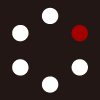
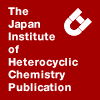
HETEROCYCLES
An International Journal for Reviews and Communications in Heterocyclic ChemistryWeb Edition ISSN: 1881-0942
Published online by The Japan Institute of Heterocyclic Chemistry
e-Journal
Full Text HTML
Received, 30th September, 2008, Accepted, 21st November, 2008, Published online, 28th November, 2008.
DOI: 10.3987/REV-08-SR(D)3
■ Synthesis of 1α,25-Dihydroxy-2β-(3-hydroxypropoxy)vitamin D3 (Eldecalcitol) and Related Compounds by the Trost Convergent Methodology
Noboru Kubodera* and Susumi Hatakeyama
Chugai Pharmaceutical Company, Ltd., 2-1-1, Nihonbashi-Muromachi, Chuo-ku, Tokyo 103-8324, Japan
Abstract
Using Trost convergent methodology, the synthesis of 1α,25-dihydroxy-2β-(3-hydroxypropoxy)vitamin D3 (eldecalcitol) for the improved and industrial scale production and related putative metabolites of eldecalcitol is summarized. In addition, A-ring diastereomers at the 1- and 3-positions of eldecalcitol are described. The synthesis centers on a key palladium-catalyzed alkylative cyclization and coupling of ene-ynes that constitute the A-ring fragment to bromomethylenes comprising the C/D-ring fragment which affords the requisite triene framework of vitamin D3 analogs.INTRODUCTION
Active vitamin D3, 1α,25-dihydroxyvitamin D3 (calcitriol, 1), and its synthetic prodrug, 1α-hydroxyvitamin D3 (alfacalcidol, 2), are well recognized as potent regulators of cell proliferation and differentiation in addition to possessing regulatory effects on calcium and phosphorus metabolism.1 Various analogs of calcitriol (1) have been synthesized to separate differentiation-induction and antiproliferation activities from calcemic activity with the aim of obtaining useful analogs for the medical treatment of psoriasis, secondary hyperparathyroidism, cancer, etc., without risk of hypercalcemia.2 There is also intense interest in obtaining analogs more potent than calcitriol (1) and alfacalcidol (2) in regulating calcium and phosphorus metabolism with the objective of treating bone disease such as osteoporosis. 1α,25-Dihydroxy-2β-(3-hydroxypropoxy)vitamin D3 (eldecalcitol, 3, developing code: ED-71), which possesses a hydroxypropoxy substituent at the 2β-position of the A-ring of calcitriol (1), is such an analog that shows more potent effects on bone therapy.3-8 Phase III clinical studies of eldecalcitol (3) as a promising candidate for the treatment of osteoporosis and bone fracture prevention are now being successfully conducted in Japan and will be completed by the end of 2008 after 3 years of medication of osteoporotic patients (Figure 1).9
Synthetic studies on related compounds such as putative metabolites for structural elucidation of actual metabolites obtained in pharmacokinetic and metabolic studies, biologically interesting diastereomers for investigation of detailed mode of action, etc., in addition to improvement of practical synthesis for industrial scale production are generally indispensable to the development of new medicines.10 Since during the development of eldecalcitol (3) as an anti-osteoporotic agent,11 various related compounds were also necessary to be synthesized, we adopted the Trost convergent methodology to construct triene framework of 3 and related analogs as a common key reaction. The Trost reaction, which involves tandem palladium-catalyzed alkylative cyclization of ene-ynes as the A-ring fragment with bromomethylenes as C/D-ring fragment seemed to be versatile and applicable to our synthetic strategy (Scheme 1).12-13 In this review, we describe an improved practical synthesis of eldecalcitol (3) and the synthesis of related analogs of 3, A-ring diastereomers at the 1- and 3-positions and putative metabolites, which focuses on the Trost convergent coupling reaction. The detailed biological background of each targeted analog of 3 is also described.
AN IMPROVED PRACTICAL SYNTHESIS OF ELDECALCITOL (3)
Considering the potential clinical applications of eldecalcitol (3) as a useful drug in the near future, we investigated a practical synthesis of 3 for industrial scale production. Eldecalcitol (3) was initially synthesized in a linear manner in which the 1,2α-epoxide 6, prepared from lithocholic acid (4) via 25-hydroxycholesterol (5),14 served as a key intermediate for the introduction of the characteristic hydroxypropoxy substituent at the 2β-position (Scheme 2).3 The 27-step linear sequence, however, was suboptimal due to its lengthiness and low overall yield. We, therefore, sought out to develop a more efficient and practical route that involves a convergent approach for the preparation of eldecalcitol (3) based on the Trost coupling reaction.
