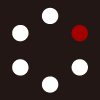
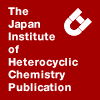
HETEROCYCLES
An International Journal for Reviews and Communications in Heterocyclic ChemistryWeb Edition ISSN: 1881-0942
Published online by The Japan Institute of Heterocyclic Chemistry
e-Journal
Full Text HTML
Received, 23rd August, 2008, Accepted, 22nd September, 2008, Published online, 22nd September, 2008.
DOI: 10.3987/COM-08-S(D)12
■ De Novo Asymmetric Approach To 8a-epi-swainsonine
Jason A. Coral, Haibing Guo, Mingde Shan, and George A. O’Doherty*
Department of Chemistry, West Virginia University, Morgantown, WV 26506, USA
Abstract
An improved method for the synthesis of (-)-8a-epi-swainsonine has been developed with 9 fewer steps than the original route. The synthetic improvements include a two-step procedure for the preparation of benzyl 4-(furan-2-yl)-4-oxobutylcarbamate from pyrrolidin-2-one and a two-step procedure for the preparation of benzyl 3-((3aS,4S,6R,7aR)-4-(benzyloxy)tetrahydro-2,2-dimethyl-7-oxo-3aH-[1,3]dioxolo[4,5-c]pyran-6-yl)- propylcarbamate from benzyl 3-((2R,6S)-6-(benzyloxy)-3,6-dihydro-3-oxo-2H-pyran-2- yl)propylcarbamate.INTRODUCTION
As part of our continuing efforts toward the synthesis of biologically important carbohydrate based natural products, we have been working toward the de novo asymmetric synthesis of various iminosugar based alkaloid natural products. In particular, we have been focused on the preparation of the mannosidase inhibitor, such as (−)-swainsonine (1), 1-deoxy-manno-nojirimycin (2) and other swainsonine analogues (Figure 1). Inspired by the fact that the 8a-epimer of swainsonine, (−)-8a-epi-swainsonine (3), has shown similar activity to (–)-swainsonine as a mannosidase inhibitor, we recently undertook its preparation.c These efforts resulted in a 19-step asymmetric synthesis of (−)-8a-epi-swainsonine (3) from furfural.2c Herein, we report an improved synthesis of (−)-8a-epi-swainsonine (3), which resulted in 3 in only ten steps from achiral pyrrolidin-2-one and furan.
RESULTS AND DISCUSSION
Previously, we had demonstrated that (−)-8a-epi-swainsonine 3 could be prepared by a one-pot reductive hydrogenation of pyranone 5 and acetonide deprotection.2c The hydrogenation occured via a sequence of iterative debenzylation/imine formation/reduction reactions. In our earlier route, pyranone 5 was prepared from 6 in a 4-step reduction/dihydroxylation/protection/oxidation sequence. In this revised approach, we hoped to eliminate two of these steps, (i.e., direct dihydroxylation and acetonide protection). While we were satisfied with our 4-step stereoselective sequence for the formation of 6 from 7, the 9 steps required for the formation of 7 from furfural needed improvement. To this end, we decided to investigate a new approach that could preapre the acylfuran 7 from pyrrolidinone 8 and furan 9 in two steps.
Our revised synthesis of acylfuran 7 began with imide 10, which could be easily prepared from commercially available pyrrolindone 8 and CbzCl.4 All that is required to complete this task is a regioselective carbonyl addition of a furan nucleophile to open the lactam carbonyl in 10. It has been shown that for this substrate 10, carbonyl addition to the carbamate predominated for alkyl lithium nucleophiles.4 Therefore, our investigation started with the search for a suitable 2-furanyl nucleophiles in order to improve upon the poor carbonyl regioselectivity. To this end, 2-furanyllithium, Grignard, copper and cuprate reagents were tested (Table 1). To our delight, we found that all 2-furanyl nucleophiles favored the addition to the lactam carbonyl group, with 2-furanyllithium showing slightly higher selectivity than 2-furanylmagnesium bromide (2:1 vs 1.4:1). The less reactive 2-furanylcuprate reagent gave only similar selectivity as 2-furanyllithium, whereas, the 2-furanyl copper reagent did not react.
