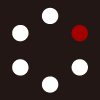
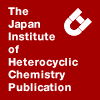
HETEROCYCLES
An International Journal for Reviews and Communications in Heterocyclic ChemistryWeb Edition ISSN: 1881-0942
Published online by The Japan Institute of Heterocyclic Chemistry
e-Journal
Full Text HTML
Received, 4th October, 2008, Accepted, 25th November, 2008, Published online, 3rd December, 2008.
DOI: 10.3987/REV-08-646
■ Synthesis of Five- and Six-Membered Heterocyclic Compounds by the Application of the Metathesis Reactions
Krishna C. Majumdar,* Sanjukta Muhuri, Rafique Ul Islam, and Buddhadeb Chattopadhyay
Department of Chemistry, University of Kalyani, Kalyani 741 235, West Bengal, India
Abstract
This review describes the formation of five- and six-membered heterocyclic rings in various compounds by ring closing diene and enyne metathesis.Contents
1. Introduction
2. General aspects of metathesis reaction
2.1 Catalysts used for the metathesis and their recent modifications
3. Synthesis of oxygen heterocycles
3.1 Synthesis of five-membered cyclic ethers
3.2 Synthesis of six-membered cyclic ethers
3.3 Synthesis of five- and six-membered lactones
4. Synthesis of nitrogen heterocycles
4.1 Synthesis of five-membered nitrogen heterocycles
4.2 Synthesis of six-membered nitrogen heterocycles
4.3 Synthesis of five- and six-membered lactams
5. Synthesis of nitrogen and oxygen heterocycles by domino process via RCM
5.1 Synthesis of heterocycles by using ROM / RCM strategy
5.2 Synthesis of heterocycles by using tandem RCM
6. Miscellaneous
7. Conclusions
8. Acknowledgements
9. Abbreviations
10. References
1. INTRODUCTION
Since the 1960s the famous olefin metathesis has been known, but it was not the early 1990s that this conversion became an important tool in synthetic organic chemistry. Grubbs and Fu published two significant papers1 indicating the application of ring-closing metathesis (RCM) for the synthesis of simple five- six- and seven-membered monocyclic systems bearing oxygen and nitrogen atoms by utilizing a molybdenum catalyst that had been first prepared by Schrock. Metathesis is the exchange of parts of two substances. In the generic reaction,
AB + CD ---> AC + BD

B has changed position with C. The name “olefin metathesis” was first given by Calderon.2 Olefin metathesis is truly an amazing reaction in which the strongest bond in an alkene, the C=C double bond, is broken and remade. In 1971, Chauvin proposed3 a mechanism for the olefin metathesis that is beautiful in its simplicity: a metal carbene comes together with an alkene to reversibly form a four-membered ring metallocycle. This metallocyclobutane ring can open in a different way to generate a new carbene complex and a new alkene (Figure 1).
Other supports for Chauvin’s metallocyclobutane mechanism came from the research group of Robert H. Grubbs.4 With understanding of the basic mechanism of olefin metathesis the new challenge was to construct efficient catalyst that would tolerate the various functional groups in the typical organic molecules. Though olefin metathesis has been known since 1960’s, but this area of research became familiar to us after the discovery of the air stable Grubbs’ catalysts (1992 and 1995). The number of applications of this reaction has dramatically increased in the past few years due to tremendous improvements in the functional group compatibility and reactivity of the catalysts.
2. GENERAL ASPECTS OF METATHESIS REACTION
Olefin metathesis is a unique carbon skeleton redistribution in which unsaturated carbon- carbon bonds are rearranged in the presence of metal carbene complexes. With the advent of efficient catalysts, this reaction has emerged as a powerful tool for the formation of C-C bonds in synthetic organic chemistry. Although alkene metathesis constitutes, for example, the most widely utilized type of metathesis reaction, recent years have witnessed the discovery and development of a number of related processes employing a broader range of substrates. Prominent amongst these are the enyne metathesis, which involves the union of an alkyne with an alkene to form 1,3-diene system. Enyne metatheses are wholly atom economical and are, therefore, driven by enthalpic rather than entropic factors. On the basis of mechanistic studies on enyne metathesis, a Chauvin-type3 mechanism involving an ‘yne-then-ene’ (Figure 2) sequence was proposed. Very recently Lloyd-Jones5 reported an alternative ‘ene-then-yne’ mechanism for Ru-catalyzed enyne ring closing metathesis reaction for which ethylene can catalyze the reaction.
Ring closing metathesis has been developed into one of the most powerful and reliable method for ring formation. A variety of ring systems,6-8 such as regular, medium and large carbo- or heterocyclic, can be constructed by the application of ring-closing metathesis reaction. Selectivity issues can arise in the metathesis chemistry when more than one ring is formed during a ring closing process. Recently, relay RCM has been developed as a method to overcome both the reactivity and selectivity issues in the metathesis chemistry.9 The presence of heteroatom in the substrate leads to a variety of results.10-19 Nitrogen is generally not tolerated unless non basic functional groups such as tosylamides are used. Certain metathesis reactions of amine-containing compounds were carried out with one equivalent of p-toluenesulfonic acid to prevent coordination of the electron pair on the nitrogen atom to the catalysts.14 Oxygen appears to be beneficial for the metathesis reactions.15 Surprisingly, however, very limited examples19 of substrates containing sulfur atom have so far been reported. The limited number of examples of metathesis with sulfur containing substrates can be partly explained by the fact that middle to late transition metals used as catalyst may interact favorably with the soft sulfur atom.
A number of reviews20-23 on RCM have been published to date, but none of these focuses solely on the formation of five- and six-membered heterocyclic compounds.24,25 In this review, important recent advances related to the formation of regular rings via RCM is discussed based on the reports published in the literature from 2004 to 2006. An attempt has been made to summarize the reaction conditions, such as choice of catalyst, solvent, temperature, concentration and reaction time, which are crucial for ring closing metathesis reactions. In general this review is organized into oxygen and nitrogen categories and further divided according to the each functional group subclass. It is designed to illustrate typical examples and situations where olefin metathesis was, and can be, used to construct various five- and six-membered heterocyclic compounds.
2.1 CATALYSTS USED FOR THE METATHESIS AND THEIR RECENT MODIFICATIONS
The success of metathesis reaction is largely due to the advent of today’s readily available catalyst systems that display high activity and excellent functional group tolerance. The three catalysts most routinely used by organic chemists (all of which are commercially available) are shown in Figure 3.
One of the most important catalyst system developed by Schrock and co-workers is the alkoxyimidomolybdenum complex represented by A26 in Figure 3. Major advances of this system are its high reactivity towards a broad range of substrates with many steric and electronic variations. The alkoxides in the [Mo] system can readily be altered to adjust their activities. Critical drawbacks of this Mo-based carbene complexes are however its moderate to poor functional group tolerance, high sensitivity to air, moisture or even to trace of impurities present in solvents, thermal instability on storage and expenses. Grubbs and coworkers subsequently introduced ruthenium-based carbene complexes, initially optimized to Grubbs’ catalyst I, as general and practical catalysts for metathesis. Although less reactive than the Schrock Mo-based system A, the first generation Grubbs’ catalyst exhibits much greater functional group tolerance and expanded the scope of olefin metathesis. In particular, the replacement of one of the phosphine ligand in Grubbs’ catalyst I with N-heterocyclic carbene ligands,27 increases the catalytic activity, thermal stability and functional group tolerance of the complex. The second generation Grubbs’ catalyst engenders metathesis reaction with particularly high levels of activity, in certain cases approaching that the Schrock system A, and with a unique reactivity profile that nicely complements both earlier Grubbs’ catalysts I and II. Grubbs’ detail mechanistic studies provided evidence that phosphine dissociation to give a reactive 14-electron ruthenium intermediate was required for reaction with alkene. Highly active NHC catalysts were synthesized by the groups of Herrmann,28 Nolan,29 and Fürstner.30 Along this line Hoveyda,31 Hoffmann,32 and Blechert33,34 reported other related, very active, stable and functional group tolerant ruthenium metathesis catalysts. The first Hoveyda metathesis catalyst is derived from Grubbs’ 1st generation catalyst. It bears only one phosphine and a chelating carbene ligand. The second one bears in addition, an N-heterocyclic carbene ligand instead of the phosphine (Figure 4).
The family of Hoveyda catalysts whose activity compares with that of the 2nd generation Grubbs’ catalyst are especially useful for difficult cases of metathesis of polysubstituted olefins and selective cross metathesis in which homo coupling needs to be avoided.35,36 Grela et al. reported37 variations of the Hoveyda catalyst with increased efficiency (active even at 0oC) when aryl group of the benzylidene ligand bears a nitro group in the meta or para positions or two methoxy substituents. Grela’s group has recently introduced38 a new air stable ruthenium olefin metathesis catalyst derived from inexpensive bisphenol-S with Hoveyda-Grubbs’ catalyst. Grela’s successful idea was based on the destabilization of the Ru-O(ether) chelation that generates the catalytically active 14-electron species. They have also developed39 a novel pyridinium-tagged ruthenium complex to perform metathesis reaction in aqueous as well as ionic medium. Following the discovery of the original Grubbs’ catalyst, much of research40,41 in this area has focused on increasing the stability, selectivity and particularly the activity of ruthenium olefin metathesis catalysts. Grubbs’ recently developed42 a ruthenium based asymmetric olefin metathesis catalyst, containing chiral, and monodentate N-heterocyclic carbene ligands. These are shown to be promising due to their high activity, low catalyst loading, ease of handling and different functional group tolerance relative to that of the molybdenum catalysts. Grubbs’s success story has encouraged the search for ruthenium metathesis catalyst and other ruthenium structures and variations have been published,43-45 including water-soluble ones,46 reaction carried out in ionic liquids47,48 and on solid supports.49-52 Another successful variation of the Ru-benzylidene catalysts was reported by Blechert53 whose strategy to sterically destabilize the Ru-O (ether) bond was to introduce an aryl (phenyl or naphthyl) substituent on the benzylidene aryl in the ortho-position relative to the O-(ether). Very recently Buchmeiser et al.54 also reported a derivative of Schrock catalyst containing fluorinated carboxylates which displays moderate metathesis activity in ROMP, cyclopolymerization and RCM upon addition of quinuclidine as a base.
