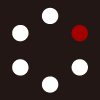
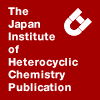
HETEROCYCLES
An International Journal for Reviews and Communications in Heterocyclic ChemistryWeb Edition ISSN: 1881-0942
Published online by The Japan Institute of Heterocyclic Chemistry
e-Journal
Full Text HTML
Received, 2nd October, 2008, Accepted, 19th November, 2008, Published online, 26th November, 2008.
DOI: 10.3987/COM-08-S(D)59
■ N-Glycosylhydroxylamines as Masked Polyhydroxylated Chiral Nitrones in Cycloaddition Reactions: An Access to Pyrrolizidines
Marco Marradi, Massimo Corsi, Stefano Cicchi, Marco Bonanni, Francesca Cardona, and Andrea Goti*
Department of Organic Chemistry “Ugo Schiff”, University of Florence, via della Lastruccia 13, I-50019 Sesto Fiorentino (FI), Italy
Abstract
N-glycosylhydroxylamines undergo 1,3-dipolar cycloadditions via their tautomeric open chain nitrones. Cycloadditions to maleic acid esters occur with high diastereoselectivity and the products can be converted into highly functionalized pyrrolizidines.INTRODUCTION
During the last decades, chiral nitrones have emerged as useful substrates for the enantiospecific synthesis of nitrogen heterocycles through 1,3-dipolar cycloadditions,1 additions of organometallic reagents,2 or SmI2 induced reductive couplings.3 In this context, carbohydrate-derived nitrones were particularly useful for accessing iminosugars and their hydroxylated congeners.4
N-glycosylnitrones 6 are readily available by condensation of the corresponding N-glycosylhydroxylamines 3a with an aldehyde5 or by oxidation of the corresponding N-alkyl-N-glycosylhydroxylamines 3b (Scheme 1).6,7 In turn, hydroxylamines 3 are directly accessible by condensation of carbohydrate derivatives, such as lactol 1, with hydroxylamine hydrochloride to give 3a5 or with an appropriate N-substituted hydroxylamine to afford 3b.6-8 N-glycosylnitrones 6 have been used extensively in organic synthesis in reactions with both dipolarophiles7,9 and nucleophiles10 in order to achieve asymmetric induction. The sugar moiety serves as a chiral auxiliary which can be removed by an acidic treatment at a later stage of the synthetic sequence. However, the same N-alkyl-N-glycosyl hydroxylamines 3b may react as masked nitrones in view of their tautomeric equilibrium with open chain nitrones 5, albeit the equilibrium is completely or largely shifted to cyclic hydroxylamines,7,8 in contrast to the usual behaviour of unsubstituted N-glycosylhydroxylamine, where the equilibrium favours the oximes 4.5,9 In this case, reactions of nitrones 5 afford adducts embodying the carbohydrate chain framework, which can then be used for the construction of the final desired compound. The discovery that N-alkyl-N-glycosylhydroxylamines 3b react via the tautomeric open chain nitrones 5 is due to the pioneering studies of Dondoni and co-workers, who reported the addition of organolithium and organomagnesium reagents to a series of hydroxylamines derived from furanoses en route to iminosugar derivatives.8,11 More recently, we have applied this concept in order to achieve both domino and stepwise double additions of Grignard reagents at C(1) and C(1’) of N-glycosylnitrones 6 and N-alkyl-N-glycosylhydroxylamines 3b.12 We have also disclosed in a preliminary communication the first example of an intermolecular cycloaddition reaction of an N-glycosylhydroxylamine,13 i.e. N-benzyl-N-erythrofuranosyl hydroxylamine 7, with dimethyl maleate, thus demonstrating that also the cycloaddition route was viable for compounds 3b.7 A recent article by Argyropoulos and co-workers detailing the cycloaddition of (ent)-7 to methyl acrylate and the elaboration of the adducts into trihydroxypyrrolizidines14 prompted us to report our own results on this subject. In this paper we describe studies on the cycloaddition reactions of a few N-benzyl-N-glycosylhydroxylamines with dimethyl maleate and the conversion of a cycloadduct into a pyrrolizidine, precursor of natural product analogues which possess glycosidase inhibitory properties.
In principle, an appropriate carbohydrate derivative/lactol, eg 10, may afford the same, or a stereoisomeric, N-bridghehead bicyclic compound either by means of a cyclic nitrone, eg 11, or a N-glycosylhydroxylamine/open chain nitrone, eg 7/8, after cycloaddition and subsequent cyclization (Scheme 2). Since we have recently used the chiral cyclic nitrone 11, readily obtained from erythrose 10, to access polyhydroxypyrrolizidines 14 and 15,15 unnatural analogues of the alkaloids croalbinecine and crotanecine, respectively, we selected the same starting carbohydrate derivative in order to compare the expected product(s) from adduct(s) 9 with pyrrolizidinone 13, intermediate to the target compounds. Indeed, the same compound or one (or more) of its diastereoisomers might be produced, depending on the diastereoselectivity of the cycloaddition step.