Based on the above results, 2-lithiofuran was chosen for the large-scale conversion of imide 10 to acylfuran 7 (Scheme 2). The pyrrolidin-2-one 8 was first acylated with CbzCl to form imide 10 in 70% yield. Addition of 2-furanyllithium to imide 10 at –78 ˚C on 2 g scale resulted in 50% yield of acylfuran 7, together with 21% yield of ester 12, and 11% recovered starting material. This reaction is quite amenable to scale up. For instance, the addition of slight excess 2-lithiofuran does not affect the isolated yield of 7. Presumably the anionic tetrahedral intermediate that leads to 7 does not collapse at −78 °C, thus leaving 12 to consume the remaining 2-furanyllithium.
With a practical synthesis of acylfuran 7 in hand, we introduced the asymmetry into the synthesis by a Noyori reduction of acylfuran 7 (HCO2H/Et3N, 2 mol% Noyori (R,R) catalyst) to form furfuryl acohol 13 in 91% yield and >96% ee (Scheme 3).5 Exposure of the furfuryl alcohol 13 to the Achmatowicz oxidation condition (NBS/THF/H2O) resulted in hemiacetal 14 in 92% yield, which was acylated with (Boc)2O and 15 mol% DMAP at −78 ˚C to afford Boc-protected pyranone 15 with good diastereoselectivity (α/β 7:1). A palladium(0)-catalyzed glycosylation reaction developed in our lab converted the carbonate 15 to benzyl acetal 6 in 88% yield with complete stereocontrol.7
With our improved approach to 6, we next investigated a more direct route to 8a-epi-swainsonine precursor 5.2c To these ends, we explored the dihydroxylation of enone 6 with many dihydroxylation systems (e.g., OsO4, KMnO4, RuO4). We found that a direct dihydroxylation of enone 6 by using a catalytic RuO4 system (NaIO4, 7 mol% RuCl3) afforded the desired diol, which after protection (2,2-DMP/TsOH) resulted in acetonide 5 in 30% yield. The low yield was presumably due to the over-oxidation of the diol intermediate by RuO4 or NaIO4.8
The synthesis was completed by a one-pot hydrogenation (H2, Pd/C; 76%) and acetonide deprotection (HCl/THF; 92%), which provided 8a-epi-swainsonine 3 in 70% yield for the two steps (Scheme 4). A possible mechanistic pathway for this multistep conversion is outlined in Scheme 4. This revised synthesis of 8a-epi-swainsonine 3 provided the target molecule in 10 steps from 2-pyrrolidone and 3.8% overall yield, which to date constitutes the shortest and highest yielding synthesis of this biologically important iminosugar.
EXPERIMENTAL9
General Methods and Materials. 1H and 13C NMR spectra were recorded on 200 MHz, 270 MHz, 300 MHz and 500 MHz spectrometers. Chemical shifts were reported relative to internal tetramethylsilane (δ 0.00) or CDCl3 (δ 7.26) for 1H NMR and CDCl3 (δ 77.0) for 13C NMR. Infrared (IR) spectra were obtained on FT-IR spectrometer. Optical rotations were measured with a digital polarimeter in the solvent specified. Flash column chromatography was performed on 60-200 mesh silica gel. Analytical thin-layer chromatography was performed with precoated glass-backed plates (60 Å, F254) and visualized by quenching of fluorescence and by charring after treatment with p-anisaldehyde or phosphomolybdic acid or potassium permanganate stain. Rf values are obtained by elution in the stated solvent ratios (v/v). Ether (Et2O), THF, methylene chloride (CH2Cl2) and triethylamine (Et3N) were dried by passing through activated alumina columns with argon gas pressure. Commercial reagents were used without purification unless otherwise noted. Air- and/or moisture-sensitive reactions were carried out under an atmosphere of argon/nitrogen using oven-dried glassware and standard syringe/septa techniques.
