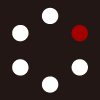
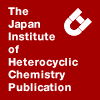
HETEROCYCLES
An International Journal for Reviews and Communications in Heterocyclic ChemistryWeb Edition ISSN: 1881-0942
Published online by The Japan Institute of Heterocyclic Chemistry
e-Journal
Full Text HTML
Received, 2nd October, 2008, Accepted, 17th November, 2008, Published online, 19th November, 2008.
DOI: 10.3987/COM-08-11571
■ On the Photoisomerization of Thiophene and Thiazole Derivatives
Maurizio D'Auria* and Rocco Racioppi
Department of Chemistry, University of Basilicata, Via N. Sauro 85, 85100 Potenza, Italy
Abstract
The photochemical isomerization of thienyl and thiazolyl derivatives can be explained by assuming the formation of the Dewar isomer. The isomerization of the Dewar isomer towards the most stable one can account for the observed reaction. No biradical intermediate was found. In the case of thiophene an isomerization of the Dewar thiophene allows the formation of a tricyclic intermediate; a retroelectrocyclic reaction can afford the reaction product. This tricyclic intermediate cannot be formed in the photoisomerization of thiazole derivative.INTRODUCTION
The isomerization of pentaatomic heterocyclic compounds is one of the most important photochemical reaction of this type of compounds.1-8 Five mechanisms can be invoked in order to justify the photochemical isomerization of pentaatomic aromatic heterocycles: 1. the ring contraction – ring expansion route (RCRF) (Scheme 1, A); 2. the internal cyclization – isomerization route (ICI) (Scheme 1, B); 3. the van Tamelen – Whitesides general mechanism (VTW) (Scheme 1, C); 4. the zwitterion – tricycle route (ZT) (Scheme 1, D); 5. the fragmentation – readdition route (FR) (Scheme 1, E). Recently, we reported that the photochemical isomerization of pentaatomic aromatic heterocycles can be described using a unifying hypothesis.1-8
If the first excited singlet state of a molecule is populated, the molecule can convert into the corresponding triplet state or into the corresponding Dewar isomer. The efficiency of these processes will depend on energetic factors. If the Dewar isomer is formed, the isomeric product is obtained. If the triplet state is formed, cleavage of X-C<συβ>α</συβ> bond can occur to give ring opening products, decomposition products or ring contraction products.
Here we want to report our results on the photochemical isomerization of thiophene and thiazole derivatives. All the reactions can be explained supposing the formation of the Dewar isomer as a first intermediate. Biradical intermediates were not found in our calculations. The possibility of other type of intermediates has be studied.
RESULTS AND DISCUSSION
The irradiation of thiophene in liquid phase gave the Dewar thiophene.9 The Dewar thiophene and cyclopropene-3-thiocarbaldehyde can be obtained by irradiation in argon matrices at 10 K.10 Wynberg discovered the most interesting reaction in the photochemical reactivity of thienyl derivatives. The irradiation of 2-substituted thiophenes gave the corresponding 3-substituted derivatives (Scheme 1). Several studies have been accomplished on the mechanism of this photoisomerization showing that the reaction takes place from the singlet excited state of the molecule.11,12 Four mechanisms have been proposed (Scheme 1, A-D).13,14 Several studies showed that mechanism B is the most probable.15,16 Alkylthiophenes reacted to give the corresponding transposition products but they showed low reactivity.17 Better results were obtained using perfluoroalkyl derivatives.18-20 Arylthiophenes were used as substrates in the photoisomerization described in the Scheme 1.21-26 Carbonyl and olefinic substituents inhibit the rearrangement.
We performed some calculations by using DFT/B3LYP/6-31G+(d,p) method on Gaussian03.27 Excited states were determined by using TD/DFT/B3LYP/6-31G+(d,p) method. We studied the photoisomerization of 2-phenylthiophene, as depicted in Scheme 2.
The results of our calculations are shown in Figure 1 and Table 1. The first excited π,π* singlet state was an effect of a transition at 286 nm. The absorption is due to a HOMO-LUMO transition and the involved orbitals are depicted in the Figure 2. The electronic transitions allowed populating an orbital where both C2-C3 and C4-C5 bonds are broken allowing the formation of the Dewar isomer.
On the basis of our results we can note that: 1. we did not observe the formation of biradical species as showed in SINDO1 semiempirical calculations performed on 2-cyanopyrrole;28 2. we did not observe the formation of the biradical species 1 (Figure 3), found in a semiempirical study on thiophene isomerization;29 3. the transposed Dewar thiophene is less stable than the first one for 0.1 kcal mol-1.
However, a second way to obtain a photoisomerization of 2-phenylthiophene can be described. If 1 cannot be found as a reaction intermediate, the ring closure product 2 (Figure 3) has been identified as a possible intermediate.23 This intermediate can be converted into 3-phenylthiophene through a retroelectrocyclic reaction.
