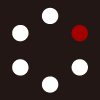
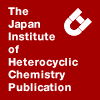
HETEROCYCLES
An International Journal for Reviews and Communications in Heterocyclic ChemistryWeb Edition ISSN: 1881-0942
Published online by The Japan Institute of Heterocyclic Chemistry
e-Journal
Full Text HTML
Received, 27th September, 2008, Accepted, 4th November, 2008, Published online, 7th November, 2008.
DOI: 10.3987/REV-08-644
■ Application of Stable Nitrenium Ions to Preparative Organic Chemistry
Yasuo Kikugawa*
Faculty of Pharmaceutical Sciences, Josai University, 1-1 Keyakidai, Sakado, Saitama 350-0295, Japan
Abstract
Nitrenium ions and their related species are involved in many important chemical and biological processes. The present review will survey the recent progress of their utility in preparative organic chemistry.CONTENTS
1 INTRODUCTION
2 USES OF NITRENIUM IONS IN ORGANIC SYNTHESIS
2-1 N-ALKYLNITRENIUM IONS
2-2 N-ARYLNITRENIUM IONS
2-3 N-ACYL-N-ARYLNITRENIUM IONS
2-4 N-METHOXY- AND N-ALLYLOXY-N-ACYLNITRENIUM IONS
2-5 N-PHTHALIMIDO-N-ACYLNITRENIUM IONS AND NITRENIUM IONS FROM AZODICARBOXYLATES
3 ADDENDUM
1 INTRODUCTION
Nitrenium ions (1) are divalent, electron-deficient nitrogen species which show umpolung of reactivity relative to the usual trivalent nitrogen species and can exist in either the singlet or triplet state as the lowest energy configuration (Figure 1).
The increased interest in nitrenium ions is mainly due to the important role of these species in carcinogenetic processes and also is related to their applications in the development of novel methods in synthetic organic chemistry. A critical survey of carcinogenetic processes that might involve these intermediates is beyond the intended scope of this review. Instead, nitrenium ions having important synthetic applications form the subject of this report. Useful reviews dealing with the general chemistry of nitrenium ions have been published.1
Subclasses of nitrenium ions include alkyl, aryl, and acyl nitrenium ions having, respectively, alkyl, aryl, and acyl groups attached to the nitrogen. The majority of these ions are reactive short-lived species that are generally difficult to identify and investigate experimentally. However, arylnitrenium ions are more stable than alkylnitrenium ions owing to delocalization of the positive charge onto the aryl ring. Furthermore, the presence of at least one powerful donor substituent on the nitrogen atom increases the stability of nitrenium ion with the singlet state being stabilized to a great extent than the triplet state. These stable long-lived nitrenium ions open up exceptional opportunities for the development of synthetically useful new reactions. Quite recently, many reports have appeared concerning practical applications of nitrenium ions in organic synthesis. The present review includes a survey of the recent advances of the chemistry of nitrenium ions and their derivatives, particularly of new chemical reactions having synthetic utility in organic synthesis.
2 USES OF NITRENIUM IONS IN ORGANIC SYNTHESIS
2-1 N-ALKYLNITRENIUM IONS
Although many N-alkylnitrenium ions are known, this section will be relatively short, since few new synthetically useful reactions of these ions have been reported. This is probably because they are short-lived and are not suitable for generation of synthetically useful intermediates. An extensive series of studies by Gassman marked the beginning of the development of the chemistry of these reactive nitrogen-containing intermediates.2
Many compounds that contain an N-Hal bond have proven to be very convenient substrates for the preparation of nitrenium ions. Scheme 1 illustrates the formation of nitrenium ions by solvolysis of 2-(cyclopent-2-enyl)-N- methylethanamine (1) in a methanol solution of silver nitrate.3
Generally arylnitrenium ions have higher stabilization energy than the aliphatic ions.2 Therefore, it can be concluded that N-alkylnitrenium ions are less applicable to synthetic organic chemistry than N-arylnitrenium ions. Alkylhydroxylamines bearing a benzene ring in a molecule were subjected to intramolecular cyclization in trifluoroacetic acid (TFA) or in the presence of Lewis acids, and benzene-fused six-menbered nitrogen heterocycles (5 and 6) were obtained in moderate yields (Scheme 2).4 However, it is not clear that the cyclization reaction proceeds via a nitrenium ion intermediate.
Nonetheless, it is evident that an electrophilic electron-deficient nitrogen must play an important role in this reaction because an electron-donating substituent on the benzene ring facilitates the cyclization reaction.
2-2 N-ARYLNITRENIUM IONS
N-Arylnitrenium ions are expected to be more stable than alkyl substituted ions owing to the possible delocalization of the positive charge onto the aryl ring. Nucleophilic attack on these intermediates results in the introduction of various substituents on the aryl ring. Gassman, et al. first reported a synthetic method for preparation of anisoles (8) and derivatives (10) of 2,5-cyclohexadienone by solvolysis of N-chloroanilines (7 and 9) through a nitrenium ion intermediate in a methanol solution of silver trifluoroacetate (Scheme 3).5
Many compounds that contain an azide group are very convenient substrates for the preparation of arylnitrenium ions. Olah, et al. reported that phenyl azide/trifluoromethanesulfonic acid (TFMSA) is a highly efficient reagent for the electrophilic phenylamination of aromatics.6 Phenylnitrenium ions generated from phenyl azides in the presence of TFMSA/TFA undergo nucleophilic substitution reactions, that is, aromatic N-substitutions and/or C-substitutions, depending on the initial azide structure.7
1-(m-Azidobenzyl)-1,2,3,4-tetrahydroisoquinolines (11) with TFMSA/TFA afforded the corresponding 9- and 11-aminoaporphines (12 and 13).8 Similarly, TFMSA catalyzed decomposition of 1-(3-azidophenyl)pyrroles (14) produced the 6- and 8-aminopyrrolo[1,2-f]phenanthridines (16 and 17) through cyclization of the intermediate arylnitrenium ions (15) in good overall yields.9 Medium-sized rings were formed by a similar methodology and 3-aminodibenzo[a,c]octadiene (19) (28%) and 21 (70%) were synthesized by the intramolecular cyclization of arylnitrenium ions generated from azides (18 and 20) with TFMSA.10 Generally 6-menbered ring formation is favorable. However, in a special case, the 16-menbered ring (23) was formed by a nitrenium ion upon an aromatic nucleus (Scheme 4).8
Phenylnitrenium ions generated from phenylhydrazines in the presence of TFMSA undergo intramolecular aromatic C-substitutions to afford cyclized products. Treatment of N-phenyl-N-(4-phenylbutyl)hydrazine (24, n = 4) in TFMSA at 80 °C for 30 min produced dibenz[b,d]azonine (25, n = 4) in 38% yield (Scheme 5).11
Similarly, phenylnitrenium ions generated from phenylhydrazines (26) in the presence of AlCl3 were trapped with solvent arenes to give both N-substitution and C-substitution products (27 and 28), while an N-methyl-N-phenylnitrenium ion generated from N-methyl-N-phenylhydrazine (26b) reacted with arenes to give exclusively aromatic C-substitution products 29b (Scheme 6).12 Reaction of N-arylaminophthalimide derivatives with AlCl3 in benzene results in heterolytic N-N bond cleavage to give an arylnitrenium ion and canonical forms involving the arene. These species are trapped by benzene to give aminobiaryls and N-arylanilines.13
In an extension of this work the reaction of N-(N,N-diarylamino)phthalimides (30) with AlCl3 in benzene was investigated, with the expectation that AlCl3-mediated cleavage of the N-N bond would give a diarylnitrenium ion (31a).14
Treatment of N-(N,N-diphenylamino)phthalimide (30a) with AlCl3 in benzene for 4 h at rt gave carbazole (32a) along with N-p-biphenyl-N-phenylamine (33a). The formation of these products indicates that AlCl3-mediated heterolytic cleavage of the N-N bond in fact produces a
diphenylnitrenium ion. Arylation of the phenylnitrenium intermediate could occur at three sites (nitrogen, the para ring carbon and the ortho ring carbon). In the present case, the ortho ring carbon is attacked intramolecularly by the adjacent ortho ring carbon to form a new C-C bond (Scheme 7). The results are presented in Table 1.
