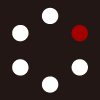
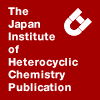
HETEROCYCLES
An International Journal for Reviews and Communications in Heterocyclic ChemistryWeb Edition ISSN: 1881-0942
Published online by The Japan Institute of Heterocyclic Chemistry
e-Journal
Full Text HTML
Received, 17th September, 2008, Accepted, 15th October, 2008, Published online, 23rd October, 2008.
DOI: 10.3987/COM-08-S(D)23
■ Reaction of β-Trifluoroacetylketene Acetals and β-Trifluoroacetylvinyl Ethers with 1,2-Phenylenediamines Accessing Fluorine-Containing Benzo[b][1,4]diazepine Derivatives: A Molecular Orbital Calculation Study
Norio Ota, Yasuhiro Kamitori,* Naoya Terai, Tsuneaki Sakata, and Etsuji Okada*
Department of Chemical Science and Engineering, Graduate School of Engineering, Kobe University, 1-1 Rokkodai-cho, Nada-ku, Kobe 657-8501, Japan
Abstract
β-Trifluoroacetylketene acetals (1) were found to react easily with 1,2-phenylenediamines to give dihydrobenzodiazepinols (5a) together with benzodiazepines (6a) under very mild conditions. In contrast, β-trifluoroacetyl-α-phenylvinyl ethers (2) and β-trifluoroacetylvinyl ethers (3) exclusively yielded O-N exchanged products (9) when they were reacted with 1,2-phenylenediamines. The factors determining product formation by the reaction of each of three similar substrates (1-3) with 1,2-phenylenediamine were elucidated on the basis of molecular orbital calculations. The dehydration processes converting dihydrobenzodiazepinols (5 and 7) to the corresponding benzodiazepines (6 and 8) are also discussed.INTRODUCTION
Recently much attention has focused on developing new methodologies for the syntheses of many kinds of fluorine-containing heterocycles, as these compounds are now widely recognized as important organic materials showing specific functions and interesting biological activities.1-4 In our preceding papers, we reported facile and convenient methods for synthesizing fluorine-containing dihydrobenzo[b][1,4]diazepinols and benzo[b][1,4]diazepines, which have remarkable anti-tumor activities,5 from β-trifluoroacetylketene acetals6 and β,β-bis(trifluoroacetyl)vinyl ethers.7 In these investigations, we found that β-trifluoroacetylketene dimethyl acetal (1) readily reacts with 1,2-phenylenediamine under very mild conditions to give 2,3-dihydro-1H-benzo[b][1,4]diazepin-2-ol (5a) as the major product and 3H-benzo[b][1,4]diazepine (6a) as the minor product. In contrast, only O-N exchanged products (9) were obtained by the reaction of β-trifluoroacetylvinyl iso-butyl ether (3) with 1,2-phenylenediamine. Bonacorso et al. reported that 9b was the sole product of the reaction of β-trifluoroacetyl-α-phenylvinyl methyl ether (2) with 1,2-phenylenediamine.8 In addition, it has been reported that 3H-benzo[b][1,4]diazepine (6b) is obtained by heating 2 with 1,2-phenylenediamine in the presence of acetic acid.8 In contrast, neither heating nor acid catalysis is necessary for the reaction of 1 with 1,2-phenylenediamine to produce benzodiazepinol (5a) and benzodiazepine (6a).6 However, no benzodiazepine derivatives were obtained even when 3 was heated with 1,2-phenylenediamine in the presence of an acid catalyst.
We also found that β,β-bis(trifluoroacetyl)vinyl ether (4) reacts with 1,2-phenylenediamine under very mild conditions to give 2,5-dihydro-1H-benzo[b][1,4]diazepin-2-ol (7d).7 Moreover, it was reported that the reaction of 4 with 1,2-phenylenediamine under microwave irradiation afforded 1H-benzo[b][1,4]diazepine (8d).9 When 1 was reacted with 1,2-phenylenediamine, only 5a and 6a were obtained, with no formation of benzodiazepinol (7a) or benzodiazepine (8a).
Using molecular orbital calculations, we previously elucidated the reaction of β,β-bis(trifluoroacetyl)vinyl ether (4) with 1,2-phenylenediamine, affording benzodiazepinol (7a).10 In the present report, the factors determining the products obtained by the reaction of each of three similar substrates (1-3) with 1,2-phenylenediamine are clarified using molecular orbital calculations. Also, the dehydration of dihydrobenzodiazepinols (5 and 7) to benzodiazepines (6 and 8) is discussed.