Our synthesis of eldecalcitol (3) was envisioned using the coupling reaction of A-ring fragment 7 derived from C2-symmetrical epoxide 8 with C/D-ring fragment 9 which can be obtained from 25-hydroxyvitamin D3 (25OHD3, 10) (Scheme 3).
The required A-ring fragment 7 for the synthesis of eldecalcitol (3) was synthesized based on the methodology that has been previously established.15 Thus, cleavage of the known C2-symmetrical epoxide 816 with 1,3-propanediol (HO(CH2)3OH) in the presence of potassium tert-butoxide (t-BuOK) gave diol 11 in 86% yield. After protection of the primary hydroxyl group to give pivalate 12 in 88% yield, cleavage of the benzyl ether moiety in 12 and subsequent protection of the resulting 1,2-diol as the acetonide gave alcohol 13 in 87% overall yield. Swern oxidation of 13 and subsequent Grignard reaction of the resulting aldehyde with vinylmagnesium bromide (CH2=CHMgBr) followed by pivaloylation of the resulting alcohol afforded the dipivalate 14 as an epimeric mixture (R/S = 3/2). Without separation of the epimeric mixture, the acetonide moiety in 14 was cleaved quantitatively to give diol 15. Exposure of 15 to Mitsunobu conditions17 afforded epoxide 16 in 77% yield. The acetylene unit was successfully installed by the regioselective epoxide-opening of 16 with lithium trimethylsilylacetylide (LiC CTMS) in the presence of boron trifluoride diethyl etherate (BF3-OEt2) at –78 °C to provide ene-yne 7 as the A-ring fragment of eldecalcitol (3) in 36% yield after protecting group exchange from pivalate to tert-butyldimethylsilyl (TBS) ether. The accompanied (S)-isomer 17, which consists of the requisite
stereochemistry to obtain 1-epi-eldecalcitol (21), was separated in 24% yield by simple column
chromatography. Next, we performed the synthesis of C/D-ring fragment 9 from readily and commercially available 25OHD3 (10). 25OHD3 (10) was protected as the bis-triethylsilyl (TES) ether using triethylsily trifluoromethanesulfonate (TESOTf), and was then converted to alcohol 18 by ozonolysis and treatment with sodium borohydride (NaBH4) (72% yield from 10). The hydroxyl moiety in 18 was oxidized to ketone 19 with tetrapropylammonium perruthenate (TPAP) and N-methylmolpholine N-oxide (NMO) in 99% yield. Wittig reaction of 19 with (bromomethylene)triphenylphosphonium bromide (Ph3P+CH2Br/Br-) and sodium hexamethyldisilazide (NaHMDS) gave rise to C/D-ring fragment bromomethylene 9 (R=TES) in 38% yield. With A-ring fragment 7 and C/D-ring fragment 9 in hand, we next investigated the Trost coupling reaction. Upon treatment of 7 and 9 with triethylamine (Et3N), triphenylphosphine (PPh3) and tris(di-benzylideneacetone)dipalladium-chloroform [(dba)3Pd2-CHCl3] in boiling toluene, the desired coupled product 20 was obtained in 26% yield together with recovered 7 (45%) and 9 (56%). Deprotection of the silyl moiety in 20 with tetrabutylammonium fluoride (TBAF) afforded eldecalcitol (3) in 60% yield (Scheme 4).