In the case of methoxy-substituted substrates 30, the reaction did not proceed smoothly. Therefore, for the synthesis of methoxycarbazoles, the corresponding bromocarbazoles are synthesized by the above method. Subsequent replacement of the bromine atom with a methoxy group by the published procedure produces the methoxycarbzoles.15 This methodology was applied to the synthesis of a carbazole alkaloid, 1-methoxy-3-methyl-9H-carbazole (murrayafoline) (34).14
Falvey et al. reported that photolysis of protonated 1,1-diarylhydrazines and N,N-(diarylamino)-2,4,6-trimethylpyridinium ions generates the corresponding nitrenium ions. These were trapped by added Cl- to give chlorinated N-phenylanilines. There was negligible cyclization to form carbazoles.16
2-3 N-ACYL-N-ARYLNITRENIUM IONS
Although the rearrangement of N-alkoxy- and N-hydroxy-N-arylamides 35 has not been investigated in detail in comparison with those of arylazides and of N-arylhydroxylamines, it is generally assumed that these amides decompose under acidic conditions via N-O bond heterolysis to yield N-acyl-N-arylnitrenium ions 36. These have a positive charge on the nitrogen that is delocalized among the canonical forms involving the aryl group. Nucleophilic attack results in the introduction of various substituents on the aryl ring (Scheme 9).
1-Hydroxy-2-oxindole (37) was converted to the unstable p-toluenesulfonate at -78 °C. Warming the p-toluenesulfonate to rt with addition of methanol brought about the formation of 5-methoxy- and 7-(p-toluenesulfonyloxy)-2-oxindole in 42 % and 34 % yields, respectively.17 The same compound 40b was synthesized from 1-hydroxy- and 1-methoxy-2-oxindoles (37 and 38) under the conditions of refluxing methanol containing few drops of concentrated sulfuric acid.18 The initial product was a ring-opened methyl 2-amino-5-methoxyphenyl acetate (39). Subsequent cyclization gave 5-methoxy-2-oxindole (40b) in 72 % yield. Conversion of 40b to 5-methoxyindole (42) was accomplished in 66 % yield by chlorination of 40b with triphenylphosphine-carbon tetrachloride in acetonitrile, followed by the catalytic hydrogenolysis of the chlorine atom from 41 (Scheme10).
No ring opening was observed in the case of a six-membered ring. Thus, under similar acidic reaction conditions, 6-substituted-3,4-dihydrocarbostyrils (44) were obtained from 3,4-dihydro-1-methoxy- or 1-hydroxy-carbostyril (43) in moderate to good yields (Figure 2).19 N-Methoxy- or N-hydroxy-N-phenylbenzamide underwent rearrangement in 20 % hydrochloric acid, or in benzene with
thionyl chloride, to give 4-chloroaniline (46a) or N-(2-chlorophenyl)benzamide (46b) in 25 % or in 84 % yield, respectively (Scheme 11).20, 21
In a manner similar to that described above, introduction of a fluorine atom into the aryl group was attempted using concentrated hydrogen fluoride. Howerver, this was unsuccessful because of the weak nucleophilicity of the fluoride ion in these protic reaction conditions. However, treatment of N-aryl-N-hydroxyamides with diethylaminosulfur trifluoride (DAST) 22 in dichloromethane for 5 min with cooling, resulted in the removal of the hydroxy function and the introduction of a fluorine atom at the para position of the aromatic ring in high yield (Scheme 12).23 DAST is a commercially available reagent and often used for replacement of the hydroxyl group with fluorine. The merits of this method include regioselective introduction of a fluorine atom to an aromatic ring (the para position only), use of a commercially available reagent, high yields, short reaction times and technical simplicity.
AlCl3-mediated decomposition of N-methoxy-N-phenylamides 53 in 1,2-dichloroethane has been reported to provide a new source of N-acyl-N-arylnitrenium ions 54 that undergo nucleophilic intramolecular migration of the methoxy group from the nitrogen to the ortho position of the phenyl ring to give N-(2-methoxyphenyl)amides 55.24 N-Methoxycinnamamides bearing a-substituent groups rearrange rapidly in high yields (Scheme 13), while 1-methoxycarbostyrils do not, even with prolonged reaction time. The most interesting aspect of this reaction is that the rearrangement of 54 gave exclusively ortho methoxy compounds 55. The complete absence of the corresponding para-substituted product can be accounted for by assuming a mechanism involving a tight ion pair intermediate 54.
A similar transhydroxylation of N-phenylamides by the system (n-Bu)3P-CCl4-CH3CN was reported.25 The hydroxy group of N-hydroxy-N-phenylamide (45b, R = Ph, X = H) rearranged mainly to the ortho position of the N-phenyl group and to the para position to a much lesser extent. It is interesting to note that use of less than the stoichiometric amount of (n-Bu)3P (0.55 molar eq.) is effective for the reaction, although a somewhat longer reaction time is required for completion.