RESULTS AND DISCUSSION
The first attempts to react the hydroxylamine 7 in a 1,3-dipolar cycloaddition reaction through its tautomer 816 were carried out with dimethyl maleate by heating at 80 °C in toluene (Scheme 3).
The TLC and 1H NMR spectrum of the crude reaction mixture showed the presence of one major diastereoisomer and several other products. The major product was isolated in 62% yield and it was structurally assigned as the endo-erythro (anti) isomer 16 on the basis of its 1D and 2D NMR spectra, analogy with similar results reported in the literature and mechanistic considerations. Indeed, preferred attack of the dipolaphile to the Si face of nitrone in an endo fashion according to the models proposed by DeShong17 and Thomas18 for related cycloaddition reactions would afford product 16. The preferred N,O-antiperiplanar conformation of 16 is in agreement with the high H(3)-H(4’) coupling constant (10 Hz)14,19 and with observed nOe enhancement (Figure 1). Definite proof of the correctness of the structural assignment was given by subsequent transformation of 16 to a known pyrrolizidinone (see below).
Careful NMR investigation of all the chromatographic fractions revealed the presence of at least four minor products, three of which showed a pattern of signals resembling that of 16, while the last one gave quite a distinct spectrum. All the minor products formed in very low yield (about 20% all together), the most abundant amounting to ca. 8%, and none of them was obtained completely pure from the others. Since four diastereoisomers are theoretically expected from the addition of dimethyl maleate to 7 (two syn and two anti compounds deriving from exo and endo approaches, respectively), the question arose where one (at least) product was coming from. Studies of the outcome of the cycloaddition of 7 and other N-glycosylhydroxylamines (see below) with dimethyl maleate suggested two possible reasons for the superabundance of reaction products. The first accounts for the minor compound displaying the distinct NMR spectrum. Indeed, the lowfield portion of this spectrum resembles that of the starting sugar 10 and N-glycosylhydroxylamine 7, suggesting that the erythrofuranosyl moiety survived albeit the product was clearly an addition product of dimethyl maleate. The spectroscopic and analytic data of this compound were identical with those of an authentic sample of the cycloadduct 18, previously obtained from dimethyl maleate and C-phenyl-N-erythrosylnitrone 17.7 Therefore, the harsh reaction conditions were responsible for a partial oxidation of 7 to the corresponding N-glycosylnitrone 17 which subsequently added dimethyl maleate to give the adduct 18 (Scheme 4). The other minor compounds are most likely stereoisomers 9 (Scheme 4) of the major adduct 16. However, their structure was not unequivocally assigned, since recovery from the reaction mixture (especially from other less reactive N-glycosylhydroxylamines) of a considerable amount of dimethyl fumarate together with unreacted dimethyl maleate indicated that double bond isomerization may occur under the reaction conditions, increasing to eight the number of possible resulting stereoisomers. It was suspected that the hydroxylamine substrate may be responsible for the double bond isomerization through a reversible conjugate addition-elimination to the electron-poor alkene.20 The efficiency of a disubstituted hydroxylamine in inducing such isomerization was proved by heating dimethyl maleate in toluene at 90 °C in the presence of N,N-dibenzylhydroxylamine: after 12 h the 1H NMR spectrum of the solution revealed a ca. 3:1 fumarate/maleate mixture.
Albeit formation of the major cycloadduct was already satisfactory, occurring with a diastereomeric ratio of about 80% and good yield, it was envisaged that lowering the reaction time and/or the reaction temperature would limit both the undesired oxidation to N-glycosylnitrone 17 and isomerization to dimethyl fumarate, which were individuated as responsible for the formation of some of the minor products. All the attempts made at changing the reaction medium to a more polar one (H2O, alcohols, aprotic dipolar, ionic liquids) or by using a Lewis acid, in order to get a 7/8 equilibrium mixture more favorable to the open chain nitrone, failed and only starting materials or the hydrolyzed lactol 10 were recovered. Eventually, performing the reaction under solventless conditions allowed shortening of the reaction time by a factor 12 and only traces of dimethyl fumarate were found among the products. The yield of 16 increased of about 10% and, more significantly, the diastereomeric ratio was higher than 90%, with by-products formation being greatly avoided (Scheme 3). It is worthy to note that cycloaddition of the enantiomeric hydroxylamine (ent)-7 to methyl acrylate gave two adducts with only 2.6:1 diastereoselectivity.14
Having proved the feasibility of N-erythrosylhydroxylamine 7 to afford cycloaddition reactions, a few
other N-glycosylhydroxylamines were tested in order to generalize this methodology. More challenging substrates 19-217 (Scheme 5), whose tautomeric equilibrium lies far to the cyclic hydroxylamine and no open chain nitrone is evidenced in CDCl3 solution by 1H NMR, were then reacted with dimethyl maleate. The ribofuranosyl hydroxylamine 19 and glucopyranosyl hydroxylamine 20 gave only complex reaction mixtures, even under solvent free conditions. Cycloaddition products were only detected in low amounts and extensive isomerization to dimethyl fumarate was observed, especially in case of 20 which required to increase the reaction temperature to 100 °C in order to get a liquid mixture. Conversely, the arabinopyranosyl hydroxylamine 21 gave an excellent result, highlighting the tremendous advantage of using the solvent free conditions (Scheme 5). The reaction in xylene at 100 °C furnished a complex mixture of products. Four diastereomeric cycloadducts, formed with low stereoselectivity, were detected, two of which likely derived from cycloaddition of dimethyl fumarate, which was also detected in the mixture. The major adduct, which was assigned the structure 23 by analogy with the cycloaddition of 7, was isolated with a poor 20% yield. The same adduct could be obtained in a rewarding 82% isolated yield and 88% diastereomeric ratio by running the reaction under solvent free conditions.