Though various ruthenium catalysts have been widely used for ring closing olefin metathesis (RCM), ring opening metathesis polymerization (ROMP) and acyclic cross metathesis, one major concern is the removal of colored, toxic ruthenium metal byproducts from the desired products even after purification on silica gel column chromatography several times. Kim et al.55 introduced an efficient method for the removal of dark brown ruthenium byproducts from the reaction mixture. Grela et al. recently reported their new metathesis catalyst56 which exhibited high affinity for silica gel compared to the parent Hoveyda-Grubbs’ catalyst when CH2Cl2 is used as eluant. Utilizing this advantage, they have designed57 an efficient new strategy for homogenous Ru-catalyst phase-separation and recovery, which provided products of excellent purity. The ruthenium complex induces both the metathesis and non-metathesis processes,58 being active in non-metathesis as a result of a simple conversion of Ru-alkylidene into Ru-hydride species. Grubbs’ and co-workers have recently observed59 that decomposition of the catalyst to a hydride dinuclear carbene complex (HRuCRu) could be responsible for the undesirable isomerization during difficult olefin metathesis. When a substrate cannot give a product by metathesis, non-metathetic reactions proceed efficiently or complete recovery of the starting materials was observed. These discoveries actually expanded the synthetic utility of these catalysts beyond olefin metathesis.60,61 Presence of NHC (N-heterocyclic carbene ligand) in the ruthenium carbene complex could also be the key component for cycloisomerization.61
3. SYNTHESIS OF OXYGEN HETEROCYCLES
In recent years significant activities have been observed in the synthesis of oxygen heterocycles. The versatility of the molybdenum and ruthenium catalysts in cyclization of different ring sizes was amply demonstrated even in the presence of diverse functionalities. Substrates containing oxygen are found to be beneficial for the metathesis in some studies,15,16 while in other cases no influence is observed.
3.1 SYNTHESIS OF FIVE-MEMBERED CYCLIC ETHERS
Synthesis of substituted furans by ring closing metathesis was described by Donohoe et al.62 Precursors 2 were cyclized by Grubbs’ catalyst II (10 mol%) and these heterocycles were finally aromatized to 3 simply by adding trifluoriacetic acid (Scheme 1). To avoid the purification problem due to the non-polar phosphane residue from the catalyst and non-polar aromatic products, the RCM reaction was quenched and more polar dihydrofuran product was first purified by chromatography and finally aromatized by the reaction with TFA (Scheme 1).
Extension of this strategy towards the synthesis of some linked biaryl system was attempted and successfully synthesized pyrrole-furan biaryls 5. Further, a fully aromatized bis-furan system 7 has been synthesized by extending the same protocol (Scheme 2).
Benzofuran skeleton is a well-known structural unit in naturally occurring compounds possessing medicinal values. van Otterlo group63 reported that benzofurans 10 could also be accessible from the corresponding O- and C-allyl phenols 8 using the isomerization technique prior to RCM as reported previously for the synthesis of 2H-chromenes in moderate to excellent yields.64 They also reported the synthesis of substituted benzofurans from the corresponding O,C-diallyl benzenes 8 using 5 mol% ruthenium catalyst-mediated isomerization followed by ring closing metathesis with Grubbs’ catalyst II (Scheme 3). Using the same isomerization-RCM strategy the synthesis of benzodioxene64 starting from catechol was also reported.
Recently, Pujol et al. reported65 the synthesis of spiro-piperidines 12 as potentially valuable functionalized 1,3-dienes starting from N-benzyl piperidone. The dihydrofuran ring of 12 could be constructed at the last stage of the synthesis by intramolecular RCEM. Incidently, this is the first report of the synthesis of spiro furo piperidines using metathesis reaction (Scheme 4).
The synthesis of Solamin (13) has attracted great attention to the synthetic chemists due to its marked antitumor, antimalarial, pesticidal and immunosuppressive properties. The synthesis of central tetrahydro- furan core was achieved66 by means of a ring closing metathesis using Grubbs’ catalyst I or II (Figure 5).
Another structurally related natural product squamostolide was also recently synthesized67 via highly selective tandem ring-closing/cross metathesis step in which lactone formation and fragment coupling were accomplished.
Evans et al.68 reported the stereospecific rhodium catalyzed allylic etherification with secondary alkenyl alcohols along with ring closing metathesis to provide a stereospecific route to the synthesis of cis- and trans-2,5-disubstituted tetrahydrofuran 15. This study also demonstrated that a dramatic enhancement of the stereospecificity is possible by the modification of the Cu(I) alkoxide with trimethylphosphite. The potential of this protocol has been demonstrated by a seven step total synthesis of gaur acid 16 of which RCM is the key step (Scheme 5).
The synthesis of structurally diverse uronic acid side chain attached to the bicycle core 20 was reported by Holmes et al.69 by the application of sequential Claisen rearrangements and ring closing metathesis strategy (Scheme 6). The epoxide protected RCM precursor 18 on treatment with Grubbs’ catalyst I gave the desired ring-closing product 19 which after two steps gave the targeted bicyclic core 20 in moderate yield.
Miharamycins are the nucleoside antibiotics, which show good activity against the rice blast and other fungal diseases. Etheve-Quelquejeu et al.70 reported the synthesis of the bicyclic core of the molecule by utilizing ring closing metathesis from 2-O-allyl-3-allyl-C-ene-ethylene sugar 22. Replacement of the 4,6-O-benzylidene group by protecting acetyl group of the substrates followed by ring closing metathesis with Grubbs’ catalyst II afforded the compound 23 which after a sequence of reaction steps gave the bicyclic moiety of the miharamycin 24 in good yield. Interestingly, 4,6-O-benzylidene protected sugar 21 on ring closing metathesis with Grubbs’ catalyst I / II gave only the homodimer as a mixture of cis- and trans-isomer which may be due to intramolecular constrain during the RCM process (Scheme 7).
3.2 SYNTHESIS OF SIX-MEMBERED CYCLIC ETHERS
Benzo-fused oxa-bicyclic compounds are ubiquitous in nature and exhibit interesting biological properties. Hence they are interesting scaffolds for drug design. Chromene is one of the subclass of benzo-fused bicyclic compounds, which have potent biological activity and spread over natural products. Grubbs reported the synthesis of 2H-chromenes from 6-allyloxystyrene ring closing metathesis using Grubbs’ catalyst I.71
Recently, van Otterlo72-74 reported the synthesis of 2H-chromenes 28 involving an allyl aryl isomerization 27 as one of the key step followed by usual RCM reaction. Modification of the 2H-chromenes skeleton could be readily attainable due to the presence of versatile aldehyde substituents and NO2 group which can be reduced to amine and makes this procedure a valuable one (Scheme 8).
A new synthetic protocol described for the synthesis of naturally occurring antibiotics pentalogin 32 using ring closing metathesis was reported by de Kimpe et al.75 This was achieved from the precursor 30 by ring-closing metathesis with Grubbs’ catalyst I to afford compound 31 and further oxidation of 31 afforded the target molecule 32 (Scheme 9). The protocol opens a new way for the synthesis of other pyranonaphthoquinone antibiotics.
Highly enantioenriched cyclic β-hydroxyallylsilanes have been prepared by Ghosez et al.76 via enantioselective allylation of unsaturated aldehydes using a chiral allyltitanium reagent followed by ring closing metathesis. This strategy has been successfully demonstrated through enantioselective synthesis of dihydropyrans. The RCM precursor 34 was synthesized starting from (+/-) solketal 33. A huge consummation of Grubbs’ catalyst II (25 mol %) afforded the cyclic vinyl ether 35 (Scheme 10). These dihydropyrans could be useful intermediate for the synthesis of glycomimetics.
4H-chromenes are found as structural units in many natural products. Synthesis of 4H-chromenes 38 by RCM using Grubbs’ catalyst from substrates having electron rich vinylic olefins is known to be problematic.77 van Otterlo reported78 the synthesis of this class of compounds by metathesis reaction with phenyl vinyl ethers 37 (Scheme 11).
Recently, enantioselective synthesis of cyclic enol ether and all carbon quaternary stereogenic centres through catalytic asymmetric ring closing metathesis was reported by Hoveyda et al.79 Various chiral Mo- and Ru-based catalysts to identify the most effective one was examined. It was found that the Mo-catalyst 41 (15 mol% in benzene) effectively promoted the enantioselective transformation to access various five- and six- membered cyclic enol ethers in up to 94% ee from readily available achiral starting materials. Representative examples of the formation of the cyclic enol ethers through catalytic asymmetric ring-closing metathesis are shown in Scheme 12. Interestingly, these are the first examples of catalytic asymmetric RCM. This protocol was also utilized for the formation of all carbon quaternary stereogenic centers.