As described above, the N-O bond heterolyses in acidic conditions generate nitrenium ions. Recently, it was reported that some oxidants such as phenyliodine(III) bis(trifluoroacetate) (PIFA) react with an N-H group of N-arylamides in TFA to yield N-acylnitrenium ions. Hypervalent iodine reagents such as PIFA have received a great deal of attention due to their ready availability, low toxicity, easy handling, and similar reactivity to that of heavy metal reagents (vide post, 2-4).
The reaction of anilides with PIFA in TFA, TFA-CHCl3 or hexafluoroisopropyl alcohol (HFIP) was reported.26 When the acyl group of the anilide is highly electronegative such as trifluoroacetyl or the
phenyl group is substituted with an electron-withdrawing group such as chloro or ethoxycarbonyl group, the 4-iodophenyl group is transferred from PIFA to the amide nitrogen of 56 to afford acetyldiarylamines (57). The N-iodophenylation reaction rate was remarkably increased using HFIP as solvent. Several acetyldiarylamines having an iodo group on the para position were prepared in high yield even in the case of sterically crowded 2,6-dichloroacetanilide. The proposed reaction mechanism is illustrated in Scheme 14.26
On the other hand, when the acyl group contains an electron-donating function such as 4-methoxyphenyl or the phenyl group is substituted with an electron-donating group, a trifluoroacetoxy group is transferred to the para position of the anilide aromatic ring. This group is hydrolyzed during work-up to produce the corresponding phenol. Both reactions occur in synthetically useful yields (Tables 3 and 4).26
A novel approach to the synthesis of a wide variety of heterocycles using PIFA was undertaken based on this strategy (Scheme 15).
The oxidation of conveniently substituted N-arylamides (62 and 64) by PIFA affords the corresponding N-acylnitrenium ions. These can be intramolecularly trapped with amine functionalities and with thiol functionalities leading to the formation of new N-N and N-S bonds producing indazol-3-ones 63 and benzothiazol-3-ones 65, respectively. In the synthesis of 63, success is restricted to N-arylamides. N-Alkyl- or N-alkoxy-amides failed to afford 63, which indicates that an aromatic ring seems to be
important to stabilize the corresponding N-acylnitrenium intermediate (Scheme 16).27
On the other hand, both N-arylamides and N-alkylamides successfully generate the corresponding nitrenium ions with PIFA to produce the benzothiazolones (65).28
Other approaches to the synthesis of nitrogen heterocycles using PIFA have been investigated. PIFA promotes the intramolecular electrophilic olefin amidation to afford five- and six-menbered nitrogen heterocycles.29, 30 From 2-allyl-N-(phenyl or 4,5-dimethoxyphenyl)benzamide derivatives (66a and b) the isoquinolin-1-one derivatives (67a and b) were obtained by PIFA-promoted olefin amidohydroxylation reactions in 2,2,2-trifluoroethanol (TFEA).29 Similarly, when the amides 68a and b were treated with PIFA in TFEA at rt, indolines (69a and b) were obtained in moderate yields. These were transformed into the corresponding acetyl derivatives due to their labile nature.30a In other solvent such as CH2Cl2 and CH3CN no indolines were obtained.
Pyrrolidine and piperidine derivatives (71) were also prepared through 5-exo-trig and 6-exo-trig cyclization modes by PIFA-promoted olefin amidohydroxylation of compounds 70.30b Analogously, the oxidation of alkynylamides 72 by PIFA leads to the generation of N-acylnitrenium ions. These are trapped by intramolcular reaction with a triple bond producing the pyrrolidinones 73. In this case also the solvent is limited to TFEA (Scheme 17).31
2-4 N- METHOXY- AND N-ALLYLOXY-N-ACYLNITRENIUM IONS
Nitreniuon ions in general are quite short-lived electrophilic reactive intermediates. In order to increase their utility in organic synthesis, it would be advantageous to prolong their lifetimes. It is reasonable to suspect that this could be accomplished by the presence of at least one powerful electron donor substituent on the nitrogen atom that would stabilize the positively charged nitrenium ion. Therefore, the selection of the appropriate N-substituent is of key importance. Among the most easily formed and useful members of this class are N-methoxy-N-acylnitrenium ions. N-Methoxy-N-acylnitrenium ions, which were first reported independently by Glover32 and Kikugawa33 in 1984, proved to be the most easily formed and useful electrophiles, and readily undergo inter- and intra-molecular substitution reactions with a range of aromatic ring systems.
They can be generated by the treatment of the corresponding N-methoxy-N-chloroamides with a variety of Lewis acids, typically silver32, 33a, b or zinc ions.33c A number of nitrogen heterocyclic compounds bearing an N-methoxy group have been synthesized using these methods (Scheme 18). The synthetic success of this substitution is mainly due to the fact that ions 76 are stabilized by the electron-donating effect of the adjacent methoxy group and exist long enough to react with an aromatic ring.
In addition to the N-methoxy group, other N-alkoxy groups may be used in these reactions. Benzyl and methoxyethoxymethyl groups are not suitable. A nitrogen heterocyclic compound bearing an N-allyloxy group can be synthesized by the procedure described above and this is readily converted to the corresponding N-hydroxy compound by palladium-catalyzed removal of the allyl group.34
Since nitrogen heterocyclic compounds such as 38 and 43 bearing a methoxyamido group have been successfully modified by the introduction of a methoxy group para to the nitrogen (vide ante, 2-3), this methodology will offer a new route for the synthesis of not only nitrogen heterocyclic rings, but also natural products bearing nitrogen heterocyclic rings and oxygen functions.
Thus, a convenient synthesis of the oxindole moiety of gelsemine (81)35 and a total synthesis of 6-methoxy-5-methylbenzo[h]pyrrolo[4,3,2-de]quinolin-4(5H)-one (eupolauramine) (87)36 have been achieved (Schemes 19 and 20).
N-Chloro-N-methoxyamides are synthesized by chlorination of the corresponding N-methoxyamides with tert-butyl hypochlorite in CH2Cl2 in quantitative yields and can be used without further purification. However, because tert-butyl hypochlorite is not commercially available in most countries and is not recommended for use in bulk quantities from the standpoint of green chemistry, this method is unsuitable for large scale synthesis.37 Subsequently, limitations associated with these protocols were overcome by direct methoxyamidation of arenes using PIFA.