Carbonyl addition of imide 10 with 2-furanyllithium:
Into a solution of imide 10 (111 mg, 0.51 mmol) in THF (1.5 mL) was added solution of 2-furanyllithium in THF (0.5 mL, 0.7 M, 0.35 mmol) via syringe at −78 ˚C over 5 min. The mixture was stirred at −78 ˚C for another 10 min. Then into this mixture acetone (1.0 mL) was added at −78 ˚C, followed by addition of EtOAc (30 mL). At 0 ˚C, saturated aqueous NH4Cl solution (15 mL) was added to wash the organic layer. The aqueous layer was extracted with EtOAc (2 x 30 mL). The pooled organic layer was washed with saturated brine (15 mL), dried over Na2SO4, and concentrated under reduced pressure to give a residue. Crude 1H NMR showed that the ratio of remaining starting material / acylfuran 7 / ester 12 to be 1.1 : 1.5 : 1.0.10
Carbonyl addition of imide 10 with 2-furanylmagnesium bromide:
Into a flask with MgBr2·Et2O (382 mg, 1.48 mmol) suspended in THF (1.5 mL) was added 2-furanyllithium (2.5 mL of 0.5 M) in THF at −78 ˚C. The mixture was stirred at RT for 30 min., resulting in a clear solution. Into a solution of imide 10 (95 mg, 0.43 mmol) in 2.0 mL THF was added above 2-furanylmagnesium solution (2.0 mL) via syringe at −78 ˚C over 5 min. The mixture was stirred at −78 ˚C for another 45 min. Then into this mixture acetone (1.0 mL) was added at −78 ˚C, followed by addition of EtOAc (30 mL). At 0 ˚C, saturated aqueous NH4Cl solution (15 mL) was added to wash the organic layer. The aqueous layer was extracted with EtOAc (2 x 30 mL). The pooled organic layer was washed with saturated brine (15 mL), dried over Na2SO4, and concentrated under reduced pressure to give a residue. Crude 1H NMR showed that the ratio of remaining starting material / acylfuran 7 / ester 12 to be 2.5 : 1.4 : 1.0.
Carbonyl addition of imide 10 with 2-furanyl copper:
Into a flask with a sample of CuCN (54 mg, 0.60 mmol) was added 2-furanyllithium (1.1 mL, 0.7 mmol) in THF at −78 ˚C. The mixture was stirred at −25 ˚C for 30 min., and then cooled back to −78 ˚C. Into this mixture, a solution of imide 10 (47 mg, 0.21 mmol) in THF (1.0 mL) was added via syringe at −78 ˚C over 5 min. The mixture was stirred at −78 ˚C for another 30 min. Then into this mixture acetone (1.0 mL) was added at −78 ˚C, followed by addition of EtOAc (30 mL). At 0 ˚C, saturated aqueous NH4Cl solution (15 mL) was added to wash the organic layer. The aqueous layer was extracted with EtOAc (2 x 30 mL). The pooled organic layer was washed with saturated brine (15 mL), dried over Na2SO4, and concentrated under reduced pressure to give a residue. Crude 1H NMR showed that there was no product acylfuran 7 and ester 12 besides the starting material.
Carbonyl addition of imide 10 with lithium di-2-furanylcuprate:
Into a flask with a sample of CuCN (45 mg, 0.50 mmol) was added 2-furanyllithium (1.6 mL, 0.7 M, 1.1 mmol) in THF at −78 ˚C. The mixture was stirred at −25 ˚C for 30 min and then cooled back to −78 ˚C. Into this cuprate solution, a solution of imide 10 (112 mg, 0.51 mmol) in THF (2.0 mL) was added via syringe at −78 ˚C over 5 min. The mixture was stirred at −78 ˚C for another 30 min. Then into this mixture acetone (1.0 mL) was added at −78 ˚C, followed by addition of EtOAc (30 mL). At 0 ˚C, saturated aqueous NH4Cl solution (15 mL) was added to wash the organic layer. The aqueous layer was extracted with EtOAc (2 x 30 mL). The pooled organic layer was washed with saturated brine (15 mL), dried over Na2SO4, and concentrated under reduced pressure to give a residue. Crude 1H NMR showed that the ratio of remaining starting material / acylfuran 7 / ester 12 to be 0.8 : 1.7 : 1.0.
Benzyl 4-(furan-2-yl)-4-oxobutylcarbamate (7)
Preparation of 2-furanyllithium: to a stirred neat furan (4 mL) in a 50 mL flask was slowly added n-BuLi (6 mL, 2.5 M, 15 mmol) via syringe at 0 ˚C under Ar atmosphere. After stirring at 0 ˚C, the ice bath was removed and the mixture was allowed to stir at rt overnight resulting in a mixture with yellow suspension. Then an additional amount of THF (5 mL) was slowly added (total volume of 12.5 mL) to dissolve the yellow solid. An aliquot of this vinyl lithium solution (10 mL, 12 mmol) was then added to a solution of imide 10 (2.33 g, 10.6 mmol) via syringe at −78 ˚C over 20 min. The mixture continued to be stirred at −78 ˚C for 15 min. Then into this mixture acetone (3 mL) was added at −78 ˚C, followed by addition of EtOAc (50 mL). Then at 0 ˚C, saturated aqueous NH4Cl solution (45 mL) was added to wash the organic layer. The aqueous layer was extracted with EtOAc (3 x 100 mL). The pooled organic layer was washed with saturated brine (45 mL), dried over Na2SO4, and concentrated under reduced pressure to give a residue. Crude 1H NMR of this residue showed that ratio of 7 over 12 to be 2:1. Then the residue was loaded onto silica gel column. Elution with hexane-EtOAc (6:1 v/v) gave 0.44 g ester 12 (21%), elution with hexane-EtOAc (2:1 v/v) gave desired acylfuran 7 1.50 g (50%) and elution with hexane-EtOAc (1.5:1 v/v) recovered 0.28 g starting material 10 (11%).
