhibited toxic activity against P. tayamai, with LD50 values of 1.9 and 0.14 µg/ml, respectively.37
However, searching for metamorphosis-inducing substances was more difficult. Finally, we constructed a bioassay system by culturing coral larvae in a sample-coated glass dish. The activity was judged by visual observation (counting under a microscope) of the major change from planula larva to permanently attached and developing primary polyp. For these experiments, we defined metamorphosis to have occurred when the larvae have changed into disk-shaped structures with septal mesenteries radiating from the central mouth region. The chromatographic separation of the methanolic extract of CCA was carried out by guiding this biological assay.
The active fraction was successively purified to give 11-deoxyfistularin-3. The structure of the compound was determined spectroscopically and confirmed by comparison with published data. The compound induced a significantly higher level of larval metamorphosis than in the negative control at concentrations of 10-8 and 10-7 M, but the highest percentage of metamorphosis (37.5 ± 21.4%) was not a satisfactory result compared to the crude extract. These results suggest that two or more compounds present in the fractions have a synergistic effect in the metamorphosis of P. tayamai larvae. This effect guided by isolation chromatographies gave two known carotenoids, fucoxanthin and fucoxanthinol. In the presence of 11-deoxyfistularin-3 (10-7 M), the concentrations of either 10-8–10-11 M for fucoxanthinol or of 10-8–10-9 M for fucoxanthin induced a significantly higher level of larval metamorphosis than 11-deoxyfistularin-3 alone. These synergistic effects indicate that larval metamorphosis, one of the most important events for a marine invertebrate, might be rigidly regulated synergistically by multiple natural cues. The synergistic effect may provide a higher selectivity of recruitment than a single-component natural inducer for selection of a surface to settle on, which may confer a survival advantage of benthic invertebrates on the marine environment.38
Structures (left) and activities (right) of a natural inducer and enhancers of larval metamorphosis. The activities were represented by adding chemicals to the medium.
It is notable that both allomone and kairomone were isolated from the same coral rubble with CCA. These chemical interactions may lead to a better understanding of the competition involved in the recruitment of the same benthic marine organisms. These results may explain why coral larvae metamorphose by contacting CCA but did not metamorphose directly on the surface of it. Therefore, a delicate balance of several molecular interactions among coral larvae and other benthic marine organisms is established in larval metamorphosis.
6. BIOACTIVE SUBSTANCES FROM A SYMBIOTIC MICROALGAE
As above mentioned, biologically active marine natural products, especially super-carbon-chain compounds, have been suggested to originate mostly from microorganisms, i.e. microalgae, bacteria and fungi, which accumulate in host animals through a symbiotic relationship or the food chain. However, to identify the actual producer of these compounds is difficult because the symbiotic biota or food chain ecology of marine animals is so complex. In addition, these microorganisms are difficult to culture in an artificial environment such as a medium. Once cultured, however, these microorganisms can be regarded as good chemical sources of bioactive compounds.
In our continuous study of marine natural products, we have focused on a common marine invertebrate, the flatworm. This animal is known as a rich source of symbiotic microorganisms, and it can be supposed that it cannot live without them. We isolated a dinoflagellate (Symbiodinium) from the marine acoel flatworm Amphiscolops sp. and successfully cultured it. Further, we examined the chemical constituents of algae, in particular, symbioimine, which was isolated as a water-soluble substance from the alcohol extract. Interestingly, in our pharmacological screening, symbioimine inhibited osteoclastogenesis of the murine monocytic cell line RAW264, which can differentiate into osteoclasts following treatment with the receptor activator of the nuclear factor κB ligand, (RANKL, EC50 = 44 µg/mL), whereas its cell viability was not affected even at 100 µg/mL. Thus, symbioimine is an antiresorptive drug candidate for the prevention and treatment of osteoporosis in postmenopausal women. The culture production of the substance for pharmacological usage could be enabled by isolated microalgae.39
We also recently isolated a super-carbon-chain compound with a molecular weight of 2859 from the same dinoflagellate, and named it symbiodinolide. Notably, it was found that symbiodinolide activated the angiotensin II receptor-mediated Ca2+ channel, and also showed anti-HIV activity. The planar structure of symbiodinolide was successfully elucidated by spectroscopic analysis and chemical degradations including hydrolysis and ethenolysis using the second-generation Grubbs' catalyst with ethane. Symbiodinolide was found to be a structural congener of zooxanthellatoxins by detailed analysis. We have established the relative and absolute stereochemistries of various parts of symbiodinolide, and are currently working to determine its entire stereochemical structure.40
Through these studies, we faced the questions, “Why is the molecule biosynthesized?” or “What is the biological meaning of the molecule?” We believe the biosynthesis of such complex and characteristic molecules must logically have some function for marine animals. These compounds seem to be used to retain symbiotic relationships as chemical communication substances by specific molecular recognition of biopolymers, but the details are not clear.