In the last decades hypervalent iodine compounds such as PIFA have found wide application in synthetic organic chemistry because of their low toxicity, ready availability, ease of handling, and environmentally friendly nature. PIFA was used for the generation of N-methoxy-N-acylnitrenium ions first by Kikugawa38 and successively by Romero39 who reported elegant application of this methodology for the preparation of the dopamine D2 receptor agonist PNU-95666E (95) (Scheme 21).
Notably, Wardrop has tentatively expanded the applicability of PIFA to the synthesis of nitrogen-containing biologically active natural products.40 Subsequently, PIFA has been widely used for the generation of nitrenium ions not only from N-methoxy-N-acylamides, but also from N-aryl-N-acylamides (vide ante, 2-3).
Recently Tellitu and Domínguez reported the PIFA-promoted intramolecular aromatic amidation reaction, which turned out to be a general and efficient route for the synthesis of series of naturally occurring and biologically active benzene- and heterocycle-fused compounds. Thus, the PIFA-promoted intramolecular aromatic N-methoxyamidation reaction via N-methoxy-N-acylnitrenium ion intermediates was applied for the construction of a series of N, O, and S-containing heterocycle-fused quinolinones in a general and efficient way (Scheme 22).41
Analogously, the preparation of novel benzene- and heterocycle-fused 1,4-diazepin-2-ones (101~103) from glycine or L-alanine was carried out without loss of enantiometric purity throughout the process (Scheme 23).42
Next, the PIFA-promoted intramolecular aromatic N-methoxyamidation reaction via
N-methoxy-N-acylnitrenium ion intermediates has been utilized for the synthesis of optically pure benzo-, naphtho-, and heterocycle-fused pyrroro[2,1-c][1,4]-diazepin-5,11-dione derivatives starting from L-proline methyl ester. Following this methodology an alternative synthesis of the antibiotic DC-81 (106)
has been accomplished (Scheme 24).43
Chang and Yang reported the synthesis of optically active (-)-α-aminobenzolactam (111), a key synthetic intermediate of an angiotensin converting enzyme inhibitor Benazepril (112), from commercially available L-homophenylalanine ethyl ester hydrochloride (107) using the similar PIFA-promoted intramolecular aromatic amidation reaction as a key step. The successful synthesis of 111 suggests that this strategy could be used for the synthesis of other structurally related biologically active benzolactam derivatives, such as an antithrombotic agent (CVS-1778) and a growth hormone secretagogue (L-692428) (Scheme 25).44
As described above, the intramolecular aromatic substitution reaction with N-methoxy-N-acylnitrenium ions proved to be a useful tool for the synthesis of heterocycle-fused compounds. At the same time, when the aromatic ring bears a methoxy group ortho or para to the alkyl side chain, the nitrenium ion attacks the ipso position of the aromatic ring to afford the spirodienones (114 and 116) as the major or exclusive products (Scheme 26).33b, c, 45
The dearomatization of benzene is very energetically unfavorable, and the Birch reduction46 has long been the most important dearomatising reaction of substituted benzene rings. Therefore, this unexpected reaction is a very useful transformation since this process could lead to unusual compounds which would otherwise not be readily accessible and provides valuable intermediates for an efficient synthesis of biologically important compounds. In particular this methodology opens up opportunities for expedient preparations of biologically active natural products which have the 1-azaspiro[4.5]decane and 1-azaspiro[5.5]undecane ring systems.
The utility of this methodology was demonstrated by Wordrop’s elegant syntheses of several biologically active natural products. 1-Azaspiranes prepared using this strategy served as key intermediates.40,48-50,52-54 First, Wordrop undertook the formal synthesis of the muscarinic M1 receptor antagonist (-)-TAN1251A (120) from L-tyrosine (117). The key intermediate 118 was synthesized by azaspirocyclization of the N-methoxy-N-acylnitrenium ion derived from 117 using Kikugawa’s method.33,34 No racemization occurred during reaction and 119 was converted to 120 following Kawahara’s procedure47 (Scheme 27).40
Similarly, the formal synthesis of (±)-desmethylamino FR901483 (125), a potent immunosuppressant, isolated by a group at Fujisawa, was carried out from commercially available 3-(4-methoxyphenyl)propanoic acid (121) via spirocyclization of the corresponding N-methoxy-N-acylnitrenium ion. This alkaloid has the same 1-azaspiro[4.5]decane ring system as that of (-)-TAN1251A (120) and the same synthetic strategy is applicable for the synthesis of the key intermediate (122) (Scheme 28).48
This methodology can also be used to access the 1-azaspiro[5.5]undecane ring system using 4-(4-methoxyphenyl)butanoic acid derivatives as starting compounds.
The asymmetric synthesis of the (+)-Kishi lactam (132) and a useful intermediate (133) for the synthesis of the marine natural product fasicularin (135) was performed.49 The key intermediate, anti-127, was synthesized with anti selectivity utilizing a novel strategy reported recently for the stereocontrolled preparation of 1-azaspiranes (Scheme 29).50
Since the transformation of 132 into (±)-perhydrohistrionicotoxin (134) was reported previously,51 the synthesis of (+)-132 constitutes a formal synthesis of (-)-perhydrohistrionicotoxin. In addition, 133 is a usefully functionalized intermediate for the preparation of fasicularin (135) (Scheme 30).
As shown in Scheme 29, oxidative spirocyclization of an N-acylnitrenium ion would proceed selectively to generate anti dienone. Therefore, ozonolytic cleavage of the carbon-carbon double bonds of the
dienone ring would afford a convenient route to trisubstituted azetidinone, pyrrolidinone and piperidinone derivatives (138) with useful levels of diastereoselectivity (Scheme 31).
Utilizing this expanded strategy (the nitrenium ion spirocyclization-dienone cleavage strategy), the total synthesis of (±)-adalinine (139), α,α-disubstituted piperidinone alkaloid, and the diastereoselective total synthesis of the marine natural product (-)-dysibetaine (140) were performed (Scheme 32).52, 53
Furthermore, not only (-)-dysibetaine (140), but also other biologically active compounds such as 148~151 are potentially accessible through application of this nitrenium ion spirocyclization-dienone cleavage strategy (Scheme 33).54
As described above, using the above conditions, the presence of an ortho or para methoxy substituent is essential for the spirocyclization. In order to make the process even more versatile, more readily accessible starting compounds were explored. Since 4-halogenophenyl compounds are more readily available than the corresponding methoxy compounds, the spirocyclization reaction was examined using N-methoxy-(4-halogenophenyl)amides (152) with hypervalent iodine compounds.55 The results are presented in Table 5. Generally fluorine substituted starting compounds give good results.
Use of [hydroxy(tosyloxy)iodo]benzene (HTIB) gives better yield than use of PIFA. As for solvents, TFEA proved to be the solvent of choice.