RESULTS AND DISCUSSION
Similar to β,β-bis(trifluoroacetyl)vinyl ethers,11 β-trifluoroacetyl-α-phenylvinyl ethers,12 and β-trifluoroacetylvinyl ethers,13 β-trifluoroacetylketene acetals readily undergo nucleophilic O-N exchange reactions at olefinic carbons with various aliphatic and aromatic amines to give the corresponding β-trifluoroacetylated ketene O,N-acetals.14 Consequently, the O-N exchanged product (9a) depicted in Scheme 1 was thought to be the initial intermediate in the reaction of β-trifluoroacetylketene dimethyl acetal (1) with 1,2-phenylenediamine. The theory was similar to the cases of 9b-d which were supposed to be the initial intermediates in the reaction of β-trifluoroacetyl-α-phenylvinyl methyl ether (2), β-trifluoroacetylvinyl iso-butyl ethers (3), and β,β-bis(trifluoroacetyl)vinyl iso-butyl ether (4), respectively, with 1,2-phenylenediamine.10
The subsequent intramolecular nucleophilic addition of the aromatic NH2 group to the trifluoroacetyl carbonyl group in 9a would proceed to give dihydrobenzodiazepinol 7a, whereas a similar cyclization reaction would not take place in 9b and 9c. Dehydrobenzodiazepinol (5a) and benzodiazepine (6a) are thought to derive from 7a, if the isomerization of 7a to 5a and the dehydration of 5a to 6a are possible, as shown in Scheme 2.
As an initial step to elucidate the difference in reactivity of 9a-c, we computed the most stable structures of 9a-c and their energies (E9) using RB3LYP/6-31G*//RB3LYP/6-31G*. As illustrated in Scheme 3, transformation from the most stable conformers (9) to 10, which are suitable for subsequent cyclization, would be required for the conversion of 9 to the corresponding dihydrobenzodiazepinols (7). We presumed that the ease of cyclization of 9 to 7 would be correlated with the energy difference between 9 and 10. Structural optimization was performed for conformers (10a-c), and ΔE9-10 (E10-E9) values were calculated. Table 1 summarizes the values of ΔE9-10, together with the energies of 9a-c (E9) and 10a-c (E 10).
The largest ΔE9-10 was estimated for the transformation of 9c to 10c. This result seems to explain the extremely slow cyclization of O-N exchanged product 9c to dihydrobenzodiazepinol 7c, resulting in the reaction of β-trifluoroacetylvinyl iso-butyl ether (3) with 1,2-phenylenediamine to afford 9c as the sole product. However, the difference in ΔE9-10 between 9a and 9c was estimated to be no more than 1 kcal/mol. Moreover, ΔE9-10 for 9b is smaller than that for 9a. These results are incompatible with the experimental results, where 9a cyclized easily to give dihydrobenzodiazepinol (5a) and benzodiazepine (6a) via 7a,6 while 9b and 9c did not cyclize to any benzodiazepine derivative under similar conditions. Therefore, the conformational change required to convert 9 to 10 is not so important energetically for the overall cyclization process from 9 to 7. This means that the cyclization process from conformers (10) to dihydrobenzodiazepinols (7) is a key step determining whether O-N exchanged products 9 are converted to 7 or not.
As a second step, we tried to elucidate the transition state for the cyclization reaction from 10 to 7. Our attempts to compute the transition state structures for the cyclization reactions of 10a-c resulted in failure. Therefore, we focused on intramolecular frontier orbital interactions, i.e., the interactions between the nitrogen in the NH2 group (HOMO) and the carbonyl carbon in the COCF3 group (LUMO) for conformers (10a-c). Frontier electron densities, frHOMO at NH2 and frLUMO at COCF3 for 10a-c are shown in Table 2.
Both frHOMO and frLUMO in 10a are larger than those in 10b. These results suggest that the intramolecular HOMO-LUMO interaction in 10a is considerably greater than that in 10b. Strong intramolecular frontier orbital interaction is expected to promote the cyclization of 10a to 7a under very mild reaction conditions. In contrast, intramolecular HOMO-LUMO interaction in 10b would not be large enough to mediate the cyclization to 7b. On the other hand, the values of frHOMO and frLUMO in 10c are considerably larger than those in 10b, indicating that the intramolecular HOMO-LUMO interaction in 10c would be comparable with that in 10a. Therefore, these data are in conflict with our experimental results where O-N exchanged product (9c) did not cyclize to dihydrobenzodiazepinol (7c).