To clarify the role of symbiodinolide in the symbiotic relationships between microalgae and their host animals, we examined the effects of these compounds on host animals that possess symbiotic dinoflagellates. Notably, symbiodinolide caused immediate rupture of the tissue surface of the flatworm Amphiscolops sp. at 2.5 µM. Meanwhile, dinoflagellates released from the host animals seemed to suffer no significant damage, though they hardly moved. It is largely unknown how much symbiodinolide is accumulated in a flatworm. Still, our preliminary results suggest that symbiodinolide may act as a defense substance, which prevents digestion of the host animal. Further studies on its mode of action are currently underway.
Bioassay using symbiodinolide against host animals (acoel flatworm Amphiscolops sp.). A and C: control, B and D: treatment with 1 (2.5 µM) after 10 min. Panels A and B were taken with an optical microscope, and panels C and D were taken with an inverted microscope. Arrows in panel D indicate dinoflagellates liberated from host animals.
As a related study, we are currently interested in the Papuan jellyfish. It is a beautiful creature that hosts various algae inside and around its legs. It is supposed that the dinoflagellates (zooxanthellae) within the jellyfish produce oxygen to enable the jellyfish to survive under light. This relationship is similar to the symbiosis of coral polyps and microalgae; corals also receive oxygen from symbiotic microalgae. Recently, coral bleaching due to stress-induced expulsion of symbiotic algae has led to serious problems in reef ecosystems. Coral bleaching is suspected to be the result of increasing surface water temperatures that is often attributed to global warming, but the detailed mechanism is still unknown. Bleached coral has difficulty surviving as such; thus, the coral environments of the reef become severely damaged. If the mechanism for controlling the symbiotic relationship were revealed, it could lead to a method for protecting coral environments.
Another reason why microalgae exist in the body of marine animals is that they were consumed as a food. The algae might emit some substances that prevent the host from digesting them. Also, there must be a reason why the microalgae live in the inside of a host marine animal. Of course, the relationships between host and microalgae cannot be explained only with reference to the chemical substances. Many questions must still be answered; these answers should help us better understand a presently unknown, but very interesting, ecological system.
7. CONCLUSION
As described in this review, marine natural products comprise a most attractive part of natural product chemistry by virtue of their physiological activity and ecological importance. These compounds have been examined to clarify the mechanisms underlying their various biological actions and have been investigated as potential anticancer, antimicrobial, or antiviral drugs. They clearly play important roles in marine ecological phenomena. It should be emphasized that the importance of marine research has grown substantially as various new assay systems have appeared. Breakthroughs in scientific understanding often come from unexpected directions and lead to discoveries of general concepts. Nature seems to hold solutions to every human problem, whether theoretical or practical; however, where and how these solutions are revealed cannot be predicted.
References
1. D. Uemura, Chem. Rec., 2006, 6, 235. CrossRef
2. M. Kita and D. Uemura, In Progress in Marine Molecular Biotechnology, Vol. 43: Shellfish Poisons. ed. by W. E. G. Müller and G. Schröder, Springer-Verlag Press: Berlin, Heidelberg, 2006; p. 25.