Although the overall yield of eldecalcitol (3) by the Trost convergent synthesis was better than the previous linear approach, significant improvements were still demanded for a practical production of 3 and further investigation are ongoing.18
SYNTHESIS OF DIASTEREOMERS AT THE 1- AND 3-POSITIONS OF ELDECALCITOL (3)
To explore the biological mode-of-action of eldecalcitol (3) toward bone and related tissues such as parathyroid glands, intestines, and kidneys, diastereomers at the 1- and 3-positions of the A-ring seemed to be very helpful with inherent reasons described below. We, therefore, synthesized 1-epi-eldelacitol (21), 3-epi-eldecalcitol (22), and 1,3-diepi-eldecalcitol (23) (Figure 2).19
SYNTHESIS OF 1-EPI-ELDECALCITOL (21)
In Japan, calcitriol (1) and alfacalcidol (2) have been widely used for the treatment of osteoporosis for more than 25 years.20 Calcitriol (1) and alfacalcidol (2) have been recognized as very safe medicines showing mild or moderate increase in bone mineral density (BMD) in osteoporotic patients. In the US and Europe, bisphosphonates such as sodium alendronate, sodium risedronate and sodium ibandronate, are mainly prescribed for treating osteoporosis because of strong increment of BMD.21 Therefore, there has been strong interest in obtaining active vitamin D3 analogs more potent than calcitriol (1)/alfacalcidol (2) or comparable to bisphosphonates in increasing BMD and preventing bone fracture. Eldecalcitol (3) has been shown to be more effective than calcitrol (1) and alfacalcidol (2) in increasing BMD and mechanical bone strength in ovariectomized model rats.7,22 The detailed mode of action of the enhanced activity of eldecalcitol (3) beyond calcitriol (1) and alfacalcidol (2) toward bone remains to be clarified. The long duration of 3 in the blood stream arises from its strong affinity for vitamin D binding protein (DBP) (2-fold ~ 4-fold v. 1) and might explain, in part, the enhanced biological effects of 3.6 We, therefore, were highly interested in developing an active vitamin D3 analog with strong affinity for DBP. It was reported that the epimerization of calcitriol (1) at the 1-position of the A-ring remarkably enhances the affinity for DBP. Norman and co-workers reported that 1-epi-calcitriol shows a 65.7-fold increase in affinity for DBP as compared to 1.23 These findings stimulated our interest in the biological profile of epimerized eldecalcitol (3), particularly at the 1-position. Thus our attention turned to 1-epi-eldecalcitol (21) and studying its affects on DBP affinity and on bone.
As described above, when ene-yne 7 was prepared from epimeric epoxide 16 as the (R)-isomer, the separable (S)-isomer 17 was accompanied as a by-product, which was used to obtain 1-epi-eldecalcitol (21). Upon treatment of excess bromomethylene 9 (R=TES) and ene-yne 17 (R=TES) in the presence of tetrakis(triphenylphosphine)palladium (0) [Pd(PPh3)4] and Et3N in boiling toluene, the coupled product 24 was obtained as an inseparable mixture with recovered 9. The mixture was desilylated using 47% hydrofluoric acid (HF) in acetonitrile (MeCN) and purified to afford 1-epi-eldecalcitol (21) in 37% yield from 17 (Scheme 5).
As anticipated, 1-epi-eldecalcitol (21) showed enhanced affinity for DBP (1.6-fold v. eldecalcitol) and further in vivo biological studies with 21 including effects on bone are currently under investigation.24,25
SYNTHESIS OF 3-EPI-ELDECALCITOL (22)
Recently, it was reported that the epimerization of calcitriol (1) at the 3-position of the A-ring plays a major role in parathyroid hormone (PTH) synthesis and secretion. Epimerized 3-epi-calcitriol shows equipotent and prolonged activities in comparison with 1 at suppressing PTH secretion.26,27 During our clinical development of eldecalcitol (3), serum PTH in osteoporotic patients, however, did not change significantly upon treatment with 3.9 We assumed that a bulky hydroxylpropoxy substituent at the 2-position of the A-ring would interfere with epimerization of eldecalcitol (3) at the adjacent and sterically hindered 3-position leading to the absence of epimerized 3-epi-eldecalcitol (22) in parathyroid glands. This could explain why eldecalcitol (3) showed weak potency in PTH suppression during clinical studies. We, therefore, were interested in the synthesis and biological evaluation of 3-epi-eldecalcitol (22).