Furthermore, in TFEA unexpected results are obtained.56 Thus, treatment of unactivated 154a with PIFA in TFEA for 1 min under ice cooling afforded the benzannulated compound 155a (55%) in addition to the spirodiene compound 156a (34%). From o-tolyl derivative 154c the spirodiene compound 156c (84%) was exclusively obtained. This result was quite surprising since most reactions of unactivated monobenzenoid aromatics lead to substitution rather than addition. Ipso-Cyclization in the absence of other activating groups on the phenyl group to form the spirodiene derivatives is a very unusual result,
since this requires the loss of aromaticity. Several unactivated benzenoid compounds reacted similarly and the results are presented in Table 6.
The 1-azaspiro[4.5]decane ring system (compound 114b) is also synthesized from N-methoxy-3-(4-methoxyphenyl)propanamide (113b) by an anodic oxidation under usual constant current electrolysis conditions through a nitrenium ion intermediate.57
Not only N-methoxyamides but also N-methoxysulfonamides react with HTIB to afford the benzannulated compounds. Moreover, 2-aryl-N-methoxyethanesulfonamide bearing a 4-methoxy group at the aromatic ring generates the spiro compound 159 resulting from attack of the nitrogen to the ipso position of the molecule (Scheme 34).58 Recently it was reported that 1-methoxy-3,4-dihydro-1H-2,1-benzothiazine 2,2-dioxide (158, n = 2) was obtained from 157 (n = 2) in CH3CN in high yield with HTIB, formed in situ by a combination of iodobenzene, p-toluenesulfonic acid monohydrate, and m-chloroperoxybenzoic acid.59
2-5 N-PHTHALIMIDO-N-ACYLNITRENIUM IONS AND NITRENIUM IONS FROM AZODICARBOXYLATES
The previous section reviewed reactions of N-methoxy-N-acylnitrenium ions which are stabilized by the oxygen lone pair of a methoxy group attached to the nitrogen and are able to undergo inter- and intra-molecular substitution reactions with a range of aromatic compounds. The stabilization of nitrenium ions by other heteroatoms, such as nitrogen and sulfur, would also be expected.60 It is reasonable to predict that replacing an oxygen atom by nitrogen in the precursor would also have an additional stabilizing influence on a generated nitrenium ion. The present section describes the chemistry of nitrenium ions derived from unsymmetrical azodicarbonyl compounds and from N-acylaminophthalimides.
Intermolecular electrophilic amination of activated aromatics and other electron-rich olefins with diethyl azodicarboxylate either acid-catalysed or otherwise has already been reviewed1f and is excluded here. Therefore the intramolecular version of such a reaction is described herein, although it is uncertain that the real reaction intermediate is actually a nitrenium ion.
It is well known that azodicarboxylates are effective sources of electrophilic nitrogen.1f Treatment of the unsymmetrical hydrazides (160) with PIFA in CH2Cl2 using BF3·Et2O allowed the formation of quinolones (162), presumably via the unsymmetrical azodicarbonyl compounds (161).61
However, the reaction is limited to highly electron-rich arenes bearing such substitutents as a methoxy or dimethoxy function. This limitation is consistent with the reported behavior of an intermolecular version of a similar reaction, in which highly electron-rich arenes alone can react with azodicarboxylates.62
When arenes bear a methoxy group para to the alkyl side chain, the electron-deficient nitrogen attacks the ipso position to afford the spirodienones (163). The results are presented in Table 7.
Not only quinolones but also other important heterocyclic systems such as oxindoles, benzazepinones, benzazocinones, benzimidazolones, benzoxazinones and pyrazolones are prepared by this method.63 The results using 164 as a starting compound are presented in Table 8.
It is expected that replacing a methoxy group by nitrogen in N-methoxyamides would also have a stabilizing influence on a generated nitrenium ion. Recently, N-phthalimido-N-acylnitrenium ions (IIIa) were generated from N-acylaminophthalimides, a new class of precursors, by treatment with hypervalent iodine compounds (PIFA and HTIB), and their chemistry was investigated (Figure 4).64
It is evident that N-methoxy-N-acylnitrenium ions (IIa) are useful electrophiles, as described in section 2-4. However, the nitrenium ions (IIb) generated from 166a, 168a and 169a failed to cyclize, giving unidentifiable product mixtures.39
On the other hand, N-phthalimido-N-acylnitrenium ions (IIIb) generated from 167b, 168b and 169b readily undergo PIFA-promoted intramolecular aromatic amidation to afford benzene-fused nitrogen and oxygen heterocycles.64, 65 The PIFA-promoted cyclization reaction using IV gave ambiguous results along with recovery of the starting compound.
These facts confirm that the phthalimido group plays an important role for the stabilization of IIIa and b and for promotion of further reaction.64
A novel approach to the PIFA-promoted synthesis of a wide variety of nitrogen and oxygen heterocycles, and also spirodiene derivatives from IIIa and b has been carried out.64, 65
Treatment of 170a with PIFA (1.3 mol eq.) for 1 h in refluxing CHCl3 gave 171a in 91% yield. Several urea derivatives (170a-d, 172a, b) reacted in this way, and the results are presented in Table 9. 1,3-Dihydrobenzoimidazol-2-ones (171a-d) and 3,4-dihydro-1H-quinazolin-2-ones (173a, b) were obtained in synthetically useful yields.65
Similarly, carbamate derivatives (175a-d) were submitted to the same cyclization reaction. Treatment of 175a with PIFA (1.1 mol eq.) in TFEA for 3 h at rt gave 176a and the para-iodophenylated compound (177) in 30% and in 20% yields, respectively. It is evident that the oxygen function directly attached to the phenyl group unfavorably affected the cyclization reaction and the para-iodophenyl group of PIFA migrated to the amide nitrogen to afford 177.26 Use of 4-chlorophenyliodine(III) bis(trifluoroacetate) (p-ClPIFA) instead of PIFA in TFA improved the yield of 176a to 74%.
Several aryl carbamates (175a-d) and 175e reacted in this way, and the results are presented in Table 10. N-Phthalimido-3H-benzoxazol-2-ones (176a-d) and 4H-benzo[1,4]oxazin-3-one (176e) were obtained in synthetically useful yields.64
This reaction is influenced greatly by the choice of solvent. In the reaction of 178a with PIFA, the use of TFEA as solvent resulted in unexpected attack of the nitrenium ion on the ipso position to give a spirodiene derivative (179a) in 76% yield along with the 3,4-dihydrocarbostyril derivative (180a) (17%). From o-tolyl derivative (178c) the spirodiene compound (179c) was exclusively obtained (88%, Table 11, entry 3). Several unactivated benzenoid compounds reacted similarly and the results are presented in Table 11.