7:2c Colorless oil; Rf = 0.45 (1:1 v/v EtOAc/hexane); 1H NMR (600 MHz, CDCl3) δ 7.56 (m, 1H), 7.28-7.36 (m, 5H), 7.17 (d, J = 3.0 Hz, 1H), 6.51 (dd, J = 3.0, 1.2 Hz, 1H), 5.08 (s, 2H), 4.98 (br, 1H), 3.28 (dt, J = 6.0, 6.0 Hz, 2H), 2.88 (t, J = 7.2 Hz, 2H), 1.94 (tt, J = 7.2, 6.6 Hz, 2H); 13C NMR (150 MHz, CDCl3) δ188.8, 156.4, 152.5, 146.3, 136.6, 128.4, 128.0, 117.0, 112.2, 66.6, 40.5, 35.5, 24.2. ClHRMS: Calculated for [C16H17NO4H+]: 288.1236, found: 288.1235.
12: Colorless oil; Rf = 0.74 (1:1 v/v EtOAc/hexane); 1H NMR (600 MHz, CDCl3) δ 7.58 (dd, J = 1.8, 1.2 Hz 1H), 7.44 (m, 2H), 7.38 (m, 2H), 7.34 (m, 1H), 7.21 (dd, J = 3.6, 0.6 Hz, 1H), 6.50 (dd, J = 3.6, 1.8 Hz, 1H); 13C NMR (150 MHz, CDCl3) δ 158.5, 146.4, 144.6, 135.6, 128.6, 128.4, 128.3, 118.1, 111.8, 66.5.
Benzyl-3-((3aS, 4S, 6R, 7aR)-4-(benzyloxy)-tetrahydro-2, 2-dimethyl-7-oxo-3aH-[1,3]dioxolo[4,5-c] pyran-6-yl)propylcarbamate (5)
To a stirred solution of the pyranone 6 (395 mg, 1.0 mmol) in MeCN (12 mL) at 0-5 °C (ice/water bath) was added a solution of RuCl3•3H2O, (17.8 mg, 0.07 mmol) and NaIO4 (321 mg, 1.5 mmol) in distilled water (2 mL). The reaction mixture was stirred vigorously for 4 min, as a white precipitate formed. The suspension was filtered through a short silica gel pad eluting with EtOAc to give a crude diol, which was used without further purification. P-T acid monohydrate (18.6 mg, 10 mol%) was added to a stirred solution of the crude diol and 2,2-dimethoxypropane (2.82 mL) in acetone (15 mL) for 20 min at 45 °C. The reaction mixture was quenched with sodium bicarbonate solution (5 mL), removed acetone in vacuo, extracted with Et2O (3 x 25 mL), dried (Na2SO4), and concentrated under reduced pressure. The crude product was purified using silica gel flash chromatography eluting with 50% Et2O/hexane to give acetonide 5 (141 mg, 0.3 mmol, 30%) as colorless oil; Rf = 0.53 (40% EtOAc/hexane); [α]25D + 83 (c 1.0, CH2Cl2); IR (thin film, cm-1) ν 3376, 2934, 1709, 1521, 1455, 1229, 1073, 1018, 910, 857; 1H NMR (270 MHz, CDCl3) δ 7.27-7.39 (m, 10H), 5.09 (s, 2H), 5.04 (s, 1H), 5.0 (br., 1H), 4.72 (d, J = 11.8 Hz, 1H), 4.57 (d, J = 11.8 Hz, 1H), 4.47 (d, J = 6.6, 1H), 4.43 (d, J = 6.6, 1H), 4.18 (dd, J = 8.4, 4.8 Hz, 1H), 3.2 (m, 2H), 1.22-1.92 (m, 4H), 1.46 (s, 3H), 1.35 (s, 3H); 13C NMR (67.5 MHz, CDCl3) δ 204.0, 156.4, 136.6, 136.3, 128.6, 128.5, 128.2, 128.1, 128.0 (2 C), 111.4, 95.9, 78.6, 75.8, 73.4, 70.0, 66.6, 40.7, 27.6, 26.7, 25.9, 25.4; CIHRMS Calculated for [C26H31O7NNa+]: 492.1998, found: 492.1999.2c
ACKNOWLEDGEMENTS
We are grateful to NSF (CHE-0749451) and the ACS-PRF (47094-AC1) for the support of our research program. Funding by the National Science Foundation (NSF-EPSCoR award #0314742) for a 600 MHz spectrometer and NMR facility is gratefully acknowledged.