The synthesis of A-ring fragment 33 for 3-epi-eldecalcitol (22) began with inversion of the C3 configuration of alcohol 12. Reaction of 12 with p-nitrobenzoic acid (p-(NO2)PhCO2H) in the presence of diethyl azodicarboxylate (DEAD) and PPh3 gave p-nitrobenzoate 25 in 84% yield.28 Treatment of 25 with sodium hydrogen carbonate (NaHCO3) in methanol (MeOH) allowed selective methanolysis of the p-nitrobenzoate group to give inverted alcohol 26 in 86% yield. After hydrogenolysis of the benzyl ether functionality in 26, the resulting diol was protected as its acetonide to afford 27 in 88% yield. Swern oxidation of 27 followed by Grignard reaction of the resulting aldehyde with CH2=CHMgBr produced alcohol 28 as an epimeric mixture (S:R = 3:2) in 66% yield. To separate this epimeric mixture, 28 was subjected to lipase-catalyzed acetylation using vinyl acetate (CH2=CHOAc) and Novozyme in t-butyl methyl ether (t-BuOMe).29 The (R)-isomer preferentially underwent acetylation to give the acetate 29 and S-28 (R:S = 1:20) in 40% and 57% yields, respectively. Acidic hydrolysis of 29 gave diol 30 in 90% yield, which upon Mitsunobu reaction using DEAD and PPh3 in boiling toluene afforded epoxide 31 in 75% yield. Reaction of 31 with LiC CTMS in the presence of BF3-OEt2 at –78 °C followed by saponification provided ene-yne 32 in 65% yield.30 Protection of 32 as its TES ether produced A-ring fragment 33 quantitatively. Having secured A-ring fragment 33, we performed its coupling with C/D-ring fragment 9 using Trost methodology. Thus, A-ring fragment 33 was allowed to react with C/D-ring fragment 9 (R=H) in the presence of Pd(Ph3P)4 and Et3N in boiling toluene to give coupling product 34 which was desilylated with ammonium fluoride (NH4F) in boiling MeOH to produce 3-epi-eldecalcitol (22) in 46% yield from 33 (Scheme 6).
3-Epi-eldecalcitol (22) showed only slight inhibition of PTH secretion in bovine parathyroid cells compared to eldecalcitol (3). Eldecalcitol (3) and 3-epi-eldecalcitol (22) might be inherently weak agents toward PTH suppression, which might be a beneficial characteristic of 3 for treating osteoporotic patients, although further studies with 3 will be necessary.31,32
SYNTHESIS OF 1,3-DIEPI-ELDECALCITOL (23)
To further explore structure-activity-relationships between eldecalcitol (3) and related analogs, we focused significant attention to the diastereomer of 3 at both the 1- and 3-positions of the A-ring. We proceeded with the synthesis of structurally and biologically relevant 1,3-diepi-eldecalcitol (23), which represents the final structural member that completes the set all possible A-ring diastereomers at the 1- and 3-positions of eldecalcitol (3). This analog, in combination with others, is anticipated to enhance our understanding of the mode-of-action of medicinally important 3. The synthesis of A-ring fragment 39 commenced from (S)-alcohol 28 which was obtained in our previous lipase-catalyzed acetylation of 28 as the unreacted (S)-isomer.31 (S)-Alcohol 28 possesses the requisite stereochemistry at positions 1, 2, and 3 of the A-ring that comprises 23. Acetylation of 28 gave acetate 35 in 80% yield, from which diol 36 was obtained in 89% yield after deprotection of the acetonide moiety using 60% acetic acid (AcOH). Mitsunobu reaction of 36 with DEAD and PPh3 in boiling toluene afforded epoxide 37 in 68% yield. Reaction of 37 with LiC CTMS in the presence of BF3-OEt2 at -78 °C gave ene-yne 38 in 75% yield. Conversion of 38 to A-ring fragment 39 was achieved by saponification with 10 M sodium hydroxide (NaOH) followed by subsequent protection of the hydroxyl groups as their TES ether in 65% overall yield. With A-ring fragment 39 in hand, the key coupling step to C/D-ring fragment 9 using the Trost methodology was investigated. Thus, 39 was coupled with 9 (R=H) in the presence of Pd(PPh3)4 and Et3N in boiling toluene to produce desired coupling product 40 in 80% yield. Desilylation of 40 with 46% HF in MeCN at rt afforded 1,3-diepi-eldecalcitol (23) in 60% yield (Scheme 7).