Apparently, substrates characterized by a tautomeric equilibrium heavily shifted to the cyclic N-glycosylhydroxylamine form (ie, compounds 19 and 20) require too harsh conditions (high temperature, very long reaction time) for the cycloaddition to occur and other reactions (oxidation, hydrolysis, isomerisation, decomposition) may compete.
As an exemplification of the synthetic utility of these adducts as anticipated in Scheme 2, attempts to convert the adduct from erythrosyl hydroxylamine 7 into the corresponding pyrrolizidinone by N-O bond reduction, debenzylation and double cyclization were carried out. If the previous structural assignment was correct, the adduct 16 should afford the same pyrrolizidinone 13 previously obtained from the cyclic chiral nitrone 11.15
The first route explored was direct mesylation of the adduct 16, with the hope to isolate the cyclized isoxazolidinium salt 25, which on hydrogenation was expected to afford directly the pyrrolizidinone 13 by debenzylation, ring-opening and cyclization (Scheme 6). The primary alcohol 16 turned out to be unusually unreactive under common mesylation conditions (stoichiometric NEt3 or pyridine in CH2Cl2 or THF, either at rt or reflux), which may be reasonably ascribed to a strong intramolecular H bond with the dioxolane ring. Eventually, 16 was forced to react with methanesulfonyl chloride by using pyridine as solvent at 0 °C. However, the spectra of the isolated product did not match the expected data for the salt 25. The 1H NMR spectrum evidenced the lack of two hydrogen atoms and the 13C NMR showed the presence of two quaternary olefinic signals at 139 and 140 ppm and one methyl signal at 39 ppm typical for a mesylate. The pyrrolidine 28, whose formation would occur through the mechanism depicted in Scheme 6, is consistent with the spectroscopic data. Thus, the salt 25 is formed but is unstable under the reaction conditions. The acidic H(2) proton is abstracted by pyridine, giving ring-opening to the neutral pyrrolidine 26 which equilibrates to its tautomer 27.21 This compound may undergo further mesylation to the final product 28. This proposed route involves two equivalents of MsCl with respect to the isoxazolidine 16. Accordingly, the yield of pyrrolidine 28 was improved from 53% to 88% on increasing the amount of MsCl used from 1.3 to 2 equivalents.
Then, it was decided to invert the two cyclization steps, operating the reductive ring-opening/lactamization first (Scheme 7). Hydrogenation of the isoxazolidine 16 gave the expected lactam 29 in good yield through debenzylation, reduction of the labile N-O bond and condensation with elimination of methanol. Since attempted direct cyclization under Mitsunobu conditions failed, the alcohol 29 was converted into the corresponding mesylate 30. Final cyclization to 13, key intermediate for the synthesis of crotanecine and croalbinecine analogues 14 and 15, was achieved in 30% yield (not optimized) by treatment with NaH. The physical and spectroscopic data of pyrrolizidinone 13 were identical with those of the compound previously obtained from the chiral cyclic nitrone 11,15,22 thus confirming the structural assignment to the adduct 16. The process reported here represents therefore a formal synthesis of polyhydroxypyrrolizidines 14 and 15. It is noteworthy that the crotanecine analogue 15 is a powerful inhibitor of α–mannosidases from jack beans and from almonds15 and of drosophila Golgi α–mannosidase II (dGMII).23,24
In conclusion, we have demonstrated that N-benzyl-N-glycosyl hydroxylamines undergo intermolecular cycloaddition reactions through their open chain nitrone tautomers. The cycloadditions are favoured by solvent free conditions and occur with a high degree of stereoselectivity. The adducts are precursors of highly functionalized chiral nitrogen heterocyclic compounds, as proved by a formal synthesis of polyhydroxypyrrolizidines related to the necine bases of pyrrolizidine alkaloids.