Recently, an interesting example of the formation of small size ring by diene-ene ring closing metathesis of differently substituted pentadienyl ethers and their competition to give either a medium- or small-sized cyclic ethers has been reported by Waldmann et al (Scheme 13).80 The preference for the formation of smaller ring indicates that the reaction is initiated via ruthenium carbene complex formation at the site of the isolated double bond. Further experiments were carried out and observed that small-sized cyclic ethers 43 are thermodynamically controlled and the free hydroxyl group of the diene-ene system 42 does not affect the RCM process. Only six-membered product was obtained. No eight-membered product was isolated.
A ketal-tethered ring closing metathesis approach to the construction of spiroketals have been reported by Hsung81,82 in the synthesis of C11-C23 fragment of Spirastrellolide A, a novel macrolide from the marine sponge spirastrellolide coccinea. In addition to its ability to cause untimely mitotic arrest in cells, spirastrellolide A was shown to exhibit potent inhibitory activity against protein phosphatase 2A. The ring closing metathesis of the precursor 47 was performed by Grubbs’ catalyst I (10 mol%) to give the spiroketal 48 (Scheme 14).
The synthesis of C-glycosides has received considerable attention from both the synthetic and biological point of view. Ring closing metathesis approach is flexible enough to deliver a wide variety of C-glycoside type structure.83
Postema et al. reported84 a metathesis based approach to synthesize β-C-trisaccharides 53 which involved a highly efficient enol-ether-olefin ring closing metathesis cyclization. Precursor 51 on a double ring closing metathesis gave the C-glycal 52. Functionalization of the bis-glycal double bond afforded the β-C-trisaccharides 53. Using this reaction sequence, eight different β-C-trisaccharides were prepared by the same group. β-C-glycosides, which are also convertible to β-C-glycoglycerolipids with potent anti-tumor activity were also synthesized (Scheme 15).
Various synthetic approaches have been reported in recent years to generate nucleoside analogues particularly in search of effective and non-toxic anti-viral and anti-tumor agents. A new family of spiro-annulated carbocyclic nucleosides and conformationally locked bicyclic nucleoside analogues from sugar based substrates has recently been reported by Mandal et al.85 by ring closing metathesis and intramolecular nitrone cycloaddition reaction as the key steps. Diallylated compound 54, generated from D-glucose-derived substrate through O-allylation, underwent ring closing metathesis reaction with Grubbs’ catalyst I to afford the 6/5 spirocycle 55 (Scheme 16). The spiroheterocycle 55 was further elaborated through a sequence of reactions, notably, a nonisolable nitrone intermediate at C1 to afford isooxazolidinospiroheterocycle.
Owing to the potent anticancer activity of bryostatin 70 (Scheme 17), a considerable activity towards the total synthesis of this class of compounds and analogues was displayed.86 An efficient and general synthetic approach towards the synthesis of C1-C16 part of bryostatin 70 was reported by Burke et al.87 utilizing desymmetrization by ketalization and a ring closing metathesis step.
In another approach of enantioselective total synthesis of (-)-mucocin 82, the first member of the acetogenin family bearing a tetrahydropyran (THP) ring along with a tetrahydrofuran (THF) ring was reported recently by Crimmins et al.88 This was achieved by ring closing metathesis as one of the key steps with Grubbs’ catalyst II (Scheme 18).
2,5-Disubstituted tetrahydropyran is an important structural motif in a number of natural product and possesses various biological activities. Schmidt and Nave recently89 reported the regio- and stereoselective synthesis of this class of compounds based on the combination of Grubbs’ catalyst mediated olefin metathesis with either a Claisen rearrangement or Pd-catalyzed allylic substitution as one of the key steps. In continuation of their work on ring closing metathesis, they also reported90 a novel ruthenium catalyzed tandem ring closing metathesis and double bond isomerization reaction for the synthesis of cyclic enol ether in which the metathesis active catalyst ruthenium carbene complex was converted in situ to a isomerization active ruthenium hydride species. Based on this idea, they have synthesized various cyclic enol ethers in moderate-to-good yields.
Large fused polycyclic ether natural products of marine origin are some of the most complex and formidable synthetic targets found in nature and they continue to fascinate and inspire those who are involved in the target oriented synthesis and developments of new synthetic methods.91 Gambierol is a representative marine ladder toxin family with a relatively complex structure. Recently, Rainier et al. reported92,93 the total synthesis of Gambierol 87 in 44 steps in which few key features is enol ether olefin metathesis chemistry, Claisen rearrangement, generation of glycosyl anhydride and C-ketoxide. The D-ring was constructed using Grubbs’ catalyst II (20 mol%) (Scheme 19).
A chiral methylene bis-pyran fragment (C2 – C16 fragment of Phorboxazole A 95) was successfully synthesized by Yadav and co-workers.94 The synthetic route involved the use of an oxy-anion intramolecular Michael addition, Brown’s asymmetric allylation and Grubbs’ ring closing metathesis reaction in a stereoselective manner (Scheme 20).
3.3 SYNTHESIS OF FIVE-AND SIX-MEMBERED LACTONES
Despite the electronic deficiency of the conjugated double bond of the gem-disubstituted allylic alkene unit in the starting acrylates 98, Grubbs’ catalyst I proved to be an effective promoter of the RCM affording the α,β-unsaturated γ-lactones.95 Variation of concentrations of the substrates and /or catalyst showed a positive effect on the yields of the RCM product. The optimized experimental condition was applied on the diolefin 98 with Grubbs’ catalyst I to afford differently alkyl substituted butenolides 99 and the positive effect of dilution on reactivity was attributed to the fact that low substrate concentration can indeed minimize the formation of undesired cross metathesis product, and also imply a longer lifetime of the catalyst (Scheme 21).
Fujii and co-workers reported96 a two-steps synthesis of highly optically active γ-alkyl-γ-butenolides from commercially available recemic hept-1-en-3-ol by the combination of lipase catalyzed transesterification and ring closing metathesis reaction. Grubbs’ catalyst II was found to be an effective one for the asymmetric synthesis of γ-substituted-γ-butenolides in high yields.
The disadvantages (harsh reaction conditions, use of toxic reagents, low yields) of the synthesis of coumarins by classical methods have been overcome by the use of ring closing metathesis.97 Kimpe et al. reported98 a novel synthesis of substituted coumarins 101 from the compounds 100 by ring-closing metathesis with Grubbs’ catalyst II (Scheme 22).
Synthesis of coumarins having substitution both at the aromatic ring and the α,β-unsaturated lactone ring was also developed99 by Lay et al. which can be used as molecular scaffolds suitable for further diversification through a combinatorial approach. Similarly, 3-fluorocoumarin100 has also been prepared by ring closing metathesis with Grubbs’ catalyst II starting from (2-vinylphenyl)-α-fluoroacrylate.
RCM reaction of an exo-methylene unit of a cyclic compound with double bond of suitably tethered allyl ether leading to the formation of furo-[3,4-c]pyran skeleton has been reported.101 The substrates 102, 104 and 105 on treatment with Grubbs’ catalyst II (5 mol %) in CH2Cl2 afforded the furopyrans 103, 106 and 107 in 48-92% yields respectively (Scheme 23).
Substitution either in the allylic part or in the tetrahydrofuran ring of the substrates led to the self-metathesis product rather than the cyclized product. Propargyl ether of the substrate 102 with Grubbs’ catalyst, once again demonstrated the deprotection methodology affording the corresponding alcohol.102
A new strategy for the total synthesis of phomopsolide C by ring-size selective ring closing metathesis and chemoselective cross metathesis was reported103 by Blechert and Michaelis. Cross metathesis of the compounds 108 and 109 with Grubbs-Hoveyda catalyst E (5 mol%) gave the desired regioisomer 110 exclusively in 73% yield which on further acroylation with acrylic acid and deprotection of the trityl group afforded the precursors 75 for the ring closing metathesis. Possibility of migration of the acyl group during the deprotection process was suppressed with HCl solution in CHCl3 by controlling either too acidic or basic conditions. The substrates 111 with 5 mol% Hoveyda-Grubbs’ catalyst E in dichloroethane at 800C for 12 h gave the substituted 5,6-dihydro-5-hydroxypyran-2-ones 112 in 68% yield. Further esterification and subsequent deprotection yielded the desired phomopsolide C 113 (Scheme 24).
In the ring closing metathesis step exclusive formation of the six-membered rather than five-membered lactone was achieved by the installation of more electron withdrawing enone moiety at the position-8, directing the metathesis process to the sterically less hindered more electron rich double bond of the allylic alcohol.
Substituted lactols are useful intermediates in organic synthesis as these synthetic equivalents of ω-hydroxyaldehydes. Using a three-step one-pot reaction, five-membered and six-membered ring lactones 118 and lactols 119 were devised104 by Cossy et al. The procedure consisted of a one-pot tandem cross metathesis / hydrogenation / cyclization at room temperature under 1 atm of hydrogen in the presence of Grubbs’ catalyst II and PtO2. The first step was accomplished by Grubbs’ catalyst II and the second step involved hydrogenation, which was achieved in the presence of PtO2 to give the ω-hydroxyacid 116 or ω-hydroxyaldehydes 117 which immediately cyclized to the corresponding lactones 118 and lactols 119. The catalyst II was found to be tolerant to all the aforesaid reactions and effective for the first step to afford a number of lactols and lactones in 45-70% yields (Scheme 25).
Peloruside A is a 16-membered macrolide with potent taxol-like cytotoxic properties acting on the cell mitotic process at the G2 stage through a mechanism blocking microtubule depolymerization.105 A short and efficient synthesis of the C12-C19 fragment of the cytotoxic macrolide peloruside has been achieved by Ermolenko et al.106 One of the key step of this synthesis is the formation of the six-membered lactone 121 (Scheme 26) and that was accomplished by the RCM reaction with the diene 120 and Grubbs’ catalyst II (10 mol%) for an extended reaction time. The unprecedented diastereomer-discriminating ring closing metathesis reaction leading to the lactone 121 is the vital step for the synthesis of polyketide natural products.