It is necessary to take into account steric factors in enamine (9) to explain the lack of cyclization of 9c. Steric repulsion between the methoxy group and the 2-aminophenylamino group in 9a would assist the transformation from 9a to 10a. In contrast, steric repulsion promoting the conformational change from 9c, which bears no α-substituent, to 10c is unlikely. Difficulty in forming 10c from 9c is a likely reason why 9c could not be converted to dihydrobenzodiazepinol (7c).
As shown in Scheme 4, an alternative reaction to go from 9 to 7 via enol type intermediates (11) is also possible. In order to estimate the relative stability of 11 compared to 10, we computed the optimized structures of 11a-c, and calculated the energy difference (ΔE10-11) between 10a-c and the corresponding 11a-c. The results are summarized in Table 3. In all cases, enol type intermediates (11) are ca. 13 - 15 kcal/mol more unstable compared with 10. These results indicate that the cyclization of O-N exchanged products (9) to dihydrobenzodiazepinols (7) proceeds predominantly along the direct reaction pathway (Scheme 2).
Frontier electron densities, frHOMO at NH2 and frLUMO at C(OH)CF3 for 11a-c are listed in Table 3. It appears that intramolecular HOMO-LUMO interaction in 11a is rather larger than that in 11b. Thus, the trend in the intramolecular frontier orbital interaction for 11a-c is similar to that for 10a-c, and is compatible with the experimental results described above, even if the reaction from O-N exchanged products (9) to dihydrobenzodiazepinols (7) occurs partially along the reaction pathway via 11 (Scheme 4).
To clarify the relative stability of dihydrobenzodiazepinols 5 and 7, we computed optimized structures of 5a,b and 7a,b together with their energies. Our results indicate that 5a is ca. 16 kcal/mol more stable than 7a and, therefore, 7a generated by cyclization of 9a would immediately isomerize to the more stable 5a (Scheme 5). This could explain why the reaction of β-trifluoroacetylketene dimethyl acetal (1) with 1,2-phenylenediamine gives dihydrobenzodiazepinol (5a).
Similar to the case of 5a, 5b was estimated to be ca. 10 kcal/mol more stable than 7b. Therefore, isomerization from 7b to 5b is thought to occur easily, and subsequent dehydration of 5b would give 6b, if cyclization of 9b to 7b is possible. However the cyclization reaction of 9b to afford 6b requires acid catalysis.8 As discussed previously, 10 benzodiazepine (6b) would be formed from 9b along the reaction pathway illustrated in Scheme 6. Two exothermic processes, i.e., the dehydration from cation (13) to 14 and the isomerization from 8b to 6b, are thought to be key steps in the reaction of β-trifluoroacetylvinyl ether (2) with 1,2-phenylenediamine affording benzodiazepine (6b).8
Dehydration of dihydrobenzodiazepinol (5a) to benzodiazepine (6a) was estimated to be an endothermic reaction (14.4 kcal/mol; Scheme 5). This result is compatible with our experimental result where dehydration of 5a to 6a required heating (ca. 120 °C) under reduced pressure (ca. 2.5 Torr).6 Obviously, 6a obtained by the reaction of β-trifluoroacetylketene dimethyl acetal (1) with 1,2-phenylenediamine did not derive from 5a as shown in Scheme 2, because the reaction of 1 with 1,2-phenylenediamine proceeded at ambient temperature to give 6a together with 5a.
Two reaction pathways from dihydrobenzodiazepinol (7a) to benzodiazepine (6a) are possible (Scheme 7). Path A requires dehydration of 7a to benzodiazepine (15) and subsequent isomerization of 15 to 6a. Path B requires conversion of 7a to 6a via benzodiazepine (8a). The first dehydration process in both Path A and Path B is endothermic. However, ΔE (7.5 kcal/mol) between 7a and 15 is about 3 kcal/mol less than that between 7a and 8a (10.9 kcal/mol), and about 7 kcal/mol smaller than that between 5a and 6a (14.4 kcal/mol; Scheme 5). Dehydration of dihydrobenzodiazepinol (7a) arising from cyclization of 9a to 15 would proceed partially before the isomerization of 7a to 5a occurs. This is a possible reason why the reaction of 1 with 1,2-phenylenediamine gives 6a as a minor product together with 5a under very mild conditions.