EXPERIMENTAL
General Methods: N-Benzyl-N-glycosylhydroxylamines 7 and 19-21 were synthesized according to ref. 7. Commercial reagents were used as received. All reactions were magnetically stirred and monitored by TLC on 0.25 mm silica gel plates (Merck F254) and column chromatography was carried out on Silica Gel 60 (32-63 μm). Yields refer to spectroscopically and analytically pure compounds. 1H and 13C NMR spectra were recorded on Varian Gemini-200 or Varian Mercury-400. Proton and carbon chemical shifts are reported relative to the residue peaks of the solvent CDCl3 (7.26 ppm for 1H and 77.0 ppm for 13C). Infrared spectra were recorded with a Perkin-Elmer Spectrum BX FT-IR System spectrophotometer. Mass spectra were recorded on a QMD 1000 Carlo Erba instrument by direct inlet; relative percentages are shown in brackets. Elemental analyses were performed with a Perkin-Elmer 2400 analyzer. Optical rotation measurements were performed on a JASCO DIP-370 polarimeter.
General procedure for the cycloadditions of N-glycosylhydroxylamines with dimethyl maleate.
In solution. A Sovirel vial is charged with the N-glycosylhydroxylamine 7 or 21 (1.0 mmol) and dry toluene or xylene (2 mL) is added purging with nitrogen for few minutes. Dimethyl maleate (2 equiv) is added and the mixture is heated in an oven at 80 °C for 6 d or at 100 °C for 3 d, respectively. The solvent is then evaporated and the crude mixture is purified by FCC affording adducts 16 and 23.
Neat. The reaction is performed under solvent free conditions charging the vial as above except for the aromatic solvent. The vial is then heated in an oven at 80 °C for 12 h.
Dimethyl (3S,4R,5S)-2-benzyl-3-[(4’S,5’R)-5’-(hydroxymethyl)-2’,2’-dimethyl-1’,3’-dioxolan-4’-yl]- 4,5-isoxazolidinedicarboxylate (16)
White solid, 71% yield (AcOEt:petroleum ether = 1:3, Rf = 0.21); mp 65-66 °C; 1H NMR δ 7.48-7.39 (m, 2H), 7.36-7.21 (m, 3H), 4.88 (d, J = 10.0 Hz, 1H), 4.39 (d, J = 13.0 Hz, 1H), 4.32 (dt, J = 8.0, 5.5 Hz, 1H), 4.21 (dd, J = 10.0, 1.5 Hz, 1H), 4.14 (dd, J = 10.0, 1.5 Hz, 1H), 3.99 (dd, J = 9.5, 5.5 Hz, 1H), 3.98 (d, J = 13.0 Hz, 1H), 3.80 (s, 3H), 3.79 (s, 3H), 3.68 (ddd, J = 13.0, 11.0, 1.5 Hz, 1H), 3.44-3.38 (m, 2H), 1.42 (s, 3H), 1.35 (s, 3H); 13C NMR δ 171.5 (s), 170.5 (s), 136.0 (s), 128.2 (d, 2C), 126.8 (d, 2C), 126.3 (d), 108.9 (s), 79.4 (d), 77.2 (d), 75.7 (d), 67.0 (d), 61.8 (t), 59.2 (t), 53.1 (d), 52.8 (q), 52.5 (q), 27.8 (q), 25.5 (q); IR (CH2Cl2) 3359, 3006, 2956, 2893, 1743, 1435, 1372, 1342, 1238 cm-1; MS m/z (rel. int.) 394 (4%, M+-CH3), 278 (55), 91 (100), 59 (35). Anal. Calcd for C20H27NO8: C, 58.67; H, 6.65; N, 3.42. Found: C, 58.85; H, 6.70; N, 3.44. [α]D22 –36 (c 1.04, CHCl3).
Dimethyl (3S,4R,5S)-2-benzyl-3-{(R)-hydroxy[(4’S,5’R)-5’-(hydroxymethyl)-2’,2’-dimethyl-1’,3’- dioxolan-4’-yl]methyl}-4,5-isoxazolidinedicarboxylate (23)
Colourless oil, 82% yield (AcOEt:petroleum ether = 1:1, Rf = 0.21); 1H NMR δ 7.39-7.21 (m, 5H), 4.77 (d, J = 8.8 Hz, 1H), 4.39 (d, J = 13.2 Hz, 1H), 4.15-4.02 (m, 4H), 3.75-3.62 (m, 3H), 3.49 (bd, J = 6.6 Hz, 1H), 3.72 (s, 3H), 3.69 (s, 3H), 3.20-2.80 (bs, 2H, exchangeable protons), 1.40 (s, 3H), 1.16 (s, 3H); 13C NMR δ 171.2 (s), 170.1 (s), 136.8 (s), 129.4 (d, 2C), 128.4 (d, 2C), 127.5 (d), 108.2 (s), 79.0 (d), 77.0 (d), 76.4 (d), 70.1 (d), 68.1 (d), 62.0 (t), 60.8 (t), 52.7 (d), 52.5 (q), 52.4 (q), 26.6 (q), 24.7 (q); IR (CH2Cl2) 3500-3300 (br), 3068, 3033, 2990, 2954, 2935, 2858, 1740, 1496, 1456, 1438, 1383, 1215, 1037 cm-1; MS m/z (rel. int.) 439 (4%, M+), 278 (31), 90 (100), 59 (73). Anal. Calcd for C21H29NO9: C, 57.39; H, 6.65; N, 3.19. Found: C, 57.19; H, 7.02; N, 3.23. [α]D20 –0.8 (c 0.62, CH2Cl2).