Furthermore, Wynberg reported 5-deutero and 5-methyl-2-phenylthiophene gave a photoisomerization product that cannot be obtained on the basis of first way (blue line in Figure1) described above (Scheme 3).12,24,25 However, the second reaction path (red line in Figure 1) could explain the formation of the observed products.
The results of our calculations in this case are reported in Figure 4. The formation of the tricyclic intermediate can explain the observed behavior.
More recently, we reported that the irradiation of the iodothiazole derivative 3 in the presence of aromatic and heteroaromatic compounds gave the arylation products where an isothiazole ring is present (Scheme 4).30
The irradiation of thiazole did not give any interesting product.31 However, 2-, 4-, and 5-methyl thiazole gave the corresponding isothiazoles in low yields when irradiated in trifluoroacetic acid.32
Many data have been reported on the reactivity of 2-phenylthiazole. In a pioneering work, the irradiation of this compound led to 4-phenylthiazole and 3-phenylisothiazole.33 Subsequently, the same authors reported that the irradiation of a benzene solution of 2-phenylthiazole (obtained through irradiation of 2-iodothiazole in benzene) in the presence of iodine leads to 3-phenylisothiazole, the major product, in addition to small amounts of 4-phenylthiazole. When the reaction was carried out without iodine, the main product was 4-phenylthiazole, while 3-phenylisothiazole was present in a trace amount.34-36 By contrast, 4-phenylthiazole practically did not react, and 5-phenylthiazole gave 4-phenylisothiazole as the main product in very low yields.36,37
2-Phenyl-5-methylthiazole furnishes mainly 3-phenyl-4-methylisothiazole; 2-methyl-5-phenylthiazole gives 3-methyl-5-phenylisothiazole; 4-methyl-5-phenylthiazole leads to the formation of 3-methyl-4-phenylisothiazole; 2-methyl-4-phenylthiazole gives 3-methyl-5-methylisothiazole; and, finally, 4-phenyl-5-methylthiazole is converted into 3-phenyl-4-methylisothiazole.38 2,5-Diphenylthiazole, irradiated in benzene at 80 °C, gave 3,4-diphenylisothiazole as the major product, together with minor amounts of 4,5-diphenylthiazole and its cyclized derivative. 2,4-Diphenylthiazole gave only a very low yield of 3,5-diphenylisothiazole, while 4,5-diphenylthiazole was converted into the corresponding cyclized product and 3,4-diphenylisothiazole.39,40
The irradiation of a 1:1 Cu-peplomycin complex, an antibiotic of the bleomycin family, leads to a product of an isomerization of a thiazole ring.41 This behavior has been verified with bithiazole and trithiazole derivatives.41,42
The authors generally considered the above results consistent with an ICI mechanism. In this contest, iodine favors the intersystem crossing or the opening of the Dewar intermediate, as reported in the Scheme 5.35
We studied the photoisomerization of methyl 2-phenylthiazole-5-carboxylate (4) into methyl 3-phenylisothiazole-4-carboxylate (5) (Scheme 6).
The results of our calculations are depicted in Figure 5 and Table 2. The first excited π,π∗ singlet state was populated through a HOMO-LUMO transition at 320 nm (Figure 6).
The orbital involved are very similar to those observed in the photoisomerization of 2-phenylthiophene. Also in this case the excitation populates an orbital where C2-C3 and C4-C5 bonds are broken.
Singlet excited 4 can be converted into the Dewar isomer 6 (Figures 5 and 7). This intermediate, in contrast with the hypothesis showed in the Scheme 7, isomerizes to the Dewar isomer 7 (Figures 5 and 7) without any biradical intermediate in an exothermic process (6.8 kcal mol-1). The reaction has a biradical as the transition state of the reaction. The Dewar isomer 6 could give the thiazole derivative 7 (Figure 7), but we did not observe the formation of this isomer. Furthermore, the conversion of the Dewar isomer 6 into the isomer 8 is an exothermic process (0.22 kcal mol-1) (Figures 5 and 7). Also in this case the biradical is a transition state, found at an energy higher that the starting material for 16.68 kcal mol-1).
As shown in Figure 7 the Dewar thiazole derivative 6 can be converted in 9 in a single step, with a transition state (Figure 8) with an energy 12.57 kcal mol-1 higher than the starting material. Then, this seems to be the most probable way to obtain the product.
Finally, we tested the possibility to obtain a tricyclic intermediate similar to that showed above for the photochemical isomerization of the thiophene derivatives. The results are shown in Figure 7. The tricyclic intermediate could be formed but it shows a very high energy. Starting from the Dewar isomer 6, the tricyclic intermediate could be obtained in an endothermic process, where the last shows a higher energy for almost 38 kcal mol-1 than that of 6. On this basis, we can exclude its formation, and for this reason, we did not calculate the energy of the transition states involved in the process.