Sharma et al. described107 the stereoselective synthesis of C1-C5 and C4-C5 linked deoxy disaccaharides using RCM strategy. The RCM is the key step, which is depicted in Scheme 27.
The synthesis of forstriecin starting from D-glucose, involves chelation-controlled addition, Wittig rearrangement, ring closing metathesis and iodomethylation. The key intermediate 126 was accessed108 by ring closing metathesis as one of the key steps. Ester alcohol 125 was subjected to ring closing metathesis with Grubbs’ catalyst I (0.1 equiv) in the presence of Ti(O-iPr)4 (0.3 equiv) in refluxing CH2Cl2 for 12 h to afford the lactone 126 from which fostriecin was synthesized in several steps (Scheme 28).
Lactone rings constitute structural features of many natural products. A good deal of naturally occurring lactones, most particularly those being α,β-unsaturated lactones display wide range of pharmacological properties. Total synthesis of various natural product having lactone moiety i.e. (-)-malyngolide (127), (5S,7S,9S,11S)-tetrahydroxyhexacos-2-enoic acid (128), (5R,7S,9S,11S)-tetrahydroxyhexacos-2-enoic acid (129), (+)-boronolide (130), (+)-triacetoxygoniotriol (131), spicigerolide (132), (6S)-5,6-dihydro-6-[(2R)-2-hydroxy-6-phenylhexyl]-2H-pyran-2-one (133), cryptocaryadiacetate (134), (R)-(+)-gonithalamin (135) and (R)-(+)-gonithalamin oxide (136) have been synthesized by various groups109-116 using ring closing metathesis as one of the key steps (Figure 6).
4. Synthesis of nitrogen heterocycles
A number of research groups in recent years have demonstrated the usefulness of RCM for constructing N-heterocycles. Due to the importance of nitrogen-containing compounds, for instance in the synthesis of alkaloids and other biologically active substances, the RCM protocol is an enrichment of synthetic chemistry.
4.1 SYNTHESIS OF FIVE-MEMBERED NITROGEN HETEROCYCLES
A novel sequential palladium/ruthenium-catalyzed four-component process for the synthesis of C-allyl-N-heterocycles was developed by Grigg and coworkers,117 in good yield which in turn can be used as an active dipolarophiles in 1,3-dipolar cycloaddition. 1,6-Dienes 140 underwent ruthenium-catalyzed RCM to give Δ3-pyrrolines 141 (Scheme 29).
The use of RCM to form heteroaromatic compounds has only been recently reported.118 Donohoe et al. reported119 a novel and versatile approach to the synthesis of pyrroles 143 and 145 that uses ring-closing metathesis as the key C-C bond-forming reaction (Scheme 30).
The basicity and nucleophilicity of the N-atom played a crucial role in the RCM reaction of diallylamines. The coordination between the nitrogen atom and the ruthenium species may turn down the expected RCM reaction. The metathesis of diallylamine possessing basic and nucleophilic nitrogen atoms has not been successfully carried out to afford pyrrolodines, even under harsh reaction conditions.120
Xiao and Yu successfully reported121 the straightforward method for the synthesis of enantiopure pyrrolidines by Lewis acid-assisted RCM of chiral diallylamine substrates 146. The RCM reaction of a series of diallylamines 146 were performed using 5-8 mol% Grubbs’ catalyst II and 20 mol% Ti(OiPr)4 in CH2Cl2 (10 ml per 1 mmol of diallylamine) at 400C for 2-13 h to furnish the pyrrolidine derivatives 147 (Scheme 31).
This new procedure demonstrated that basic and nucleophilic diallylamines could participate in RCM reactions with the use of Grubbs’ catalyst II in the presence of Ti(OiPr)4 to prevent the coordination of the N-atom to the ruthenium carbene intermediate. Recently, Stevens et al. reported122 the synthesis of N-benzyl-2-phosphono-3-pyrrolines via RCM starting from functionalized α-aminoalkenyl phosphonates
followed by in situ conversion to the corresponding 2-phosphonopyrroles by tetrachloroquinone (TCQ) (Scheme 32).
Pyne et al. described123 a new strategy for the synthesis of polyfunctionalized pyrrolidines 153 via the ring closing metathesis reaction of substituted 3-allyl-4-vinyl-2-oxazolidones 151 and subsequent diastereoselective cis-hydroxylation of the resulting pyrrolo[1,2-c]oxazol-3-ones 152. These reactions, however required longer reaction times and higher catalyst loading compared to the RCM reaction of their corresponding acyclic diene analogues due to the formation of relatively strained 5,5 hetero-bicyclic system, containing three sp2 hybridized centers (Scheme 33).
The increased thermal stability of Grubbs’ catalyst II, improved the usefulness of the RCM and allowed efficient performance of this reaction at elevated temperature. A rapid method for the preparation of functionalized 2,5-dihydropyrroles containing electron-withdrawing substituents, using ruthenium-catalyzed ring-closing metathesis under microwave irradiation was reported.124 The N-alkylated aza-Baylis-Hillman adducts 154 were converted to the desired ring closing products 2,5-dihydropyrroles 155 within 1 min. reaction time with one exception of the sterically more congested tert-butyl ester which required an additional 60 sec for full conversion (Scheme 34).
Piva et al. developed125 a one-pot procedure, a tandem RCM/ isomerization followed by a sequential radical cyclization, to prepare polycyclic lactams and sultams 158a-c from amides 156a and sulfonamides 156b. This process was also successfully conducted on bisallylsulfonamide to give the corresponding sultams (Scheme 35).
Chang et al. recently reported126 a formal synthesis of pyrrolizidine alkaloid 166, pseudoheliotridane in one- pot by efficient intramolecular ring-closing metathesis of 163 to give 164 using Grubbs’ catalyst II as one of the key steps. Pseudoheliotridane was obtained from the intermediate 164 in several steps (Scheme 36).
Kim et al. achieved127 a new enantioselective synthetic route to (-)-antofine 171 and a representative phenanthroquinolizidine alkaloid (-)-cryptopleurine. The ring-closing metathesis of the bisallylamine 169 was successfully performed with 5 mol% Grubbs’ catalyst II in CH2Cl2 at room temperature for the construction of the pyrrolidine ring 170 from which (-)-antofine 171 was synthesized in two steps (Scheme 37).
Another route for the synthesis of pentahydroxy indolizidine derivatives starting from a carbohydrate intermediate having nitrone functionality has been reported.128 Ring closing metathesis of the dienes 172a and 172b provided sugar substituted dihydropyrrolidine ring systems 173a and 173b respectively that could be elaborated to provide different pentahydroxylated indolizidine alkaloids (Scheme 38).
(+)-Anthramycin, possessing a pyrrolobenzodiazepine skeleton and a dienamide group conjugated with nitrogen in a pyrrolidine ring, was synthesized by Mori et al.129 using ring-closing enyne metathesis and cross metathesis followed by isomerization of the resulting double bond. Pyrrolobenzodiazepine skeleton was constructed by reductive cyclization of a nitro group and an ester group of pyrrolidine derivative 177, which in turn was obtained by enyne metathesis (Scheme 39).
Murakami et al. recently reported130 the first ring-closing metathesis reaction of allenynes 178 occurred at room temperature in the presence of molybdenum complex to give ring-closed vinyl allenes 179. Allenynes 178 were treated with Schrock catalyst (15 mol%) in toluene at room temperature for 3 h. Metathesis between the alkyne and the proximal carbon-carbon double bond of the allene moiety took place to afford five-membered ring product with an allene side chain in good yield (Scheme 40).
4.2 SYNTHESIS OF SIX-MEMBERED NITROGEN HETEROCYCLES
Quinoline is an important heterocyclic moiety present in many alkaloids and played an important role in the field of natural products and medicinal chemistry. A novel synthesis of substituted quinolines using ring closing metathesis and its application to the synthesis of key intermediates for antimalarial agents has recently been reported.131 Various α,ω-dienes 180 were prepared from anthranilic acid derivatives and subjected to ring-closing metathesis using 5 mol% Grubbs’ catalyst I at reflux condition to afford substituted quinolone derivatives 181 in good-to-excellent yields regardless of the substituent pattern on the aromatic ring (Scheme 41).
Effect of the protecting groups on nitrogen was also thoroughly examined. With catalyst I, N-benzyl derivative gave the desired product in excellent yield, N-acetyl did not give the desired product due to formation of some chelated intermediate 182 (Figure 7) and N-tert-butoxycarbonyl derivative gave the product in modest yield. On the other hand with catalyst II, yield of the N-acetyl and N-tert-butoxycarbonyl derivatives were dramatically increased to give the desired product in almost quantitative yield.
It was shown132 that a pure ruthenium hydride complex with N-heterocyclic carbene ligand was effectively generated from the reaction of Grubbs’ catalyst II with vinyloxy trimethyl silane. This ruthenium hydride complex displayed high catalytic activity in the selective isomerization of the terminal olefin and the cycloisomerization of the diene. Substituted 1,2-dihydroquinoline, indole and 3-methylene 2,3-dihydroindole were prepared selectively from the common starting material N-allyl-O-vinylaniline using Grubbs’ catalyst II by slight modification of the reaction conditions.