2-[(3a’S,6a’R)5’-Benzyl-2’,2’-dimethyl-tetrahydro-[1’,3’]dioxolo[4’,5’-c]pyrrol-4’-yl]-3- methanesulfonyloxy-but-2-enedioic acid dimethyl ester (28)
Compound 16 ( 164 mg, 0.4 mmol) was dissolved in dry pyridine and the resulting solution was cooled with an ice bath. The stirring solution was slowly (5 min) added with freshly distilled methanesulfonyl chloride (104 μl, 0.8 mmol). The solution was left at 0 °C for 20 min and at rt for 2 h. The final suspension was filtered and the solid washed with dry CH2Cl2. The solution was concentrated and the crude reaction mixture purified by flash column chromatography to afford pure 28 (166 mg, 0.35 mmol).
Colorless viscous oil, 88% yield (AcOEt:petroleum ether = 1:2, Rf = 0.40); 1H NMR δ 7.33-7.19 (m, 5H), 4.99 (dd, J = 6.8, 5.0 Hz, 1H), 4.59 (dt, J = 5.2, 6.4 Hz, 1H), 4.08 (d, J = 13.2 Hz, 1H), 4.03 (d, J = 5.0 Hz, 1H), 3.85 (s, 3H), 3.84 (s, 3H), 3.38 (s, 3H), 3.0 (d, J = 12.8, 1H), 3.20 (dd, J = 10.4, 6.2 Hz, 1H), 2.37 (dd, J = 10.2, 5.0 Hz, 1H), 1.54 (s, 3H), 1.30 (s, 3H); 13C NMR δ 165.5 (s), 161.0 (s), 139.2 (s), 138.2 (s), 138.1 (s), 128.4 (d, 2C), 128.3 (d, 2C), 127.1 (d), 113.7 (s), 83.4 (d), 77.8 (d), 68.5 (d), 58.1 (t), 57.9 (t), 52.3 (q), 52.7 (q), 39.7 (q), 27.4 (q), 25.5 (q); IR (CH2Cl2) 3027 (w), 2956 (m), 1736 (s), 1601 (m), 1437 (m), 1374 (w), 1289 (s), 1149 (m), 1089 (m) cm-1; MS m/z (rel. int.) 391 (4%), 91 (100), 59 (6). Anal. Calcd for C21H27NO9S: C, 53.72; H, 5.80; N, 2.98. Found: C, 53.58; H, 6.13; N, 3.29. [α]D20 +15.7 (c 0.72, CHCl3).
Methyl (2S,3R,4S)-4-hydroxy-2-[(4’S,5’R)-5’-(hydroxymethyl)-2’,2’-dimethyl-1’,3’-dioxolan-4’-yl]- 5-oxo-3-pyrrolidinecarboxylate (29)
Palladium hydroxide, 20% wt. on carbon (1.13 g) is added to a solution of isoxazolidine 16 (1.13 g, 2.75 mmol) in MeOH (26 mL) and the mixture is stirred under hydrogen atmosphere at rt for 15 h. After removal of the solvent the crude is quickly filtered through a short pad of silica gel to get the desired product 29 (644 mg, 2.2 mmol).
Oil, 81% yield (AcOEt:CH2Cl2:MeOH = 10:1:1, Rf = 0.48); 1H NMR δ 4.63 (br s, 1H, exchangeable proton), 4.46 (d, J = 8.8 Hz, 1H), 4.34 (dt, J = 9.6, 4.8 Hz, 1H), 4.11-3.87 (m, 3H), 3.75 (s, 3H), 3.67 (dd, J = 11.0, 2.6 Hz, 1H), 3.05 (dd, J = 8.8, 7.2 Hz, 1H), 1.30 (s, 6H); 13C NMR δ 175.5 (s), 172.8 (s), 108.9 (s), 80.6 (d), 76.2 (d), 73.0 (d), 59.5 (t), 53.4 (d), 52.8 (d), 52.5 (q), 27.1 (q), 25.1 (q); IR (CH2Cl2) 3331, 2995, 2903, 1713, 1437, 1373, 1342, 1256, 1166, 1060 cm-1; MS m/z (rel. int.) 274 (12%, M+-CH3), 159 (80), 131 (100), 59 (75). Anal. Calcd for C12H19NO7: C, 49.82; H, 6.62; N, 4.84. Found: C, 50.30; H, 6.84; N, 4.82; [α]D20 –41.7 (c 0.46, CHCl3).