In conclusion, the photochemical isomerization of thienyl and thiazolyl derivatives can be explained assuming the formation of the Dewar isomer. The isomerization of the Dewar isomer towards the most stable one can account for the observed reaction. No biradical intermediate was found. In the case of thiophene an isomerization of the Dewar thiophene allows the formation of a tricyclic intermediate; a retroelectrocyclic reaction can afford the reaction product. This tricyclic intermediate cannot be formed in the photoisomerization of thiazole derivative.
EXPERIMENTAL
Gaussian03 have been used for the discussions about the computed geometries. All the computations were based on the Density Functional Theory (DFT) 43 and Time-Dependent DFT (TD-DFT)44 by using the B3LYP hybrid functional.45 Geometry optimizations and TD-DFT results from the Gaussian03 program have been obtained at the B3LYP/6-31G(d,p) level of approximation. Geometry optimizations were performed with default settings on geometry convergence (gradients and displacements), integration grid and electronic density (SCF) convergence. Redundant coordinates were used for the geometry optimization as produced by the Gaussian03 program. Analytical evaluation of the energy second derivative matrix w.r.t. Cartesian coordinates (Hessian matrix) at the B3LYP/6-31G(d,p) level of approximation confirmed the nature of minima of transition structures of the energy surface points associated to the optimized structures.
References
1. M. D’Auria, ‘Targets in Heterocyclic Systems, Chemistry and Properties: unified approach to the photochemical isomerization of five-membered heteroaromatic compounds’, Vol. 2, ed. by O. A. Attanasi and D. Spinelli, Italian Society of Chemistry, Rome, 1999, pp. 233-279.
2. M. D’Auria, Heterocyles, 1999, 50, 1115. CrossRef
3. M. D’Auria, J. Org. Chem., 2000, 65, 2494. CrossRef
4. M. D’Auria, Adv. Heterocyclic Chem., 2001, 79, 41. CrossRef
5. M. D’Auria, J. Photochem. Photobiol., 2002, 149, 31. CrossRef
6. M. D’Auria, Tetrahedron, 2002, 58, 8037. CrossRef
7. M. D’Auria and R. Racioppi, Lett. Org. Chem., 2004, 1, 12. CrossRef
8. S. Buscemi, M. D’Auria, A. Pace, I. Pibiri, and N. Vivona, Tetrahedron, 2004, 60, 3243. CrossRef
9. W. A. Rendall, M. Torres, and O. P. Strausz, J. Am. Chem. Soc., 1985, 107, 723. CrossRef
10. W. A. Rendall, A. Clement, M. Torres, and O. P. Strausz, J. Am. Chem. Soc., 1986, 108, 1691. CrossRef
11. R. M. Kellogg and H. Wynberg, Tetrahedron Lett., 1968, 5895. CrossRef
12. H. Wynberg, R. M. Kellogg, H. van Driel, and G. E. Beekhuis, J. Am. Chem. Soc., 1967, 89, 3501. CrossRef
13. H. Wynberg, Acc. Chem. Res., 1971, 4, 65. CrossRef
14. R. M. Kellogg, Tetrahedron Lett., 1972, 1429. CrossRef
15. T. Matsushita, H. Tanaka, K. Nishimoto, and Y. Osamura, Theor. Chim. Acta, 1983, 63, 55. CrossRef
16. K. Jug and H.-P. Schluff, J. Org. Chem., 1991, 56, 129. CrossRef
17. R. M. Kellogg, J. K. Dik, H. van Driel, and H. Wynberg, J. Org. Chem., 1970, 35, 2737. CrossRef
18. A. Greenberg, J. F. Liebman, and D. van Vechten, Tetrahedron, 1980, 36, 1161. CrossRef
19. Y. Kobayashi, K. Kawada, A. Ando, and I. Kumadaki, Heterocycles, 1983, 20, 174. CrossRef
20. Y. Kobayashi, K. Kawada, A. Ando, and I. Kumadaki, Tetrahedron Lett., 1984, 25, 1917. CrossRef
21. H. Wynberg and H. van Driel, J. Am Chem. Soc., 1965, 87, 3998. CrossRef
22. H. Wynberg, R. M. Kellogg, H. van Driel, and G. E. Beekhuis, J. Am. Chem. Soc., 1966, 88, 5047. CrossRef