The formation of 179d can be explained as follows. The trifluoroethoxy group of the transient spirodiene intermediate can be eliminated to afford the exo methylene compound and subsequent addition of TFEA
to the methylene group would give 179d (Scheme 35, path a). It is interesting to note that the methyl group of the corresponding N-methoxyamide 154d remains intact in the similar spirodiene formation reaction (Table 6, entry 4). Compound 180d could be formed through direct ortho attack or through ipso attack followed by C-N bond migration (Scheme 35, path b).61, 63, 66
Transformation of benzenoid compounds to nonbenzenoid compounds can provide valuable intermediates for the synthesis of a variety of functionalized six-membered ring compounds.54, 67 Conversion of N- methoxy- and N-phthalimido-N-acylnitrenium ions (IIa and IIIa) to spirodienones and spirodienes bearing the nitrogen atom bound to the spiro carbon should offer valuable intermediates for the synthesis of a variety of organic compounds.
To expand the synthetic applications of IIIa, the reactions of IIIa with other functional groups have been investigated. It was assumed that olefinic moieties might play a similar role as the nucleophilic partner of
the reaction. Indeed, N-phthalimido-N-acylnitrenium ions (IIIa) readily undergo inter- and intra-molecular substitution reactions with carbon-carbon double bonds.68, 69
Initially, the reaction of N-methoxycyclohex-3-enecarboxamide with PIFA in CHCl3 was examined. However, the resulting reaction mixture contained a mixture of unidentified products (TLC). In contrast, when the N-substituent of the starting compound was changed from an N-methoxy to an N-phthalimido group, the ring closure reaction underwent smoothly and the reaction mixture contained a single product (TLC), the structure of which was determined to be the cyclized compound 182a. Cyclization reactions of other starting compounds (181b-g) were carried out similarly. The results are presented in Table 12.68
Intermolecular substitution reactions of IIIa with carbon-carbon double bond also were studied. Regioselective hydrazidohydroxylation of styrenes (184) with N-acetylaminophthalimide (185) using PIFA were performed to afford 2-(N-acetyl-N-phthalimido)aminoethyl trifluoroacetates (186) in high yield.69
Normally, the nitrenium ion (IIIa) could attack both α- and β-carbons of styrenes to afford two regioisomers. In practice, however, HPLC analysis of the reaction mixture revealed that the reaction is extremely regioselective and a single regioisomer is obtained in high yield in the case of unsubstituted and α-methyl substituted styrenes (Table 13, entries 1-9).69 With β-methyl substituted styrene (184j) two regioisomers (186j and j’) were obtained (Table 13, entry 10). As for the reaction mechanism, the hydrazidohydroxylation reaction is initiated by an electrophilic attack of IIIa on the double bond. Subsequently, without formation of aziridinium ions,70 the carbocationic species created can be captured by a trifluoroacetate anion or quenched by the elimination of an adjacent hydrogen (Table 13, entry 9) to afford the products.
It is often observed that changing the solvent can change the site attacked by a nitrenium ion. Intramolecular cyclization of N-(5-phenylpentanamido)phthalimide (187a) with PIFA in various solvents was examined with anticipation of formation of an eight-membered ring formation. However, the nitrenium ion generated by PIFA attacked the benzylic position to afford 5-phenyl-N-phthalimido-δ-lactam (188a) in 46% yield in 2,2,3,3-tetrafluoro-1-propanol and in 36% yield in TFEA.71 As it is generally accepted that a nitrenium ion attacks electron-rich site, this is the first case wherein a nitrenium ion attack at an aliphatic carbon is preferred over aromatic substitution.
Several N-(phenylpentanamido)phthalimides (187b-g) reacted in a similar way and the results are presented in Table 14.
These results show that benzylic cyclization occurs in good yields with compounds having a 4-halogenophenyl group (Table 14, entries 1-5). Compound 187g, which has an electron-withdrawing cyano group in the para-position, did not give rise to either the benzannulated or spirobenzannulated products. Instead, the 4-iodophenyl group was transferred from PIFA to the amide nitrogen to afford the acyldiarylamine 191 in 70% yield.26
Regarding the reaction mechanism, it is assumed from the by-products that PIFA initially attacks the amide moiety of 187 to afford an electron deficient nitrogen that behaves as a nitrenium ion. However, the precise reaction mechanism, especially the reason for the benzylic carbon-nitrogen bond formation, remains unclear.
3 ADDENDUM
A nitrenium ion is a nitrogen atom which bears a formal positive charge and two covalent bonds. It is not easy to clarify whether all the electrophilic nitrogens in the present review should be truly regarded as nitrenium ions from the strict definition. Therefore it should be emphasized that the proposed mechanisms concerning nitrenium ion intermediates are often speculative.