The detailed biological properties of 1,3-diepi-eldecalcitol (23) in comparison with eldecalcitol (3), 1-epi-eldecalcitol (21) and 3-epi-eldecalcitol (22) are currently under investigation.33
SYNTHESIS OF PUTATIVE METABOLITES OF ELDECALCITOL (3)
It is known that calcitriol (1) is hydroxylated at the 24-position of the side chain as a first step in its metabolism to produce 24-hydroxylated calcitriol.34-39 On the assumption that hydroxylation pathway of eldecalcitol (3) and 1 would be similar, we undertook the synthesis of 24-hydroxylated eldecalcitol in 24(R) and 24(S) forms (41R and 41S).15 In the case of calcitriol (1), 24-hydroxylated calcitriol is further hydroxylated at the 23- and 26-positions of the side chain and oxidized to keto-alcohol, lactone (calcitriol lactone), or carboxylic acid (calcitroic acid).34-39 Since eldecalcitol (3) has a substituent at the 2β-position of the A-ring, it is recognized that the metabolic pathway of 3 might be more
complicated than calcitriol (1) because of metabolism at the 2β-position substituent in addition to the inherent metabolism of the side chain. In fact, preliminary metabolic studies on eldecalcitol (3) revealed the presence of 24-hydroxylated eldecalcitol (41R and 41S) as metabolites of 3 which were
also accompanied by several other metabolic associates that may be derived from metabolism of both
the hydroxypropoxy substituent of the 2β-position and a combination of metabolism between the side chain and 2β-position. In order to assist in the confirmation and structure elucidation of putative metabolic analogs of eldecalcitol (3), the synthesis of 2β-truncated eldecalcitol (42) and 24-hydroxylated and 2β-truncated eldecalcitol (43R and 43S) was pursued (Scheme 8).
SYNTHESIS OF 24-HYDROXYLATED ELDECALCITOL (41R and 41S)
The synthesis of C/D-ring fragments in both 24(R)- and 24(S)-hydroxylated series, respectively, was performed. Inhoffen-Lythgoe diol (44), prepared from vitamin D2 by ozonolysis,40 was converted to sulfone 46 via 45 by known methodology.41,42 Sulfone 46 was alkylated independently with (R)- and (S)-2,3-dihydroxy-3-methylbutyl p-toluenesulfonate 47R and 47S43,44 to afford alcohols 48R in 71% yield and 48S in 28% yield, respectively. The (R)- and (S)-alcohols 48R and 48S were converted to C/D-ring fragments 55R and 55S as follows:
1. Desulfurization of 48R and 48S with sodium amalgam (Na-Hg) produced 49R and 49S in 97% and 68% yields, respectively.
2. Acetonide formation of 49R and 49S with 2,2-dimethoxypropane (Me2C(OMe)2) and subsequent acetylation giving 50R and 50S in 83% and 64% yields, respectively.
3. Deprotection of the acetonide moiety in 50R and 50S with iodine (I2) in MeOH produced 51R and 51S in 61% and 83% yields, respectively.
4. Silylation of 51R and 51S with TBSOTf gave 52R and 52S in 92% and 91% yields, respectively.
5. Reductive cleavage of the acetyl groups in 52R and 52S with lithium aluminum hydride (LiAlH4) afforded 53R and 53S in 96% and 100% yields, respectively.
6. Oxidation of the hydroxyl groups in 53R and 53S with NMO and TPAP produced 54R and 54S in 96% and 100% yields, respectively.
7. Wittig reaction of 54R and 54S with Ph3P+CH2Br/Br- in the presence of NaHMDS generated 55R and 55S in 49% and 57% yields, respectively.
Using Trost methodology, 24-hydroxylated C/D-ring fragments 55R and 55S were coupled with A-ring fragment 7 which produced coupled products 56R and 56S in 36% and 34% yields, respectively. Deprotection with TBAF gave 24(R)-hydroxylated eldecalcitol (41R) and 24(S)-hydroxylated eldecalcitol (41S) in 60% and 66% yields, respectively (Scheme 9).