23. H. Wynberg and H. van Driel, J. Chem. Soc., Chem. Commun., 1966, 203. CrossRef
24. H. Wynberg, H. van Driel, R. M. Kellogg, and J. Buter, J. Am. Chem. Soc., 1967, 89, 3487. CrossRef
25. R. M. Kellogg and H. Wynberg, J. Am. Chem. Soc., 1967, 89, 3495. CrossRef
26. H. Wynberg, G. E. Beekhuis, H. van Driel, and R. M. Kellogg, J. Am. Chem. Soc., 1967, 89, 3498. CrossRef
27. M. J. Frisch, G. W. Trucks, H. B. Schlegel, G. E. Scuserie, M. A. Robb, J. R. Cheeseman, J. A. Jr Montgomery, T. Vreven, K. N. Kudin, J. C. Burant, J. M. Millam, S. S. Iyengar, J. Tomasi, V. Barone, B. Mennucci, M. Cossi, G. Scalmani, N. Rega, G. A. Petersson, H. Nakatsuji, M. Hada, M.Ehara, K. Toyota, R. Fukuda, J. Hasegawa, M. Ishida, T. Nakajima, Y. Honda, O. Kitao, H. Nakai, M. Klene, X. Li, J. E. Knox, H. P. Hratchian, J. B. Cross, C. Adamo, J. Jaramillo, R. Gomperts, R. E. Stratmann, O. Yazyev, A. J. Austin, R. Cammi, C. Pomelli, J. W. Ochterski, P. Y. Ayala, K. Morokuma, G. A. Voth, P. Salvador, J. J. Dannenberg, V. G. Zakrzewski, S. Dapprich, A. D. Daniels, M. C. Strain, O. Farkas, D. K. Malik, A. D. Rabuck, K. Raghavachari, J. B. Foresman, J. V. Ortiz, Q. Cui, A. G. Baboul, S. Clifford, J. Cioslowski, B. B. Stefanov, G. Liu, A. Liashenko, P. Piskorz, I. Komaromi, R. L. Martin, D. J. Fox, T. Keith, A. Al-Laham, C. Y. Peng, A. Nanayakkara, M. Challacombe, P. M. W. Gill, B. Johnson, W. Chen, M. W. Wong, C. Gonzales, and J. A. Pople, Gaussian 03, Revison A1, Gaussian, Inc., Pittsburgh PA, 2003.
28. S. Behrens and K. Jug, J. Org. Chem., 1990, 55, 2288. CrossRef
29. K. Jug and H.-P. Schluff, J. Org. Chem., 1991, 56, 129. CrossRef
30. M. Amati, S. Belviso, M. D’Auria, F. Lelj, R. Racioppi, and L. Viggiani, Eur. J. Org. Chem., submitted.
31. J. P. Catteau, A. Lablache-Combier, and A. Pollet, J. Chem. Soc., Chem. Commun., 1969, 1018. CrossRef
32. J. W. Pavlik, C. R. Pandit, C. J. Samuel, and A. C. Day, J. Org. Chem., 1993, 58, 3407. CrossRef
33. G. Vernin, J.-C. Poite, J. Metzger, J.-P. Aune, and H. J. M. Dou, Bull. Soc. Chim. Fr., 1971, 1103.
34. G. Vernin, R. Jauffred, C. Ricard, H. J. M. Dou, and J. Metzger, J. Chem. Soc., Perkin Trans. 2, 1972, 1145. CrossRef
35. C. Riou, G. Vernin, H. J. M. Dou, and J. Metzger, Bull. Soc. Chim. Fr., 1972, 2673.
36. J. W. Pavlik, P. Tongcharoensirikul, N. P. Bird, A. C. Day, and J. A. Barltrop, J. Am. Chem. Soc., 1994, 116, 2292. CrossRef
37. C. Vernin, C. Riou, H. J. M. Dou, L. Bouscasse, J. Metzger, and G. Loridan, Bull. Soc. Chim. Fr., 1973, 1743.
38. C. Riou, J. C. Poite, G. Vernin, and J. Metzger, Tetrahedron, 1974, 30, 879. CrossRef
39. M. Kojima and M. Maeda, J. Chem. Soc., Chem. Commun., 1970, 386.
40. M. Maeda and M. Kojima, J. Chem. Soc., Perkin Trans. 1, 1978, 685. CrossRef
41. I. Saito, T. Morii, Y. Okamura, S. Mori, K. Yamaguchi, and T. Matsuura, Tetrahedron Lett., 1986, 27, 6385. CrossRef
42. I. Saito, T. Morii, S. Mori, K. Yamaguchi, and T. Matsuura, Tetrahedron Lett., 1988, 29, 3963. CrossRef
43. R. G. Parr and W. Yang, Density Functional Theory of Atoms and Molecules, Oxford University Press, New York, 1989, .
44. M. E. Casida, In “Recent Advances in Density Functional Methods”, Vol. 1, ed. by D. P. Chong, World Scientific, Singapore, 1995, pp. 155-192; M. E. Casida, C. Jamorski, K. C. Casida, and D. R. Salahub, J. Chem. Phys., 1998, 108, 4439. CrossRef
45. A. D. Becke, J. Chem. Phys., 1993, 98, 5648. CrossRef