Diethyl acetoamidomalonate (DEAM) has been used to prepare unusual amino acids, which are known to induce unique conformational restrictions when incorporated into biologically active peptides. Kotha133 developed a simple method for the synthesis of nitrogen containing heterocycles incorporating aminomalonic acid derivatives via RCM reaction. Dialkylated aminomalonic acid dertivative 184 was subjected to ruthenium catalyzed RCM reaction to obtain the corresponding cyclic amino acid derivatives 185 using Grubbs’ catalyst II in dry CH2Cl2 at room temperature under high dilution (Scheme 42).
A new six-membered ring-fused azopino[5,6-b]indole derivative 190 via ring-closing metathesis was reported by Mérour et al.134 The RCM of 189 using Grubbs’ catalyst I in CH2Cl2 at room temperature afforded the tetracyclic compound 190 in 84% yield. The same reaction conditions were also applied to get higher ring sized derivatives in excellent yields (Scheme 43).
Cuadro and Vaquero recently reported135 the first example of an olefin ring-closing metathesis reaction on cationic heteroaromatic systems. The appropriate N-(3-butenyl)-2-vinyl-pyridinium substrates 191 was reacted selectively under RCM condition with Grubbs’ catalyst I (10 mol%) to afford the desired dihydroquinolizinium cations. The quinolizinium system could also be obtained via oxidation of the dihydro compound in good yield. Attempts to achieve RCM on substrate (n = 1) using Grubbs’ catalyst I, II and even with Hoveyda-Grubbs’ catalyst in CH2Cl2 at room temperature was proved to be unsuccessful. Neither the salt nor the most stable neutral compound indolizine could be isolated even with changed reaction conditions (Scheme 44).
Another route to the 1-azabicyclic alkaloids using ring-closing metathesis reaction was reported by Barluenga et al.136 Synthetic route involved the construction of the diene 4-piperidone systems huperzine B (195) by an imino-Diels-Alder reaction between the aminotrienes and N-ω-vinylimines, in the presence of Yb(OTf)3, followed by ring closing metathesis reaction of these cyclic dienes under the influence of Grubbs’ catalyst I. Lee recently reported137 the synthesis of huperzine B via ring-closing metathesis of N-alkenylamine 193 in the presence of Grubbs’ catalyst II followed by selective hydrogenation of the double bond and deprotection with TMSI (Scheme 45).
The tetrahydropyridine moiety138 has been identified as the pharmacophore for GABA (γ-amino butyric acid), one of the major mammalian inhibitory neurotransmitters. But a systematic study of such compounds has been limited due to cumbersome routes for their preparation.139 Ramachandran et al. recently developed140 a conventional preparation of functionalized chiral tetrahydropyridine-3-carboxylate 202 from 201 by ring closing metathesis (Scheme 46).
The RCM reaction using Grubbs’ catalyst II on the aminodiene containing free secondary amine failed to provide any cyclized product. The deficiency of RCM was circumvented by conversion of the amine to the ammonium salt, conversion of secondary amine to the tertiary one or by the protection of the amine. This methodology can also be applied to the synthesis of a chiral C6-substituted tetrahydropyridines with known GABA-inhibitory properties at low concentrations.
Glycosidases are implicated in various diseases such as diabetes, metastatic cancer, malaria and viral infection making glycosidases good target for drug development. Polyhydroxylated piperidines have the ability to inhibit glycosidases and glycosyl transferases, the carbohydrate processing enzymes, which are responsible for the cleavage of glycosidic bonds. Tetrahydropyridines are useful intermediates for the synthesis of iminosugars and various glycosidase inhibitors. Although different unsaturated six-membered nitrogen heterocycles have been synthesized in the last few years using RCM, syntheses of new enantiopure branched tetrahydropyridines as precursors of the potent glycosidase inhibitors are of great interest in recent years. Isofagomine 205, a mimic of glucose and its “gulo” isomer, was prepared141 by Riva et al. through 8-step sequence in 62% overall yield from chiral THYM* [tris(hydroxymethyl) methane] precursor 203 using ring-closing metathesis for the cyclization step (Scheme 47).
Riva et al. also described142 a novel synthetic application of asymmetrized tris(hydroxymethyl)methane (THYM), obtained in both enantiomeric forms in high ee via a chemoenzymetic procedure. Starting from a monoacetate which is a synthetic equivalent of asymmetrized THYM*, six-membered N-heterocycles 207 were prepared exploiting ring-closing metathesis as one of the key steps (Scheme 48).
The O-protected carbamates 206 were subjected to RCM in the presence of Grubbs’ catalyst I to give the corresponding tetrahydropyridines 207. However, different behaviors were observed with Cbz-protected dienes, which were transformed into the corresponding tetrahydropyridines only in moderate yields, thus indicating an influence of the N-protecting group on the outcome of the reaction. After RCM, the endocyclic double bond can be exploited for different functionalizations and a large variety of artificial sugars and iminosugars such as isofagomine could be accessible.
Davies and co-workers showed143 the efficiency of Grubbs’ catalyst I over the others (Nugent catalyst and Schrock catalyst) for the synthesis of N-protected heterocyclic amino acids and amines. The protocol is based on the diastereoselective conjugate addition of lithium (S)-N-allyl-N-α-methylbenzylamide to a range of α,β-unsaturated esters 208 followed by ring closing metathesis of β-amino esters 209 with Grubbs’ catalyst I to furnish desired N-α-methylbenzyl protected pyrrolidine β-amino ester and piperidine β-amino ester 210a-c as single diastereomer in each case (Scheme 49).
The utility of a number of dienyl imidazoles in ring-closing metathesis reaction with Grubbs’ 2nd generation ruthenium carbene complex, provided that the imidazole N-3 atom should remain protonated which was demonstrated by Lovely et al.144 The substrates 211a,b successfully underwent RCM reaction to afford the cyclic six-membered products 212 and 213, respectively. Surprisingly, the 4,5 allyl vinyl system did not participate in the metathesis, but 1,5 linked congeners readily provided the cyclic products. Steric effect of 4-iodo-substituent might be important since its removal provided a substrate that participated in the metathesis but with reduced efficiency (Scheme 50).
Chiral non-recemic cyanohydrins are proven expedient starting materials for the synthesis of several classes of compounds. Brussee et al. recently reported145 the synthesis of a set of new chiral unsaturated N-heterocycles via ring-closing metathesis. Tetrahydropyridines 215 were obtained ring-closing metathesis reaction of N-protected bis-olefinic secondary amine 214 with Grubbs’ catalyst I in refluxing CH2Cl2. The smooth transformation of 214 into 215 is remarkable as the Grubbs’ catalyst I is generally ineffective for the conversion of tertiary amines (Scheme 51).
Tandem Morita-Baylis-Hillman and ring-closing metathesis reaction sequence as a high yielding method for the construction of highly functionalized heterocyclic sulfonamides 217 with high efficiency was utilized by Krafft et al. (Scheme 52).146
A new synthetic entry to the 1,4-dihydroquinoline nucleus 220 was reported147 by Bennasar et al. The synthetic strategy involved the amide olefination-enamide RCM sequence for the construction of dihydroquinolines 220 (Scheme 53).
Organic compounds containing the quinolizidine skeleton are widespread in naturally occurring alkaloids and in biologically active compounds.148 Recently, Sόsnicki reported149 a new strategy to the synthesis of quinolizidine skeleton with olefinic bonds in both the rings. The substrate 222 was subjected to ring-closing metathesis in a sequential fashion to achieve the desired tetrahydroquinolizin-4-ones 223 (Scheme 54).
Gracias et al. recently developed150 a two-step reaction sequence using a one-pot α-aminoallylation reaction followed by the RCM reaction to afford spirocyclic diamines (Scheme 55). Treatment of the secondary amines 190 with 1.0 equiv of p-TsOH in CH2Cl2 for 30 min at reflux followed by the addition of the 5 mol% Grubbs’ catalyst II gave the desired spirocyclic diamines 227 in excellent yield. In absence of the pretreatment with p-TsOH the reaction failed to undergo ring closure.
Very recently, another method for the synthesis of trifluoromethyl-containing piperidines 229 by ring-closing metathesis has been developed by Rutjes et al.151 (Scheme 56).
Attempts to cyclize 228a to tetrahydropyridine 229a failed under different RCM conditions, probably due to the presence of basic amine functionality. However, substrates 228b,c were readily cyclized to the corresponding trifluoromethyl substituted cyclic nitrogen heterocycles 229b and 229c, respectively.
Similarly, some other substrates 230 were designed and subjected to the same RCM conditions to prepare the corresponding trifluoromethyl substituted tetrahydropyridine derivatives 231 in good-to-excellent yields (Scheme 57). This methodology was also successful towards the synthesis of some cyclic amino acid derivatives in moderate yields.
Very recently, Bhattacharjya et al. reported152 an important strategy for the synthesis of azabicyclic ring systems starting from a carbohydrate derivative (Scheme 58). Furanoside proved to be a versatile precursor for the synthesis of differently substituted indolizidine and pyrrolo[1,2-a]azepine skeleton.
The diacetate 232 smoothly underwent ring-closing metathesis with the Grubbs catalyst I to provide the indolizidine derivative 233 in 82% yield. Hydrogenation of 233 followed by acetylation gave the final compound (-)8-epi-swainsonine triacetate 234.
Recently, Arisawa et al. conducted153 a thorough synthetic study of nitrogen containing heterocycles using RCM, such as chiral bicyclic lactams, 1,2-dihydroquinolines, 2-quinolines and indoles including the development of silyl-enol-ether ene metathesis and isomerization of the terminal olefins. This methodology was applied to the synthesis of (-)-coniceine 235, (+)-angustureine 236 and also for the determination of absolute configuration of the angustureine (Figure 8).