References
1. (a) R. A. Abramovitch and R. Jeyaraman, ‘Nitrenium Ions, in Azides and Nitrenes’, ed. by E. F. V. Scriven, Academic Press, Inc., New York, 1984, pp. 297-357; (b) T. P. Simonova, V. D. Nefedov, M. A. Toropova, and N. F. Kirillov, Uspekhi Khimii, 1992, 61, 1061; (c) Y. Kikugawa, Rev. Heteroatom Chem., 1996, 15, 263; (d) D. Bogdal, Heterocycles, 2000, 53, 2679; CrossRef (e) D. E. Falvey, ‘Nitrenium ions in Reactive Intermediate Chemistry’ , ed. by R. A. Moss, M. S. Platz, and M. Jones Jr., Wiley-Interscience, A John Wiley & Sons, Inc., 2004, pp. 593-650; (f) G. I. Borodkin and V. G. Shubin, Russian J. Org. Chem., 2005, 41, 473. CrossRef
2. G. Gassman, Acc. Chem. Res., 1970, 3, 26. CrossRef
3. (a) P. G. Gassman, F. Hoyda, and J. Dygos, J. Am. Chem. Soc., 1968, 90, 2716; CrossRef (b) P. G. Gassman and J. Dygos, Tetrahedron Lett., 1970, 4745. CrossRef
4. M. Kawase and Y. Kikugawa, Chem. Pharm. Bull., 1981, 29, 1615. CrossRef
5. P. G. Gassman, G. A. Campbell, and R. C. Frederick, J. Am. Chem. Soc., 1972, 94, 3884. CrossRef
6. G. A. Olah, P. Ramaiah, Q. Wang, and G. K. S. Prakash, J. Org. Chem., 1993, 58, 6900. CrossRef
7. (a) H. Takeuchi and K. Takano, J. Chem. Soc., Chem. Commun., 1983, 447; CrossRef (b) H. Takeuchi and K. Takano, J. Chem. Soc., Perkin Trans. 1, 1986, 611. CrossRef
8. (a) R. A. Abramovitch, P. Chinnasamy, K. Evertz, and G. Huttner, J. Chem. Soc., Chem. Commun., 1989, 3; CrossRef (b) R. A. Abramovitch and X. Ye, J. Org. Chem., 1999, 64, 5904. CrossRef
9. A. M. Almerico, G. Cirrincione, P. Diana, S. Grimaudo, G. Dattolo, and E. Aiello, Heterocycles, 1994, 37, 1549. CrossRef
10. R. A. Abramovitch, J. Miller, and A. J. C. Souza, Tetrahedron Lett., 2003, 44, 6965. CrossRef
11. T. Ohta, S. Miyake, and K. Shudo, Tetrahedron Lett., 1985, 26, 5811. CrossRef
12. A. Ohwada, S. Nara, T. Sakamoto, and Y. Kikugawa, J. Chem. Soc., Perkin Trans. 1, 2001, 3064. CrossRef
13. A. Ohwada, H. Li, T. Sakamoto, and Y. Kikugawa, Heterocycles, 1997, 46, 225. CrossRef
14. Y. Kikugawa, Y. Aoki, and T. Sakamoto, J. Org. Chem., 2001, 66, 8612. CrossRef
15. Y. Kikugawa, Y. Miyake, and M. Kawase, Chem. Pharm. Bull., 1981, 29, 1231. CrossRef
16. (a) A. H. Winter, S. I. Thomas, A. C. Kung, and D. E. Falvey, Org. Lett., 2004, 6, 4671; CrossRef (b) S. I. Thomas and D. E. Falvey, J. Org. Chem., 2007, 72, 4626. CrossRef
17. P. G. Gassman and G. A. Campbell, Chem. Commun., 1971, 1437. CrossRef
18. T. Sakamoto, I. Hosoda, and Y. Kikugawa, J. Heterocycl. Chem., 1988, 25, 1279. CrossRef
19. (a) T. J. McCord, J. L. Kreps, J. N. Hubbard, and A. L. Davis, J. Heterocycl. Chem., 1969, 6, 937; CrossRef (b) T. J. McCord, D. H. Kelly, J. A. Rabon, D. C. Foyt, and A. L. Davis, J. Heterocycl. Chem., 1972, 9, 119; CrossRef (c) T. J. McCord, S. F. Kelley, J. A. Rabon, L. D. Gage, L. L. Maples, and A. L. Davis, J. Heterocycl. Chem., 1976, 13, 1091; CrossRef (d) T. J. McCord, C. P. Crawford, J. A. Rabon, L. D. Gage, J. M. Winter, and A. L. Davis, J. Heterocycl. Chem., 1982, 19, 401. CrossRef
20. Y. Konda, M. Onda, A. Hirano, and S. Omura, Chem. Pharm. Bull., 1980, 28, 2987. CrossRef
21. N. R. Ayyangar, U. R. Kalkote, and P. V. Nikrad, Tetrahedron Lett., 1982, 23, 1099. CrossRef
22. M. Hudlicky, Org. Reactions, 1988, 35, 513.
23. Y. Kikugawa, K. Matsumoto, K. Mitsui, and T. Sakamoto, J. Chem. Soc., Chem. Commun., 1992, 921. CrossRef
24. Y. Kikugawa and M. Shimada, J. Chem. Soc., Chem. Commun., 1989, 1450. CrossRef
25. Y. Kikugawa and K. Mitsui, Chem. Lett., 1993, 1369. CrossRef
26. N. Itoh, T. Sakamoto, E. Miyazawa, and Y. Kikugawa, J. Org. Chem., 2002, 67, 7424. CrossRef
27. (a) A. Correa, I. Tellitu, E. Domínguez, and R. SanMartín, J. Org. Chem., 2006, 71, 3501; CrossRef (b) A. Correa, I. Tellitu, E. Domínguez, and R. SanMartín, Tetrahedron, 2006, 62, 11100. CrossRef
28. A. Correa, I. Tellitu, E. Domínguez, and R. SanMartín, Org. Lett., 2006, 8, 4811. CrossRef
29. S. Serena, I. Tellitu, E. Domínguez, I. Moreno, and R. SanMartín, Tetrahedron Lett., 2003, 44, 3483. CrossRef
30. (a) A. Correa, I. Tellitu, E. Domínguez, and R. SanMartín, J. Org. Chem., 2006, 71, 8316; CrossRef (b) I. Tellitu, A. Urrejola, S. Serna, I. Moreno, M. T. Herrrero, E. Domínguez, R. SanMartín, and A. Crrea, Eur. J. Org. Chem., 2007, 437. CrossRef
31. (a) S. Serna, I. Tellitu, E. Domínguez, I. Moreno, and R. SanMartín, Org. Lett., 2005, 7, 3073; CrossRef (b) I. Tellitu, S. Serna, M. T. Herrrero, I. Moreno, E. Domínguez, and R. SanMartín, J. Org. Chem., 2007, 72, 1526. CrossRef
32. S. A. Glover, A. Goosen, C. W. McCleland, and J. L. Schoonraad, J. Chem. Soc., Perkin Trans. 1, 1984, 2255. CrossRef
33. (a) Y. Kikugawa and M. Kawase, J. Am. Chem. Soc., 1984, 106, 5728; CrossRef (b) M. Kawase, T. Kitamura, and Y. Kikugawa, J. Org. Chem., 1989, 54, 3394; CrossRef (c) Y. Kikugawa and M. Shimada, Chem. Lett., 1987, 1771. CrossRef
34. Y. Kikugawa, M. Shimada, and K. Matsumoto, Heterocycles, 1994, 37, 293. CrossRef
35. I. Fleming, R. C. Moses, M. Tercel, and J. Ziv, J. Chem. Soc., Perkin Trans. 1, 1991, 617. CrossRef
36. (a) Y. Kikugawa, M. Kawase, Y. Miyake, T. Sakamoto, and M. Shimada, Tetraherdron Lett., 1988, 29, 4297; CrossRef (b) M. Kawase, Y. Miyake, T. Sakamoto, M. Shimada, and Y. Kikugawa, Tetrahedron, 1989, 45, 1653. CrossRef
37. U. Tilstam, M. Harre, T. Heckrodt, and H. Weinmann, Tetrahedron Lett., 2001, 42, 5385. CrossRef
38. Y. Kikugawa and M. Kawase, Chem. Lett., 1990, 581. CrossRef
39. (a) A. G. Romero, W. H. Darlington, E. J. Jacobsen, and J. W. Mickelson, Tetrahedron Lett., 1996, 37, 2361; CrossRef (b) A. G. Romero, W. H. Darlington, and M. W. McMillan, J. Org. Chem., 1997, 62, 6582. CrossRef
40. D. J. Wardrop and A. Basak, Org. Lett., 2001, 3, 1053. CrossRef
41. M. T. Herrero, I. Tellitu, E. Domínguez, S. Hernández, I. Moreno, and R. SanMartín, Tetrahedron, 2002, 58, 8581. CrossRef
42. (a) M. T. Herrero, I. Tellitu, E. Domínguez, S. Hernández, I. Moreno, and R. SanMartín, Tetrahedron Lett., 2002, 43, 8273; CrossRef (b) A. Correa, M. T. Herrero, I. Tellitu, E. Domínguez, I. Moreno, and R. SanMartín, Tetrahedron, 2003, 59, 7103. CrossRef
43. A. Correa, I. Tellitu, E. Domínguez, I. Moreno, and R. SanMartín, J. Org. Chem., 2005, 70, 2256. CrossRef
44. C.-Y. Chang and T.-K. Yang, Tetrahedron: Asymmetry, 2003, 14, 2081. CrossRef
45. A. Glover, C. A. Rowbottom, A. P. Scott, and J. L. Schoonraad, Tetrahedron, 1990, 46, 7247. CrossRef
46. (a) A. J. Birch, Quart. Rev., Chem. Soc., 1950, 4, 69; CrossRef (b) P. W. Rabideau and Z. Marcinow, Org. Reactions, 1992, 42, 1.