Methyl (2S,3R,4S)-2-[(4’S,5’R)-2’,2’-dimethyl-5’-{[(methylsulfonyl)oxy]methyl}-1’,3’-dioxolan-4’- yl]-4-hydroxy-5-oxo-3-pyrrolidinecarboxylate (30)
Compound 29 (270 mg, 0.93 mmol) is dissolved in dry pyridine (9.2 mL) and the solution is cooled at -18 °C. A solution of methanesulfonyl chloride (87 μL, 1.1 mmol) in pyridine (3 mL) is then added and the mixture is allowed to go to rt under stirring over 2 h. The solvent is then evaporated and the crude is purified by FCC to obtain pure 30 (219 mg, 0.59 mmol).
Solid, 64% yield (CH2Cl2:MeOH = 20:1, Rf = 0.16); mp 50-51 °C; 1H NMR δ 7.62 (bs, 1H, exchangeable proton), 4.48 (d, J = 8.4 Hz, 1H), 4.46-4.39 (m, 1H), 4.32-4.24 (m, 2H), 4.19-4.11 (m, 1H), 3.99 (dd, J = 8.4, 7.3 Hz, 1H), 3.76 (s, 3H), 3.13 (s, 3H), 3.08 (dd, J = 8.4, 7.3 Hz, 1H), 1.39 (s, 3H), 1.34 (s, 3H); 13C NMR δ 176.2 (s), 172.2 (s), 109.5 (s), 79.2 (d), 74.2 (d), 72.4 (d), 67.0 (t), 52.7 (d), 52.5 (d), 52.2 (q), 37.5 (q), 27.2 (q), 25.1 (q); IR (CH2Cl2) 3556, 3414, 2992, 2963, 2940, 1734, 1439, 1365, 1270, 1179 cm-1; MS m/z (rel. int.) 367 (M+, 1), 324 (15), 240 (26), 213 (25), 136 (39), 108 (28), 96 (44), 79 (67), 70 (100), 60 (84). Anal. Calcd for C13H21NO9S: C, 42.50; H, 5.76; N, 3.81. Found: C, 42.51; H, 5.82; N, 4.20. [α]D23 +9.0 (c 1.27, CH2Cl2).
Methyl (3aR,7S,8R,8aS,8bS)-7-hydroxy-2,2-dimethyl-6-oxohexahydro-4H-[1,3]dioxolo[4,5-a] pyrrolizine-8-carboxylate (13)
Sodium hydride dispersed in mineral oil (60%, 13 mg, 0.32 mmol) is washed with petroleum ether (freshly distilled from CaH2) and then suspended in dry THF (3 mL). A solution of compound 30 in dry THF (3 mL) is added to the cooled (-20 °C) suspension and the mixture is allowed to go to rt over 2 h under stirring. After one hour at rt, the solution is quenched with water (13 mL) at 0 °C and then extracted with CH2Cl2 (3 x 4.5 mL). The organic phase is dried over Na2SO4 and concentrated to afford pyrrolizidinone 13 (13 mg, 0.05 mmol).
White solid, 30% yield; (AcOEt:petroleum ether = 5:1, Rf = 0.20); mp 160-162 °C; [α]D20 –76.0 (c 1.00, CHCl3). The spectroscopic data are in agreement with those reported in the literature.15,22
ACKNOWLEDGEMENTS
The Authors thank MIUR - Italy for financial support and Ente Cassa di Risparmio di Firenze - Italy for granting a 400 MHz NMR spectrometer. Brunella Innocenti and Maurizio Passaponti are acknowledged for technical assistance.
References
1. J. J. Tufariello, ‘1,3-Dipolar Cycloaddition Chemistry’, ed. by A. Padwa, John Wiley & Sons, New York, 1984, ; P. N. Confalone and E. M. Huie, Org. React., 1988, 36, 1; K. B. G. Torssell, ‘Nitrile Oxides, Nitrones, and Nitronates in Organic Synthesis’, ed. by H. Feuer, VCH Publishers, New York, 1988, ; R. C. F. Jones and J. N. Martin, ‘Synthetic Applications of 1,3-Dipolar Cycloaddition Chemistry Toward Heterocycles and Natural Products’, ed. by A. Padwa and W. H. Pearson, John Wiley & Sons, New York, 2002.
2. R. Bloch, Chem. Rev., 1998, 98, 1407; CrossRef D. Enders and U. Reinhold, Tetrahedron: Asymmetry, 1997, 8, 1895; CrossRef M. Lombardo and C. Trombini, Synthesis, 2000, 759; CrossRef P. Merino, In ‘Science of Synthesis’ , ed. by A. Padwa, Thieme, Stuttgart, 2004, Vol. 27.