Chang et al. recently accomplished154 the synthesis of α-conhydrine from trans-(2S,4R)-4-hydroxyproline via diastereoselective Grignard addition, regioselective Bayer-Villiger reaction and ring-closing metathesis of 237 to 238 as the key step (Scheme 59).
Recently, Weinreb and co-workers reported155 the total synthesis of the cylindricine B 243, a pyridoquinoline subclass of tricyclic marine ascidian alkaloids. The key steps involved the N-acylketiminium ion / olefin hetero-Diels-Alder reaction and ring-closing metathesis of vinyl chlorides (Scheme 60).
Highly functionalized tetracyclic intermediate of pentacyclic indole alkaloid mitralactonine 246 was conveniently synthesized156 by Chavan et al. employing RCM as the pivotal step for the construction of the D-ring (Scheme 61). The diene precursor 244 was subjected to 10 mol% Grubbs’ catalyst II in toluene at 800C for 3 h to afford the desired cyclized product 245 in excellent yield. Easy functionalization of this tetracyclic unit finally gave the indole alkaloid mitralactonine 246.
Savignac and Genêt described157 the synthesis of new tricyclic β-lactams 251 and 252 via one-pot enyne metathesis and Diels-Alder reaction (Scheme 62).
Ring-closing enyne metathesis (RCEM) of 249 with 5 mol% of Grubbs’ catalyst I afforded the bicyclic diene compound 250 which upon treatment with dimethylacetylenedicarboxylate (DMAD) gave cycloadducts 251 and 252. Interestingly, one-pot metathesis-cycloaddition of the enyne 251 gave better yield of the cycloadducts, compared to stepwise procedure.
Pérez-Castells and co-workers158 made an exhaustive study on the reactivity of different generations of catalysts on the enyne metathesis reaction course. Substrate 253 was treated with different catalysts in CH2Cl2/toluene to give different results. Grubbs’ catalyst I afforded the highest yield (95%) of the 4-vinyl-1,2-dihydroquinoline 254. The reaction was incomplete with Grubbs’ catalyst II whereas catalyst E furnished the cyclized product 254 in 70% yield. This study was also extended to the synthesis of benzazepine from N-acetyl,N-homoallyl-2-prop-2-ynyl benzene (Scheme 63).
It is quite clear that Grubbs’ catalyst II was much more sensitive towards steric hindrance as it improved the yield of the cyclized product with less hindered substrate 263. It should also be noted that neither Grubbs’ catalyst I nor catalyst II were able to catalyze the cyclization of the substrate 255 having double bond conjugated with the aromatic ring. On the other hand such type of compounds 255 underwent complete conversion with 5 mol% Hoveyda-Grubbs’ catalyst E (Scheme 64).
This methodology was also applied to the synthesis of indole nucleus with Hoveyda-Grubbs’ catalyst (E). The reactivity of the most popular ruthenium complexes were studied but there is no exact rule for their preferintial use although Grubbs’ catalyst II gave better results with monosubstituted olefins and worse with hindered substrates.
Synthesis of a variety of N-protected cyclic α-aminoboronates159 259a-g was achieved by Carboni et al. that could be further engaged in boropeptide synthesis. Ruthenium catalyst I was found to be effective for six-membered ring α-amidoboronic esters in 40-90% isolated yield in CH2Cl2 at reflux for 4 h. Grubbs’ catalyst I or II is unable to cyclize compound 257a (Scheme 65).
Six-membered heterocycles containing an N-N bond possess significant biological activity. Piperazic acid and 1-azafagomine are well known six-membered compounds with cyclic hydrazine skeleton. Although a number of methods have been reported for the synthesis of six-membered cyclic hydrazine,160 Tae and coworkers synthesized161 cyclic hydrazine 261 by ring closing metathesis of dienes and enynes tethered by an N-N bond (Scheme 66).
Cyclic dienamides are important skeletons of natural products and biologically active substances. Very recently Mori et al. synthesized162 a number of cyclic dienamides 264 using ruthenium catalyzed ring-closing metathesis of ene-ynamides 263. Substituents on the alkyne part (i.e. Ph group or an electron withdrawing group) were well tolerated under this reaction condition. The cyclic dienamides 264 are also good precursor towards the Diels-Alder reaction and afforded an indole or isoquinoline derivatives under mild conditions in high yield (Scheme 67).
Recently, a new approach to 1- and 2-vinyl substituted 3,4-dihydroquinolizinium cation by ring-closing enyne metathesis (RCEM) reaction from appropriately substituted pyridinium substrates under an atmosphere of ethylene and using the Hoveyda-Grubbs’ catalyst (D) was reported by Cuadro and Vaquero.163 Ring-closing enyne metathesis of the enyne 265 with Hoveyda-Grubbs’ catalyst D in CH2Cl2 gave the desired 2-vinyl-3,4-dihydroquinolizinium salt 266 in 83% yield. Substitution on the vinyl moiety of substrates 265 resulted in failure of the RCEM reaction and formation of cross metathesis products (Scheme 68).
Allyl-homoallyl adducts in the presence of 5-10 mol% Grubbs’ catalyst I easily reacted at room temperature to afford the expected dihydropiperidine derivative. However, RCM of N-methallylamines proceeded under reflux and with a longer reaction time. Homopropargyl allylamine 231a did not undergo cyclization even under reflux condition or with the use of Grubbs’ catalyst II (Scheme 69).164
4.3 SYNTHESIS OF FIVE- AND SIX-MEMBERED LACTAMS
Vinyl fluorides are versatile building blocks and are widely used in organic and medicinal chemistry e.g. isosteres of peptides and amides.165
Very recently, Fustero et al. reported166 a new example of the tandem RCM-isomerization reaction that allowed the efficient preparation of fluorinated and non-fluorinated unsaturated lactam derivatives of various sizes. Grubbs’ catalyst II in refluxing toluene is likely to generate a ruthenium hydride species59 in situ, without any use of additives which may in turn be responsible for the isomerization reaction. The presence of gem-difluoro moiety in the starting materials also exerted a pivotal effect by directing the isomerization step, making the overall transformation a regioselective process (Scheme 70).
Grubbs and co-workers167 reported their unsuccessful attempts to cross metathesis reaction of vinyl halides and olefins. The first ring closing metathesis of alkenyl α-fluoroacrylamides incorporating a fluorinated double bond was successfully reported by Haufe and co-workers.100 They showed that vinyl fluorides that are a part of an α,β-unsaturated carbonyl system to be more suitable for RCM reaction. A number of terminal N-alkenyl-N-benzyl-α-fluoroacrylamides 271 were synthesized by acylation with α-fluoroacrylic acid. Several N-alkenyl-α-fluoroacrylamides 271 were cyclized in the presence of 2 mol% of Grubbs’ catalyst II at room temperature to form an unsaturated γ-lactam 272a and at 800C to form the corresponding δ-lactam 272b,c bearing the fluoro vinyl moiety in 76-86% yields. Under the same conditions corresponding seven- and eight-membered N-heterocycles were not formed (Scheme 71).
Manzoni et al. reported168 the first example of ring-closing metathesis of dehydroamino acid for the construction of bicylic lactams. It was observed that both the steric and electronic factors of the N-protecting group played an important role to the behavior of ring-closing metathesis reaction.
Low yields of metathesis were obtained when the sterically bulky amino acid moiety was hindering the approach of the catalyst ruthenium species to the double bond of the substrate. Substrate 273c, when subjected to Grubbs’ catalyst II at 1000C in toluene for 72 h gave the cyclized product 274c in 53% yield along with the bicylic lactam 274a (14%) due to isomerisation of double bond followed by ring closure of the isomerized intermediate (Scheme 72).
Hiemstra and Rutjes demonstrated169 the usefulness of α-vinyl tetrahydroisoquinolines and tetrahydro-1H-β-carboline systems for the construction of heterocycles via ring-closing metathesis reaction. Sterically more hindered and rigid characteristics of the vinyl moiety compared to the flexible allyl moiety make this functionality less attractive for the metathesis reaction. However, they showed that vinyl moiety could be constructively used in RCM (Scheme 73).
Both the tertiary amines 276a,b failed to undergo metathesis reaction. However the amides 276c,d showed good conversion to the metathesis products 277c,d. 5,6-bicyclic systems 277c was formed in good yield but isolation of the product was impossible due to rapid decomposition via aerial oxidation. Best result was obtained with the longest alkenyl chain on nitrogen atom. The metathesis precursor 278 on treatment with Grubbs’ catalyst II in toluene at 800C afforded the metathesis product but could not be isolated due to aerial oxidation (Scheme 74).
The stereoselective total synthesis of novel quinolizidiene alkaloid (+)-epiquinamide was presented by Blaauw et al.170 starting from amino acid L-allysine ethylene acetal. Key steps in the synthesis involved highly diastereoselective N-acyliminium ion allylation and a ring closing metathesis reaction to provide the bicyclic skeleton.
A stereocontrolled total synthesis of an alkaloid (-)-250B (286), isolated from the skin of neotropical poison-frogs, was reported171 recently by Smith III et al. employing a dithiane three component linchpin coupling, a one pot sequential construction of the embedded indolizidine ring and ring closing metathesis reaction of 284 to 285 (Scheme 75).
A concise and practical catalytic asymmetric synthesis of (-)-CP-99,994 and (-)-L-733,061 was achieved by Nakano et al.172 Key steps for these syntheses involved the Pd-catalyzed asymmetric allylic amination and a ring-closing metathesis reaction of 289 to 290 (Scheme 76).
Ring-closing metathesis reaction sometimes accompanied by variable amounts of the other compounds that arise as a result of isomerization of the newly created double bond.60,173 Several reports indicated that the decomposition products of Ru-metathesis catalyst (generally Ru-hydride complexes) may be responsible for these side reactions and especially for alkene isomerization. Although the side reactions constitute a general problem but it is also true that several authors in specific synthetic strategies used them advantageously.