References
1. This paper is in memory of Professor John W. Daly.
2. (a) H. Guo and G. A. O’Doherty, Org. Lett., 2006, 8, 1609; CrossRef (b) H. Guo and G. A. O’Doherty, Tetrahedron, 2008, 64, 304; CrossRef (c) J. N. Abrams, R. S. Babu, H. Guo, D. Le, J. Le, J. M. Osbourn, and G. A. O’Doherty, J. Org. Chem., 2008, 73, 1935. CrossRef
3. (a) B. Dudot, L. Micouin, I. Baussanne, and J. Royer, Synthesis, 1999, 688; CrossRef (b) G. E. Keck and E. R. Romer, J. Org. Chem., 1993, 58, 6083; CrossRef (c) J. Bi and V. K. Aggarwal, Chem. Commun., 2008, 120; CrossRef (d) Y. G. Kim and J. K. Cha, Tetrahedron Lett., 1989, 30, 5721; CrossRef (e) K. Tadano, Y. Hotta, M. Morita, T. Suami, B. Winchester, and I. Cenci di Bello, Bull. Chem. Soc. Jpn., 1987, 60, 3667; CrossRef (f) K. Tadano, Y. Hotta, M. Morita, T. Suami, B. Winchester, and I. Cenci di Bello, I. Chem. Lett., 1986, 2105. CrossRef
4. A. Giovannini, D. Savoia, and A. Umani-Ronchi, J. Org. Chem., 1989, 54, 228. CrossRef
5. (a) A. Fujii, S. Hashiguchi, N. Uematsu, T. Ikariya, and R. Noyori, J. Am. Chem. Soc., 1996, 118, 2521; CrossRef (b) R. Noyori and T. Ohkuma, Angew. Chem., Int. Ed., 2001, 40, 40; CrossRef (c) H. Guo and G. A. O’Doherty, Org. Lett., 2005, 7, 3921; CrossRef (d) M. H. Haukaas and G. A. O’Doherty, Org. Lett., 2001, 3, 3899. And see ref. 2. CrossRef
6. O. Achmatowicz and R. Bielski, Carbohydr. Res., 1977, 55, 165. CrossRef
7. (a) R. S. Babu and G. A. O’Doherty, J. Am. Chem. Soc., 2003, 125, 12406; CrossRef (b) R. S. Babu, M. Zhou, and G. A. O’Doherty, J. Am. Chem. Soc., 2004, 126, 3428; CrossRef For examples see: (c) S. R. Guppi, M. Zhou, and G. A. O‘Doherty, Org. Lett., 2006, 8, 293; CrossRef (d) M. Zhou and G. A. O’Doherty, Org. Lett., 2006, 8, 4339; CrossRef (e) M. Shan and G. A. O’Doherty, Org. Lett., 2006, 8, 5149; CrossRef (f) H. Guo and G. A. O‘Doherty, Angew. Chem. Int. Ed., 2007, 46, 5206; CrossRef (g) H. Guo and G. A. O’Doherty, J. Org. Chem., 2008, 73, 5211. CrossRef
8. T. K. M. Shing, E. K. W. Tam, V. W.-F. Tai, I. H. F. Chung, and Q. Jiang, Chem. Eur. J., 1996, 2, 50. CrossRef
9. Only new procedures are reported in this paper, for the conversion of 7 to 6 and 5 to 3, see ref. 2c.
10. The ratios of desired product 7 over ester 12 could range from 1.5 to 2.0 in different runs.
11. K. Saigo, M. Usui, K. Kikuchi, E. Shimada, and T. Mukaiyama, Bull. Chem. Soc. Jpn., 1977, 50, 1863. CrossRef