3. F. Cardona and A. Goti, Angew. Chem. Int. Ed., 2005, 44, 7832; CrossRef G. Masson, S. Py, and Y. Vallée, Angew. Chem. Int. Ed., 2002, 41, 1772; CrossRef G. Masson, P. Cividino, S. Py, and Y. Vallée, Angew. Chem. Int. Ed., 2003, 42, 2265; CrossRef D. Riber and T. Skrydstrup, Org. Lett., 2003, 5, 229; CrossRef O. N. Burchak, C. Philouze, P. Y. Chavant, and S. Py, Org. Lett., 2008, 10, 3021. CrossRef
4. M. Frederickson, Tetrahedron, 1997, 53, 403; CrossRef K. V. Gothelf and K. A. Jørgensen, Chem. Rev., 1998, 98, 863; CrossRef A. E. Koumbis and J. K. Gallos, Curr. Org. Chem., 2003, 7, 585; CrossRef H. M. I. Osborn, N. Gemmell, and L. M. Harwood, J. Chem. Soc., Perkin Trans. 1, 2002, 2419; CrossRef J. Revuelta, S. Cicchi, A. Goti, and A. Brandi, Synthesis, 2007, 485; P. Merino, S. Franco, F. L. Merchan, and T. Tejero, Synlett, 2000, 442; M. Lombardo and C. Trombini, Curr. Org. Chem., 2002, 6, 695; CrossRef M. Kleban, P. Hilgers, J. N. Greul, R. D. Kugler, J. Li, S. Picasso, P. Vogel, and V. Jäger, ChemBioChem, 2001, 2, 365; CrossRef A. M. Palmer and V. Jäger, Eur. J. Org. Chem., 2001, 1293; CrossRef M. Bonanni, M. Marradi, F. Cardona, S. Cicchi, and A. Goti, Beilstein J. Org. Chem., 2007, 3, 44, doi:10.1186/1860-5397-3-44. CrossRef
5. A. Vasella, Helv. Chim. Acta, 1977, 60, 426. CrossRef
6. P. Merino, S. Franco, F. L. Merchan, and T. Tejero, Synth. Commun., 1997, 27, 3529. CrossRef
7. S. Cicchi, M. Corsi, M. Marradi, and A. Goti, Tetrahedron Lett., 2002, 43, 2741; CrossRef S. Cicchi, M. Marradi, M. Corsi, C. Faggi, and A. Goti, Eur. J. Org. Chem., 2003, 4152. CrossRef
8. A. Dondoni and D. Perrone, Tetrahedron Lett., 1999, 40, 9375; CrossRef A. Dondoni, P. P. Giovannini, and D. Perrone, J. Org. Chem., 2002, 67, 7203. CrossRef
9. A. Vasella, Helv. Chim. Acta, 1977, 60, 1273; CrossRef A. Vasella and R. Voeffray, J. Chem. Soc., Chem. Commun., 1981, 97; CrossRef A. Vasella and R. Voeffray, Helv. Chim. Acta, 1982, 65, 1134; CrossRef A. Vasella and R. Voeffray, Helv. Chim. Acta, 1982, 65, 1953; CrossRef A. Vasella, R. Voeffray, J. Pless, and R. Huguenin, Helv. Chim. Acta, 1983, 66, 1241; CrossRef K. Kasahara, H. Iida, and C. Kibayashi, J. Am. Chem. Soc., 1986, 108, 4647; CrossRef S. Mzengeza and R. A. Whitney, J. Org. Chem., 1988, 53, 4074; CrossRef K. Kasahara, H. Iida, and C. Kibayashi, J. Org. Chem., 1989, 54, 2225; CrossRef F. Machetti, F. M. Cordero, F. De Sarlo, A. Guarna, and A. Brandi, Tetrahedron Lett., 1996, 37, 4205; CrossRef O. Tamura, N. Mita, N. Kusaka, H. Suzuki, and M. Sakamoto, Tetrahedron Lett., 1997, 38, 429; CrossRef U. Chiacchio, A. Corsaro, G. Gumina, A. Rescifina, D. Iannazzo, A. Piperno, G. Romeo, and R. Romeo, J. Org. Chem., 1999, 64, 9321; CrossRef U. Chiacchio, A. Rescifina, A. Corsaro, V. Pistarà, G. Romeo, and R. Romeo, Tetrahedron: Asymmetry, 2000, 11, 2045; CrossRef O. Tamura, A. Kanoh, M. Yamashita, and H. Ishibashi, Tetrahedron, 2004, 60, 9997; CrossRef O. Tamura, N. Iyama, and H. Ishibashi, J. Org. Chem., 2004, 69, 1475. CrossRef