Martin group recently developed174 a novel approach to a variety of indole alkaloids from a key intermediate 294 by employing a sequence of two RCM reactions and one zirconocene-catalyzed carbomagnesation. A variety of substituents at C (15) in 294, can be installed by exploring the 1,4-conjugate addition of various nucleophiles onto α,β-unsaturated lactams to complete the concise total synthesis of corynanthe group of indole alkaloid 295 and also the formal synthesis of other indole alkaloids such as 296, 297 and 298 (Figure 9).
5. SYNTHESIS OF NITROGEN AND OXYGEN HETEROCYCLES BY DOMINO PROCESS VIA RCM
Tandem catalysis can offer unique and powerful strategies for converting simple starting materials into more complex products in a single step generating less waste and minimizing the excessive handling of multistep process. In this regard, ruthenium catalyst i.e. Grubbs’ catalysts are shown to catalyze two mechanistically distinct transformations to offer unique protocol that effects multiple bond changes in a single operation.
5.1 SYNTHESIS OF HETEROCYCLES BY ROM / RCM STRATEGY
An efficient stereoselective route to the synthesis of dihydrofuropyran skeleton starting from furan using ring-opening, cross and ring-closing metathesis approach was recently described175 by Plumet et al. The RCM precursor 303on treatment with Hoveyda-Grubbs catalyst E (10 mol%) in anhydrous CH2Cl2 under ethylene atmosphere afforded the desired furanopyrone 304 in 90% yield (Scheme 77).
Among the different types of metathesis reactions, diastereoselective ring rearrangement metathesis (dRRM) is especially powerful in the asymmetric synthesis leading to various natural products. Blechert et al. recently reported176 the stereoselective synthesis of 2,6-disubstituted dihydropyrans to the total synthesis of (-)-centrolobine. During the study of dRRM, different conditions were employed and in the optimized procedure, the dRRM reaction was carried out using Grubbs’ catalyst II in benzene, saturated ethylene atmosphere at 500C in a pressure vessel to furnish the desired product 306. Isomerization of the dRRM product with 40 mol% NaBH4 to dihydropyran without affecting the endocyclic double bond followed by cross-metathesis finally afforded the targeted molecule (-)-centrolobine 309 (Scheme 78).
Ring rearrangement metathesis has already proven to be a powerful tool for the synthesis of complex molecules. An interesting methodology to create a new stereogenic centre by RCM was recently reported177 by Blechert et al. They have envisaged that cyclodienes such as 311 would rearrange to give products with an internal double bond in the side chain, However, 311 was converted into the dihydropyran 312 with a diastereomeric mixture 7:2 in favor of the 2,6-cis product. Similarly, cyclopentene derivatives of carbamate 313 underwent rearrangement most effectively with Hoveyda catalyst C (2 mol%) in 89% yields and good diastereoselectivity (5:1) to give the nitrogen heterocycles (Scheme 79).
Rainier et al. also recently utilized178 7-azanorbornenes for the synthesis of hexahyrdroindoline derivatives 316 via ring opening / ring closing metathesis upon treatment with Grubbs’ catalyst II (Scheme 80).
Blechert et al. reported179 the first enantioselective synthesis of naturally occurring alkaloid (+)-dumetorine by the application of ring rearrangement metathesis strategy to convert the intermediate 329 to 322 (Scheme 81).
A tandem metathesis approach has been reported180 for the synthesis of stereochemically defined tri- and penta-heterocyclic ring system by Winkler et al. The RCM precursor 325 on exposure to Grubbs’ catalyst II, in ethylene atmosphere initially afforded a ring opening of oxanorbornanene then the six-membered tetrahydropyridine ring intermediate and finally a tricyclic heterocycle 328. In a similar manner the pentacyclic heterocycle 329 have also been synthesized starting from the basic unit 323 (Scheme 82).
Ring opening metathesis (ROM)-ring closing metathesis (RCM) is a very attractive method for the synthesis of bicyclic compounds.181,182 Utilizing this methodology, Mori et al. synthesized183 isoquinoline derivatives from cyclobutenylmethyl amine derivatives having an alkenyl moiety in a tether using Grubbs’ 2nd generation ruthenium carbene complex under ethylene gas. When cyclobutenylmethylamines and cyclobutenecarboxamides 330a-d were treated with 5-10 mol% of Grubbs’ catalyst II under suitable condition afforded the desired isoquinoline and isoquinolone derivatives 331a-d in good yields respectively (Scheme 83).
5.2 SYNTHESIS OF HETEROCYCLES BY TANDEM RCM
Α pyrone subunit is found to be present in a large number of natural products. Similarly, butenolide is also a framework of many compounds having interesting biological activity. A direct synthesis of butenolides and β,γ-unsaturated δ-lactones has been devised184 by Piva et al. by combining a ring closing metathesis with cross-metathesis process.
Recently, Piva et al. developed an efficient method for the synthesis of pyrones by a tandem ring-closing-cross-coupling metathesis reaction.185 The approach was based first on a RCM process of 3-O-(1,4-pentadienyl)3-butenoate 332 promoted by Grubbs’ catalyst II to form compound 334 as an intermediate. In the presence of various alkenes and with the same catalyst, 334a was converted into functionalized lactones 334b which were isomerised into α-pyrones 334c by treatment with a base such as DBU (Scheme 84).186 The first step of the reaction generates a new carbene group, which is capable of functionalizing the lateral unsaturated chain during the second coupling reaction. Following this route, the transfer of the alkyl group, initially fixed on the acid chain, avoids the use of an alkene partner introduced in large excess. This method also avoids the use of toxic acrylic esters.
Conjugated esters 298 were converted directly into five-membered ring lactones 338 without the introduction of an additional alkene as a counterpart in the reaction media (Scheme 85).186
A tandem enyne/ring-closing metathesis approach on a sugar template leading to a novel angularly fused dioxatriquinane was achieved187,188 from a readily available ketone 339. The precursor dienyne 342 on treatment with Grubbs’ catalyst I or II afforded the RCM product 343 exclusively. The dioxa-triquinanes skeleton 344 was achieved by removing the acetonide group from the precursor 342 which in turn could probably bring the two double bonds closer after the initial enyne metathesis to relief the ring strain and protecting the hydroxyl group as acetate and finally with Grubbs’ catalyst I / II under different reaction conditions (Scheme 86).
An interesting multiple RCM reaction i.e. quadruple ring-closing metathesis strategy has been developed189 by Wallace (Scheme 87).
A novel synthesis190 of enantiopure (+)-N-Boc-norpandamarilactone-A, a part of Pandanus variety distributed in tropical and subtropical regions used in traditional folk medicine for strengthening the heart and hypoglecamic purpose was reported recently by Honda et al. A double ring closing metathesis is the key step where two five membered heterocyclic rings were constructed in one step. The RCM precursor triene 352 was prepared by a sequence of reaction starting from L-serine. Examining a variety of reaction conditions for compound 352, they have found among several catalysts (Grubbs’ catalyst I, Hoveyda catalyst and Grela catalyst) Grela catalyst was proved to be most effective one in 10 mol% which afforded the targeted molecule 353 in 76% yield (Scheme 88). The bis-five-membered product 353 is supposed to be a kinetically controlled product.
Harrity et al. recently reported191 the stereo-controlled synthesis of functionalized spiro-piperidine through a diastereoselective tandem RCM reaction. The tetraene 356 was subjected to the tandem RCM reaction in CH2Cl2 and toluene at elevated temperature using Grubbs’ catalyst II to form spiropiperidine in excellent yield, but with an almost equal mixture of diastereomers. The diastereoselectivity could be improved by subjecting the tetraene substrate to less active Grubbs’ catalyst I (10 mol%) in refluxing CH2Cl2 to provide the spiropiperidine 358 in high yield and in 12:1 diastereomeric ratio. Diastereoslectivity, therefore strongly depends on the nature of the catalyst used in the reaction medium (Scheme 89).
A diastereoselective total synthesis of (-)-securinine, a naturally occurring securinega alkaloid in optically pure form was achieved192 by ring closing metathesis strategy as one of the key steps from the corresponding dienynes. The dienyne 363 was treated with highly active ruthenium catalyst (Grubbs’-Hoveyda catalyst D) in CH2Cl2 to furnish the cyclized product 364 and accomplishment of the total synthesis of (-) securinine 365 was completed in a sequence of reactions, oxidation at the allylic position followed by bromination, deprotection with TFA and subsequent cyclization with K2CO3 (Scheme 90).
Additionally further functionalization of the spiropiperidine was carried out through a regio-stereoselctive dihydroxylation reaction employing Donohe’s OsO4-TMEDA conditions (Scheme 91). This tandem RCM-dihydroxylation sequence had successfully set three of the four-stereogenic centres required for elaboration to histrionicotoxin target.
Fused bicyclic lactams having quinolizidine alkaloid skeleton are common structural units of many important compounds displaying a broad range of biological activities.193 Recently, Ma and co-workers reported194 a highly stereoselective synthesis of bicyclic quinolizidine alkaloids and their analogues bearing 1,3-diene moiety via tandem ring closing metathesis reaction of N-alkenyl-(1,ω)-alkedienyl acrylamides using Grubbs’ catalyst I or II. The excellent stereoselectivity of the fused/dumbbell-mode cyclization has been realized by the higher reactivity of the electron rich –C=C_ bond or carbon-carbon triple bond combined with the lower reactivity of the electron deficient C=C towards metallocarbenes and the thermodynamically more stable nature of the fused-bicyclic compounds versus dumbbell-type bicyclic compounds. The RCM precursors, N-containing trienynes 369a-f were subjected to tandem RCM using 5 mol% of Grubbs’ catalyst I or II in refluxing CH2Cl2 for 7-11 h to afford the desired fused bicyclic lactams that are suitable substrates for the preparation of polycyclic quinolizidine and other alkaloid derivatives via Diels-Alder reaction (Scheme 92).