3. M. Kita and D. Uemura In Topics in Heterocycles, Vol. 6: Bioactive Heterocyclic Alkaloids from Marine Origin. ed. by S. Eguchi, Springer-Verlag Press: Berlin, Heidelberg, 2006; p. 157.
4. M. Kita and D. Uemura, Chem. Lett., 2005, 34, 454. CrossRef
5. M. Kuramoto, H. Arimoto, and D. Uemura, J. Synth. Org. Chem. Jpn., 2003, 61, 1099. CrossRef
6. M. Kuramoto, H. Arimoto, and D. Uemura, Mar. Drugs, 2004, 1, 39. CrossRef
7. M. Kita, E. Sakai, and D. Uemura, J. Synth. Org. Chem. Jpn., 2006, 64, 471. CrossRef
8. J. W. Blunt, B. R. Copp, W.-P. Hu, M. H. G. Munro, P. T. Northcote, and M. Prinsep, Nat. Prod. Rep., 2008, 25, 35. CrossRef
9. P. J. Scheuer and R. E. Moore, Science, 1971, 172, 4951. CrossRef
10. R. D. Macfarlane, D. Uemura, K. Ueda, and Y. Hirata, J. Am. Chem. Soc., 1980, 102, 875. CrossRef
11. Y. Hashimoto and S. Kimura, Toxicon, 1972, 10, 611. CrossRef
12. J. S. Wiles, J. A. Vick, and M. K. Christensen, Toxicon, 1974, 12, 427.. CrossRef
13. R. J. Seltzer, C&E News, 1975, December 16, p. 20..
14. R. E. Moore, R. F. Dietrich, B. Hatton, T. Higa, and P. J. Scheuer, J. Org. Chem. 1975, 40, 540. CrossRef
15. D. Uemura, K. Ueda, Y. Hirata, H. Naoki, and T. Iwashita, Tetrahedron Lett., 1981, 21, 1909; CrossRef D. Uemura, K. Ueda, Y. Hirata, H. Naoki, and T. Iwashita, Tetrahedron Lett., 1981, 21, 2781. CrossRef
16. L. L. Klein, W. W. McWhorter Jr., S. S. Ko, K.-P. Pfaff, Y. Kishi, D. Uemura, and Y. Hirata, J. Am. Chem. Soc., 1982, 104, 7362; CrossRef S. S. Ko, J. M. Finan, M. Yonaga, Y. Kishi, D. Uemura, and Y. Hirata, J. Am. Chem. Soc., 1982, 104, 7364; CrossRef H. Fujioka, W. J. Christ, J. K. Cha, J. Leder, Y. Kishi, D. Uemura, and Y. Hirata, J. Am. Chem. Soc., 1982, 104, 7367; CrossRef J. K. Cha, W. J. Christ, J. M. Finan, H. Fujioka, Y. Kishi, L. L. Klein, S. S. Ko, J. Leder, W. W. McWhorter Jr., K.-P. Pfaff, M. Yonaga, D. Uemura, and Y. Hirata, J. Am. Chem. Soc., 1982, 104, 7369. Hawaii group‘s reports are cited therein. CrossRef
17. T. Inuzuka, T. Fujisawa, H. Arimoto, and D. Uemura, Org. Biomol. Chem., 2007
, 897. CrossRef
18. D. W. Hilgemann, Proc. Natl. Acad. Sci. USA, 2003, 100, 386; CrossRef P. Artigas and D. C. Gadsby, Proc. Natl. Acad. Sci. USA, 2003, 100, 501. CrossRef
19. K. Ito, I. Toyoda, M. Higashiyama, D. Uemura, M. H. Sato, S. H. Yoshimura, T. Ishii, and K. Takeyasu, FEBS Lett., 2003, 543, 108. CrossRef
20. D. Uemura and Y. Hirata, In Studies in Natural Products Chemistry, vol. 5 (Part B), ed. by Atta-ur-Rahman, Elsevier; 1989, p. 377.