10. R. Huber, A. Knierzinger, J.-P. Obrecht, and A. Vasella, Helv. Chim. Acta, 1985, 68, 1730; CrossRef B. Bernet, E. Krawczyk, and A. Vasella, Helv. Chim. Acta, 1985, 68, 2299; CrossRef R. Huber and A. Vasella, Helv. Chim. Acta, 1987, 70, 1461; CrossRef R. Huber and A. Vasella, Tetrahedron, 1990, 46, 33; CrossRef I. Lantos, J. Flisak, L. Liu, R. Matsuoka, W. Mendelson, D. Stevenson, K. Tubman, L. Tucker, W.-Y. Zhang, J. Adams, M. Sorenson, R. Garigipati, K. Erhardt, and S. Ross, J. Org. Chem., 1997, 62, 5385; CrossRef N. Mita, O. Tamura, H. Ishibashi, and M. Sakamoto, Org. Lett., 2002, 4, 1111; CrossRef F. Mancini, M. G. Piazza, and C. Trombini, J. Org. Chem., 1991, 56, 4246; CrossRef J. C. Rohloff, T. V. Alfredson, and M. A. Schwartz, Tetrahedron Lett., 1994, 35, 1011; CrossRef A. Basha, R. Henry, M. A. McLaughlin, J. D. Ratajczyk, and S. J. Wittenberger, J. Org. Chem., 1994, 59, 6103; CrossRef R. Fässler, D. E. Frantz, J. Oetiker, and E. M. Carreira, Angew. Chem. Int. Ed., 2002, 41, 3054; CrossRef S. K. Patel, S. Py, S. U. Pandya, P. Y. Chavant, and Y. Vallée, Tetrahedron: Asymmetry, 2003, 14, 525. CrossRef
11. A. Dondoni and D. Perrone, Tetrahedron, 2003, 59, 4261; CrossRef A. Dondoni and A. Nuzzi, J. Org. Chem., 2006, 71, 7574. CrossRef
12. M. Bonanni, M. Marradi, S. Cicchi, C. Faggi, and A. Goti, Org. Lett., 2005, 7, 319; CrossRef M. Bonanni, M. Marradi, S. Cicchi, and A. Goti, Synlett, 2008, 197.
13. For examples of intramolecular cycloadditions of such compounds, see: S. M. Jachak, N. P. Karche, and D. D. Dhavale, Tetrahedron Lett., 2001, 42, 4925; CrossRef R. J. Ferrier and P. Prasit, J. Chem. Soc., Chem. Commun., 1981, 983. CrossRef
14. N. G. Argyropoulos, P. Gkizis, and E. Coutouli-Argyropoulou, Tetrahedron, 2008, 64, 8752. CrossRef
15. S. Cicchi, M. Marradi, P. Vogel, and A. Goti, J. Org. Chem., 2006, 71, 1614. CrossRef
16. The 1H NMR in CDCl3 at rt showed a 94:6 7/8 equilibrium mixture (see ref. 7). .
17. P. DeShong, C. M. Dicken, J. M. Leginus, and R. R. Whittle, J. Am. Chem. Soc., 1984, 106, 5598. CrossRef
18. M. J. Fray, R. H. Jones, and E. J. Thomas, J. Chem. Soc., Perkin Trans. 1, 1985, 2753. CrossRef
19. Similar values have been reported for related cycloadducts (see ref. 17).
20. An analogous isomerization induced by a monosubstituted hydroxylamine has been observed: H. G. Aurich, H. Franzen, and H. G. Rohr, Tetrahedron, 1994, 50, 7417. CrossRef
21. For similar oxidative ring-opening processes of isoxazolidines to aminoketones induced by strong bases, see: F. M. Cordero, F. Machetti, F. De Sarlo, and A. Brandi, Gazz. Chim. Ital., 1997, 127, 25; S.-I. Murahashi, Y. Kodera, and T. Hosomi, Tetrahedron Lett., 1988, 29, 5949; CrossRef C. Hootelé, W. Ibebeke-Bomangwa, F. Driessens, and S. Sabil, Bull. Soc. Chim. Belg., 1987, 96, 57; J. J. Tufariello and Sk. Asrof Ali, J. Am. Chem. Soc., 1979, 101, 7114. CrossRef
22. A. Goti, S. Cicchi, M. Cacciarini, F. Cardona, V. Fedi, and A. Brandi, Eur. J. Org. Chem., 2000, 3633. CrossRef
23. W. Zhong, D. A. Kuntz, B. Ember, H. Singh, K. W. Moremen, D. R. Rose, and J.-G. Boons, J. Am. Chem. Soc., 2008, 130, 8975; CrossRef N. Shah, D. A. Kuntz, and D. R. Rose, Proc. Natl. Acad. Sci. U.S.A., 2008, 105, 9570. CrossRef
24. The structure of a dGMII.15 complex has been resolved (D. A. Kuntz and D. R. Rose, personal communication). Full details will be given in a forthcoming full paper.