47. S. Nagumo, A. Nishida, C. Yamazaki, K. Murashige, and N. Kawahara, Tetrahedron Lett., 1998, 39, 4493. CrossRef
48. D. J. Wardrop and W. Zhang, Org. Lett., 2001, 3, 2353. CrossRef
49. D. J. Wardrop, W. Zhang, and C. L. Landrie, Tetrahedron Lett., 2004, 45, 4229. CrossRef
50. D. J. Wardrop, M. S. Burge, W. Zhang, and J. A. Ortíz, Tetrahedron Lett., 2003, 44, 2587. CrossRef
51. M. Aratani, L. V. Dunkerton, T. Fukuyama, Y. Kishi, H. Kakoi, S. Sugiura, and S. Inoue, J. Org. Chem., 1975, 40, 2009. CrossRef
52. D. J. Wardrop, C. L. Landrie, and J. A. Ortíz, Synlett, 2003, 1352. CrossRef
53. D. J. Wardrop and M. S. Burge, Chem. Commun., 2004, 1230. CrossRef
54. D. J. Wardrop and M. S. Burge, J. Org. Chem., 2005, 70, 10271. CrossRef
55. E. Miyazawa, T. Sakamoto, and Y. Kikugawa, J. Org. Chem., 2003, 68, 5429. CrossRef
56. E. Miyazawa, T. Sakamoto, and Y. Kikugawa, Heterocycles, 2003, 59, 149. CrossRef
57. Y. Amano and S. Nishiyama, Tetrahedron Lett., 2006, 47, 6505. CrossRef
58. Y. Misu and H. Togo, Org. Biomol. Chem., 2003, 1, 1342. CrossRef
59. A. Moroda and H. Togo, Synthesis, 2008, 1257. CrossRef
60. S. A. Glover and A. P. Scott, Tetrahedron, 1989, 45, 1763. CrossRef
61. D.-T. V. Clemente, A. M. Lobo, S. Prabhakar, and M. J. Marcelo-Curto, Tetrahedron Lett., 1994, 35, 2043. CrossRef
62. (a) I. Zaltsgendler, Y. Leblanc, and M. A. Bernstein, Tetrahedron Lett., 1993, 34, 2441; CrossRef (b) H. Mitchell and Y. Leblanc, J. Org. Chem., 1994, 59, 682; CrossRef (c) Y. Leblanc and N. Boudreault, J. Org. Chem., 1995, 60, 4268; CrossRef (d) C. Dufresne, Y. Leblanc, C. Berthelette, and C. McCooeye, Synth. Commun., 1997, 27, 3613; CrossRef (e) J. S. Yadav, B. V. S. Reddy, G. M. Kumar, and K. C. Madan, Synlett, 2001, 1781; CrossRef (f) J. S. Yadav, B. V. S. Reddy, G. Veerendhar, R. S. Rao, and K. Nagaiah, Chem. Lett., 2002, 318. CrossRef
63. J. V. Prata, D.-T. S. Clemente, S. Prabhakar, A. M. Lobo, I. Mourato, and P. S. Branco, J. Chem. Soc., Perkin Trans. 1, 2002, 513. CrossRef
64. Y. Kikugawa, A. Nagashima, T. Sakamoto, E. Miyazawa, and M. Shiiya, J. Org. Chem., 2003, 68, 6739. CrossRef
65. M. Shiiya, T. Sakamoto, E. Miyazawa, and Y. Kikugawa. Presented in part at the 123th Pharmaceutical Society of Japan, Nagasaki, Japan, March 2003. Abstracts of Papers, 2, p. 10.
66. Y. Kikugawa, T. Kitamura, and M. Kawase, J. Chem. Soc., Chem. Commun., 1989, 525. CrossRef
67. (a) P. G. Gassman and G. A. Campbell, J. Chem. Soc., Chem. Commun., 1970, 427; (b) E. J. Corey, S. Barcza, and G. Klotmann, J. Am. Chem. Soc., 1969, 91, 4782. CrossRef
68. M. Tsukamoto, K. Murata, T. Sakamoto, S. Saito, and Y. Kikugawa, Heterocycles, 2008, 75, 1133. CrossRef
69. K. Murata, M. Tsukamoto, T. Sakamoto, S. Saito, and Y. Kikugawa, Synthesis, 2008, 32. CrossRef
70. In the intramolecular cyclization of an N-acylnitrenium ion and the olefin fragment in a molecule, Tellitu and Domínguez reported that the created carbocationic species are stabilized by the formation of aziridinium ion intermediates. See refs 29, 30a and 31a.
71. A. Nagashima, T. Sakamoto, and Y. Kikugawa, Heterocycles, 2007, 74, 273. CrossRef