21. Y. Hirata and D. Uemura, Pure Appl. Chem., 1986, 58, 701. CrossRef
22. D. Uemura, K. Takahashi, T. Yamamoto, C. Katayama, J. Tanaka, Y. Okumura, and Y. Hirata, J. Am. Chem. Soc., 1985, 107, 4796. CrossRef
23. T. D. Aicher, K. R. Buszek, F. G. Fang, C. J. Forsyth, S. H. Jung, Y. Kishi, M. C. Matelich, P. M. Scola, D. M. Spero, and S. K. Yoon, J. Am. Chem. Soc., 1992, 114, 3162. CrossRef
24. D. P. Stamos, S. S. Chen, and Y. Kishi, J. Org. Chem., 1997, 62, 7552. CrossRef
25. K. Namba, H.-S. Jun, and Y. Kishi, J. Am. Chem. Soc., 2004, 126, 7770. CrossRef
26. R. L. Bai, K. D. Paull, C. L. Herald, L. Malspeis, G. R. Pettit, and E. Hamel, J. Biol. Chem., 1991, 266, 15882.
27. D. Uemura, T. Chou, T. Haino, A. Nagatsu, S. Fukuzawa, S. Z. Zheng, and H. Chen, J. Am. Chem. Soc., 1995, 117, 1155; CrossRef T. Chou, O. Kamo, and D. Uemura, Tetrahedron Lett., 1996, 37, 4023; CrossRef T. Chou, T. Haino, M. Kuramoto, and D. Uemura, Tetrahedron Lett., 1996, 37, 4027. CrossRef
28. J. A. McCauley, K. Nagasawa, P. A. Lander, S. G. Mischke, M. A. Semones, and Y. Kishi, J. Am. Chem. Soc., 1998, 120, 7647. CrossRef
29. S. Sakamoto, H. Sakazaki, K. Hagiwara, K. Kamada, K. Ishii, T. Noda, M. Inoue, and M. Hirama, Angew. Chem. Int. Ed., 2004, 43, 6505. CrossRef
30. C. E. Stivala and A. Zakarian, J. Am. Chem. Soc., 2008, 130, 3774. CrossRef
31. F. Matsuura, J. Hao, R. Reents, and Y. Kishi, Org. Lett., 2006, 8, 3327. CrossRef
32. N. Takada, N. Umemura, K. Suenaga, T. Chou, A. Nagatsu, T. Haino, K. Yamada, and D. Uemura, Tetrahedron Lett., 2001, 42, 3491. CrossRef
33. N. Takada, N. Umemura, K. Suenaga, and D. Uemura, Tetrahedron Lett., 2001, 42, 3495. CrossRef
34. F. Matsuura, R. Peters, M. Anada, S. S. Harried, J. Hao, and Y. Kishi, J. Am. Chem. Soc., 2006, 128, 7463; CrossRef J. Hao, F. Matsuura, Y. Kishi, M. Kita, and D. Uemura, N. Asai, T. Iwashita, J. Am. Chem. Soc., 2006, 128, 7742. CrossRef
35. M. M. Littler and M. S. Doty, J. Ecol., 1975, 117, 63.
36. D. E. Morse, Bull. Mar. Sci., 1985, 37, 697.
37. M. Kitamura, T. Koyama, Y. Nakano, and D. Uemura, Chem. Lett., 2005, 34, 1272. CrossRef
38. M. Kitamura, T. Koyama, Y. Nakano, and D. Uemura, J. Exp. Mar. Biol. Ecol., 2006, 340, 96. CrossRef
39. M. Kita, M. Kondo, T. Koyama, K. Yamada, T. Matsumoto, K.-H. Lee, J.-T. Woo, and D. Uemura, J. Am. Chem. Soc., 2004, 126, 4794. CrossRef
40. M. Kita, N. Ohishi, K. Konishi, M. Kondo, T. Koyama, M. Kitamura, K. Yamada, and D. Uemura, Tetrahedron, 2007, 63, 6241 CrossRef