When eldecalcitol (3) was orally administered to rats, 24-hydroxylated eldecalcitol (41R and 41S) were found in rat plasma as actual metabolites of 3, for which the synthesized 41R and 41S were conveniently used as authentic samples for structure elucidation.15
SYNTHESIS OF 2β-TRUNCATED ELDECALCITOL (42)
Next, the synthesis of the A-ring fragment 66 for the preparation of 2β-truncated eldecalcitol (42) was undertaken. Diol 57, obtained from D-mannitol,45 was protected as pivalate 58 in 72% yield. Pivalate 58 was subjected to hydrolysis with 1N hydrochloric acid (HCl) to give diol 59 (50% yield) accompanied by tetraol 60 (34% yield) as well as recovered starting material 58 (16% yield), which were easily separated by silica gel column chromatography. Diol 59 was converted to orthoester 61 (88% yield) with triethyl orthoformate (HC(OEt)3) and p-toluenesulfonic acid monohydrate (TsOH). Upon treatment of orthoester 61 with boiling acetic anhydride (Ac2O), terminal olefin formation took place cleanly to produce olefin 62 in 93% yield.46-49 Deketalization of 62 afforded diol 63 in 93% yield. Subsequent
Mitsunobu reaction of 63 with diisopropyl azodicarboxylate (DIAD) and Ph3P in dioxane gave epoxide 64 in 74% yield. Treatment of epoxide 64 with LiC CTMS in the presence of BF3-OEt2 provided 1,7-ene-yne 65 in 85% yield. Then, 2β-truncated A-ring fragment 66 was obtained from 65 by hydrolysis and subsequent TES ether formation in 93% yield. With A-ring fragment 66 in hand, the coupling reaction with C/D-ring fragment 9 (R=TMS) following the Trost methodology gave rise to 67 in 17% yield, which was deprotected using TBAF to afford 2β-truncated eldecalcitol (42) in 50% yield (Scheme 10).
The synthesized 2β-truncated eldecalcitol (42) is currently in use as an authentic reference sample for metabolic studies of eldecalcitol (3).50
SYNTHESIS OF 24-HYDROXYLATED AND 2β-TRUNCATED ELDECALCITOL (43R and 43S)
2β-Truncated A-ring fragment 66 and 24-hydroxylated C/D-ring fragments 55R and 55S consist of the requisite components for the synthesis of 24-hydroxylated and 2β-truncated eldecalcitol (43R and 43S). The coupling reaction between 55R or 55S and 66 under Trost conditions produced coupled products 68R and 68S in 23% and 27% yields, respectively. Deprotection using TBAF afforded 24(R)-hydroxylated and 2β-truncated eldecalcitol (43R) and 24(S)-hydroxylated and 2β-truncated eldecalcitol (43S) in 50% and 70% yields, respectively (Scheme 11).
The synthesized putative metabolites, 43R and 43S, are currently in use as authentic reference samples for metabolic studies of eldecalcitol (3).50
CONCLUSION
Based on the Trost coupling methodology involving A-ring fragments 7, 17, 33, 39, and 66 and C/D-ring fragments 9, 55R, and 55S, the successful total synthesis of eldecalcitol (3) and related compounds, 1-epi-eldecalcitol (21), 3-epi-eldecalcitol (22), 1,3-diepi-eldecalcitol (23), 24-hydroxylated eldecalcitol (41R and 41S), 2β-truncated eldecalcitol (42), and 24-hydroxylated and 2β-truncated eldecalcitol (43R and 43S) has been achieved. These analogs play important roles as indispensable structural entities during the development of eldecalcitol (3) as an anti-osteoporotic agent.
ACKNOWLEDGEMENTS
The authors thank all coworkers of Chugai Pharmaceutical Co., Ltd, and Graduate School of Biomedical Sciences, Nagasaki University for their efforts and cooperation. We are grateful to Professor David Horne of Department of Molecular Medicine, City of Hope for helpful suggestions and reading of the manuscript.
References
1. R. Bouillon, W. H. Okamura, and A. Norman, Endocrine Rev., 1995, 16, 200.
2. G. H. Posner and M. Kahraman, ‘Overview: Rational Design of 1α,25-Dihydroxyvitamin D3 Analogs (Deltanoids)’ Vitamin D Second Edition, ed. by D. Feldman, J. W. Pike, and F. H. Glorieux, Elsevier Academic Press, Burlington, 2005, pp. 1405-1422.
3. K. Miyamoto, E. Murayama, K. Ochi, H. Watanabe, and N. Kubodera, Chem. Pharm. Bull., 1993, 41, 1111.
4. Y. Ono, H. Watanabe, A. Shiraishi, S. Takeda, Y. Higuchi, K. Sato, N. Tsugawa, T. Okano, T. Kobayashi, and N. Kubodera, Chem. Pharm. Bull., 1997, 45, 1626.