CONCLUSION
On the basis of molecular orbital calculations, we can explain reasonably the preferred products formed by the individual reaction of β-trifluoroacetylketene dimethyl acetal (1), β-trifluoroacetyl-α-phenylvinyl methyl ether (2), and β-bis(trifluoroacetyl)vinyl iso-butyl ether (3) with 1,2-phenylenediamine. Intramolecular frontier orbital interaction in O-N exchanged products (10) formed as intermediates in the above reactions would be a key factor determining whether the subsequent cyclization yielding dihydrobenzodiazepinols (7) proceeds or not. Major formation of product (5a) as a result of the reaction of 1 with 1,2-phenylenediamine could be rationalized by isomerization of 7a to 5a because of the relative thermodynamic stability of 2,3-dihydro-1H-benzo[b][1,4]diazepin-2-ol (5a) compared to 2,5-dihydro-1H-benzo[b][1,4]diazepin-2-ol (7a). The minor product (6a) would not derive from 5a but would be produced from 7a via 1H-benzo[b][1,4]diazepine (15).
COMPUTATIONAL METHODS
All calculations employed in this paper were accomplished using the computer programs packages SPARTAN and PC SPARTAN 04.15 All calculations for geometrical optimizations were performed with the 6-31G* basis set at B3LYP 16 level. The starting geometries employed for all optimizations were resulted from molecular mechanics using SYBYL17 force field and subsequent semi-empirical PM318 optimizations. The calculations for energy of intermediates were also taken with the 6-31G* basis set at B3LYP level.
References
1. R. Filler and Y. Kobayashi, ‘Biomedicinal Aspects of Fluorine Chemistry,’, Kodansha & Elsevier Biomedical, Tokyo, 1982.
2. R. Filler, ‘Organofluorine Chemicals and Their Industrial Applications,’, Ellis Horwood, London, 1979.
3. J. T. Welch, Tetrahedron, 1987, 43, 3123. CrossRef
4. R. Filler, Y. Kobayashi, and L. M. Yagupolskii, ‘Organofluorine Compounds in Medicinal Chemistry and Biomedical Applications,’, Elsevier, Amsterdam, 1993 .
5. E. Okada, N. Ota, T. Tomoda, M. Fujimoto, and H. Takenaka, Jpn. Kokai Tokkyo Koho, 2006-273844, 2006.
6. N. Ota, E. Okada, N. Terai, T. Miyamura, D. Shibata, and T. Sakata, Heterocycles, 2009, 77, 983. CrossRef
7. N. Ota, T. Tomoda, N. Terai, Y. Kamitori, D. Shibata, M. Médebielle, and E. Okada, Heterocycles, 2008, 76, 1205. CrossRef
8. H. B. Bonacorso, L. M. L. Marques, N. Zanatta, and M. A. P. Martins, Synth. Commun., 2002, 32, 3225. CrossRef
9. A. C. S. Reddy, P. S. Rao, and R. V. Venkataratnam, Tetrahedron Lett., 1996, 37, 2845; CrossRef A. C. S. Reddy, P. S. Rao, and R. V. Venkataratnam, Tetrahedron, 1997, 53, 5847. CrossRef
10. N. Ota, Y. Kamitori, T. Tomoda, N. Terai, and E. Okada, Heterocycles, 2009, 77, 461. CrossRef
11. M. Hojo, R. Masuda, E. Okada, and Y. Mochizuki, Synthesis, 1992, 455. CrossRef
12. M. Hojo, R. Masuda, and E. Okada, Synthesis, 1986, 1013. CrossRef
13. M. Hojo, R. Masuda, E. Okada, S. Sakaguchi, H. Narumiya, and K. Morimoto, Tetrahedron Lett., 1989, 30, 6173. CrossRef
14. M. Hojo, R. Masuda, E. Okada, H. Yamamoto, K. Morimoto, and K. Okada, Synthesis, 1990, 195. CrossRef
15. Wavefunction, Inc.
16. A. D. Becke, J. Chem. Phys., 1993, 98, 5648. CrossRef
17. M. Clark, R. D. Cramer III, and N. van Opdensch, J. Computational Chem., 1989, 10, 982. CrossRef
18. J. J. P. Stewart, J. Computer Aided Molecular Design, 1992, 6, 69.