5. Y. Ono, A. Kawase, H. Watanabe, A. Shiraishi, S. Takeda, Y. Higuchi, K. Sato, T. Yamauchi, T. Mikami, M. Kato, N. Tsugawa, T. Okano, and N. Kubodera, Bioorg. Med. Chem., 1998, 6, 2517. CrossRef
6. T. Okano, N. Tsugawa, S. Masuda, A. Takeuchi, T. Kobayashi, Y. Takita, and Y. Nishii, Biochem. Biophys. Res. Commun., 1989, 163, 1444. CrossRef
7. T. Kobayashi, T. Okano, N. Tsugawa, M. Murano, S. Masuda, A. Takeuchi, K. Sato, and Y. Nishii, Bioorg. Med. Chem. Lett., 1993, 3, 1815. CrossRef
8. N. Kubodera, N. Tsuji, Y. Uchiyama, and K. Endo, J. Cell. Biochem., 2003, 88, 286. CrossRef
9. T. Matsumoto, T. Miki, H. Hagino, T. Sugimoto, S. Okamoto, T. Hirota, Y. Tanigawara, Y. Hayashi, M. Fukunaga, M. Shiraki, and T. Nakamura, J. Clin. Endocrinol. Metab., 2005, 90, 5031. CrossRef
10. N. Kubodera, J. Syn. Org. Chem, Jpn, 1996, 54, 139.
11. N. Kubodera, J. Syn. Org. Chem. Jpn, 2005, 63, 728.
12. B. M. Trost and J. Dumas, J. Am. Chem. Soc., 1992, 114, 1924. CrossRef
13. B. M. Trost, J. Dumas, and M. Villa, J. Am. Chem. Soc., 1992, 114, 9836. CrossRef
14. K. Miyamoto, N. Kubodera, E. Murayama, K. Ochi, T. Mori, and I. Matsunaga, Synth. Commun., 1986, 16, 513. CrossRef
15. S. Hatakeyama, A. Kawase, Y. Uchiyama, J. Maeyama, Y. Iwabuchi, and N. Kubodera, Steroids, 2001, 66, 267. CrossRef
16. K. C. Nicolau, D. P. Papahatjis, D. A. Claremon, R. L. Magolda, and R. E. Dolle, J. Org. Chem., 1985, 50, 1440. CrossRef
17. O. Mitsunobu, Synthesis, 1981, 1. CrossRef
18. J. Maeyama, H. Hiyamizu, K. Takahashi, J. Ishihara, S. Hatakeyama, and N. Kubodera, Heterocycles, 2006, 70, 295. CrossRef
19. N. Kubodera and S. Hatakeyama, Anticancer Res., in press.
20. R. Eastell and B. L. Riggs, ‘Vitamin D and Osteoporosis’ Vitamin D Second Edition, ed. by D. Feldman, J. W. Pike, and F. H. Glorieux, Elsevier Academic Press, Burlington, 2005, pp. 1101-1120. CrossRef
21. S. E. Papapoulos, ‘Bisphosphonates. Pharmacology and Use in the Treatment of Osteoporosis’ Osteoporosis, ed. by R. Marcus, D. Feldman, and J. Kelsey, Academic Press, San Diego, 1996, pp. 1209-1234.
22. Y. Uchiyama, Y. Higuchi, S. Takeda, T. Masaki, A. Shira-Ishi, K. Sato, N. Kubodera, K. Ikeda, and E. Ogata, Bone, 2002, 30, 582. CrossRef
23. A. W. Norman, R. Bouillon, M. C. Farach-Carson, J. E. Bishop, L.-X. Zhou, I. Nemere, J. Zhao, K. R. Muralidharan, and W. H. Okamura, J. Biol. Chem., 1993, 268, 20022.
24. Y. Ono, H. Watanabe, A. Kawase, N. Kubodera, T. Okano, N. Tsugawa, and T. Kobayashi, Bioorg. Med. Chem. Lett., 1994, 4, 1523. CrossRef
25. K. Eto, A. Fujiyama, M. Kaneko, K. Takahashi, J. Ishihara, S. Hatakeyama, Y. Ono, and N. Kubodera, Heterocycles, 2009, 77, 323. CrossRef
26. A. J. Brown, C. Ritter, E. Slatopolsky, K. R. Muralidharan, W. H. Okamura, and G. S. Reddy, J. Cell. Biochem., 1999, 73, 106. CrossRef
27. A. J. Brown, C. Ritter, A. S. Weiskopf, P. Vouros, G. J. Sasso, M. R. Uskokovic, G. Wang, and G. S. Reddy, J. Cell. Biochem., 2005, 96, 569. CrossRef
28. S. F. Martin and J. A. Dodge, Tetrahedron Lett., 1991, 32, 3017. CrossRef
29. K. Nakamura and Y. Hirose, J. Synth. Org. Chem. Jpn, 1995, 53, 668.
30. M. Yamaguchi and I. Hirao, Tetrahedron Lett., 1983, 24, 391. CrossRef
31. S. Hatakeyama, S. Nagashima, N. Imai, K. Takahashi, J. Ishihara, A. Sugita, T. Nihei, H. Saito, F. Takahashi, and N. Kubodera, Bioorg. Med. Chem., 2006, 14, 8050. CrossRef
32. S. Hatakeyama, S. Nagashima, N. Imai, K. Takahashi, J. Ishihara, A. Sugita, T. Nihei, H. Saito, F. Takahashi, and N. Kubodera, J. Steroid Biochem. Mol. Biol., 2007, 103, 222. CrossRef
33. A. Fujiyama, M. Kaneko, K. Takahashi, J. Ishihara, S. Hatakeyama, and N. Kubodera, Heterocycles, 2007, 71, 2263. CrossRef
34. Y. Takahashi, T. Suda, S. Yamada, H. Takayama, and Y. Nishii, Biochemistry, 1981, 20, 1681. CrossRef
35. S. Yamada, M. Ohmori, H. Takayama, Y. Takasaki, and T. Suda, J. Biol. Chem., 1983, 258, 457.
36. R. Kruse, G. Popjak, J. E. Bishop, and A. W. Norman, Biochemistry, 1983, 22, 1798. CrossRef
37. N. Ikekawa and Y. Fujimoto, J. Synth. Org. Chem. Jpn, 1988, 46, 455.
38. N. Ikekawa, Med. Res. Rev., 1987, 7, 333. CrossRef
39. M. R. Uskokovic, A. W. Norman, P. S. Manchand, G. P. Studzinski, M. J. Cambell, H. P. Koeffler, A. Takeuchi, M.-L. Siu-Caldera, D. S. Rao, and G. S. Reddy, Steroids, 2001, 66, 463. CrossRef
40. H. H. Inhoffen, G. Quinkert, S. Schutz, G. Friedrich, and E. Tober, Chem. Ber., 1958, 91, 781. CrossRef
41. E. Schrotter, B. Schonecker, U. Hauschild, P. Drorscher, and H. Schick, Synthesis, 1990, 193. CrossRef
42. X. Zhao, P. De Clercq, M. Vandewalle, K. Allwaert, H. Van Baelen, and R. Bouillon, Bioorg. Med. Chem. Lett., 1993, 3, 1863. CrossRef
43. S. Ishizuka, K. Bannai, T. Naruchi, and Y. Hashimoto, Steroids, 1981, 37, 33. CrossRef
44. L. K. Revelle, J. M. Londowski, S. B. Kost, R. A. Corradino, and R. Kumar, J. Steroid Biochem., 1985, 22, 469. CrossRef
45. C. H. Heathcock, M. C. Pirrung, J. Lampe, C. T. Buse, and S. D. Young, J. Org. Chem., 1981, 46, 2290. CrossRef
46. A. P. M. Veek and F. H. Putten, Tetrahedron Lett., 1970, 11, 3951. CrossRef
47. S. Hanessian, A. Bargiotti, and M. A. LaRue, Tetrahedron Lett., 1978, 19, 737. CrossRef
48. P. Camps, J. Font, and O. Ponsati, Tetrahedron Lett., 1981, 22, 1471. CrossRef
49. P. Camps, J. Cardellach, J. Font, R. M. Ortuno, and O. Ponsati, Tetrahedron, 1982, 38, 2395. CrossRef
50. Y. Ono, H. Watanabe, I. Taira, K. Takahashi, J. Ishihara, S. Hatakeyama, and N. Kubodera, Steroids, 2006, 71, 529. CrossRef