Erythrina alkaloids are well known owing to their interesting biological activity. The first total synthesis195 of (+)-β-erythroidine, a non-aromatic erythrina alkaloid, in naturally occurring form by employing Lewis acid promoted cyclization of the dienynes 379a,b as the key step. This work opened up a new avenue for the enantioselective synthesis of erythrina alkaloids (Scheme 93).
Mori and co-workers recently described196 the total synthesis of erythrocarine 390 by using a number of important reaction sequences and the ring-closing metathesis of dienyne as the key step. The reaction of 381 and trimethylsilylacetylene in the presence of palladium catalyst gave the alkyne 382 which was then condensed with nitromethane to afford 383. Treatment of 383 with LiAlH4 followed by Boc-protection afforded the corresponding alkyne 384. The RCM precursors 388 were synthesized from compound 384 by the application of a number of reaction sequences. When a CH2Cl2 solution of dienyne hydrochloride was treated with Grubbs’ 1st generation catalyst (10 mol%) at rt for 18 h, the corresponding cyclized product 389 was obtained in almost quantitative yield. Treatment of 389a with K2CO3 in MeOH gave the corresponding alcohol, erythrocarine 390 (Scheme 94).
The first total synthesis of (±)-erythravine was achieved197 in thirteen steps from 3,4-dimethoxyphenethylamine 391 using ring-closing dienyne metathesis as the key step by Hatakeyama et al. 3,4-Dimethoxyphenethylamine 391 was initially converted to its Boc-protected-N-allyl derivative 392, which was then reacted with diethylpropiolate in boiling TFA acid to give the diester 394 via Pictet-Spengler type of reaction. Subsequent reduction of compound 394 with LiAlH4 followed by selective silylation afforded TBDPS-ether 395. The enyne 396 was synthesized by Swern oxidation of the compound 395. Upon sequential desilylation, Swern oxidation and Grignard reaction using vinylmagnesium bromide, 396 gave dienyne 397a and 397aa as a 1:1 epimeric mixture. For the next ring-closing metathesis reaction, acetate 397a and TES-ether 397aa were also prepared each as a 1:1 epimeric mixture from 396. When the precursor 397a was treated with Grubbs’ catalyst in refluxing CH2Cl2 for 8 h, afforded the corresponding tetracyclic compounds 398a and 398aa in a ratio of 63:37 in 78% yield. (±)-Erythravine 399 was finally obtained from 389a treatment with K2CO3 in methanol (Scheme 95).
6. MISCELLANEOUS
Construction of heterocyclic ring system by ring-closing metathesis has also been extensively explored as an annulation strategy. Weinreb et al. reported198 the synthesis of six-membered heterocyclic system from the corresponding vinyl chlorides by ring-closing metathesis utilizing Grubbs’ catalyst II. This protocol also provides five- and seven-membered heterocycles as well as six-membered carbocycles (Scheme 96).
The utility of some ruthenium complexes in catalytic processes has been extended beyond olefin metathesis to other synthetic transformation. One of the most striking example is the one-pot synthesis of (R)-(-)-muscone reported by Grubbs199 in 2001 in three steps viz. RCM, transfer dehydrogenation and hydrogenation catalyzed by a single ruthenium source. Snapper et al.200 as well as Schmidt et al.201 have also reported the synthesis of cyclic enols by tandem RCM / olefin isomerization. A new process combining a metathesis step utilizing Grubbs’ catalyst I and a dihydroxylation step has been developed by Blechert et al.202 for the synthesis of a variety of dihydroxylated products. Owing to the high tollerence of functional groups, this protocol has an interesting potential for application in natural product synthesis (Scheme 97). The process also presents an alternative to the pinacol coupling. The combination of two catalytic processes depending on a single ruthenium source, in addition to low catalyst loading makes this reaction particularly useful.
Spino et al. reported203 the selective tandem Mitsunobu/[3,3] sigmatropic rearrangement of hydrazoic acid that produces enantiopure allylic azide, which provides nitrogen heterocycles by applying ring-closing metathesis. The ring closing metathesis of the precursor 406 was performed by using Grubbs’ or Nolan catalyst in refluxing CH2Cl2 to give nitrogen and oxygen heterocycles (Scheme 98). This protocol was also successfully utilized for the short and highly selective synthesis of α-amino acids and nitrogen heterocycles. This excellent regioselectivity of the azide rearrangement and the efficient cleavage of the chiral auxiliary by RCM is notable.
An efficient method for the synthesis of furan and pyrrole skeleton from the Baylis-Hillman adduct by ring closing metathesis was reported204 by Kim et al. (Scheme 99).
Isomerization-RCM strategy has recently been proved to be an excellent method for the formation of small to medium size ring heterocycles. Utilizing this strategy, van Otterlo reported205 the synthesis of benzo-fused six-membered heterocycles 412 by Grubbs’ catalyst II starting from aminophenol. However, benzofused eight-membered heterocycles were obtained from 411 by using Grubbs’ catalyst II (5 mol%) (Scheme 100).
Isomerization of the diallyl compounds followed by ring closing metathesis reaction afforded the 4H-benzo-[1,4]-oxazines in good yields, although ring closing metathesis reaction using Grubbs’ catalyst on the substrates containing electron rich vinylic olefins is known to be problematic.
One of the most interesting disclosures concerning the enyne metathesis is the formation of 2,3-di and 2,3,4-trisustituted furans from 1,2-dioxines.206 The methodology is based on the enyne-RCM/Diels-Alder reaction sequence. The reaction conditions for the transformation of 1,2-dioxines into furans are delineated in Scheme 101. Compounds 413a and 413b with the Grubbs’ catalyst I in refluxing dichloromethane yielded compounds 414a and 414b. Cycloaddition reaction of the dienes 414a and 414b with singlet oxygen followed by FeSO4-catalyzed reaction led to the corresponding substituted furan 415a and 415b, respectively (Scheme 101).
Grubbs’ carbene complexes, which are easily associated with metathesis chemistry, also proved to be efficient catalyst for the promotion of other intramolecular reactions depending upon the reaction conditions. These non-metathetical activities were mostly due to the in situ decomposition of the initial carbene complex to an uncharacterized ruthenium complex.59,207-209 Recently, Quayle and co-workers reported210,211 that sequential ring closing metathesis (RCM)-Kharasch cyclization212,213 could be promoted by the use of Grubbs’ catalysts I and II to provide rapid access to bicyclic lactones and lactams (Scheme 102).
Treatment of the substrate 416 with Grubbs’ catalyst I (5 mol% in degassed toluene) first at ambient temperature for 3 h then under mild thermolysis at 1100C afforded directly lactone 419 as the major product, however repeating the reaction with 2nd generation Grubbs’ catalyst resulted the isolation of a lactone 418 in 62% yield as the major product, indicating that the product distribution is solely dependent upon the catalyst employed in the reaction. This sequential RCM-Kharasch reaction was also found to be effective for the synthesis of bicyclic lactams 421 (Scheme 103).
7. CONCLUSIONS
The results and examples discussed above demonstrate that olefin metathesis has proven to be an efficient method, especially for the preparation of different ring-sized compounds with varying complexity. RCM catalysts display the scope and activity required for a generally useful synthetic approach to complex molecules. With the advent of well-defined metathesis catalysts the early promise of olefin metathesis as a new synthetic strategy is being realized and numerous new aspects of this chemistry have been discovered. Ring-closing metathesis reactions are simple, high yielding and truly friendly to the environment. A number of applications of RCM to the synthesis of complex and highly functionalized organic molecules, various regular-sized heterocycles, lactam and lactone containing natural products have been presented. The successful application of the olefin metathesis reaction hinges on the selectivity of the carbon-carbon bond-forming reaction and cross metathesis reaction. Improved catalyst for specific applications, including enantioselective synthesis has been developed and will continue to be developed. Metathesis is presently used by the entire organic community because of its extraordinary potential for the synthesis of molecular target. Application of this area in organic synthesis will continue to grow, as we can count on the future development of even more active and functional group tolerant metal complexes. The future holds considerable promise for more advances and applications of RCM / RCEM not only in heterocyclic chemistry but in other arenas as well. Metathesis now is at the forefront of green chemistry by providing shorter synthetic routes and more facile access to the natural products and therapeutic agents under more environmentally friendly catalytic conditions. We hope that this review article will be useful to the synthetic chemists and especially to those who work with heterocyclic compounds.
8. ACKNOWLEDGEMENTS
We thank to CSIR (New Delhi) for financial support. S.M, R.I and B.C are also thankful to CSIR (New Delhi) for their senior research fellowships.
9. ABBREVIATIONS
RCM, Ring-closing metathesis; RCEM, Ring-closing enyne metathesis; ROM, ring opening metathesis; CH2Cl2, Dichloromethane; Bn, Benzyl; Boc, Tertiary-butyloxycarbonyl; PhH, Benzene; Ac2O, Acetic anhydride; THF, Tetrahydrofuran; DMAP, Dimethylaminopyridine; Pyr, Pyridine; DCC, Dicyclohexylcarbodiimide; Et3N, Triethylamine; LHMDS, lithium hexamethyldisilazide; KHMDS, Potassium bis(trimethylsilyl)amide; DBU, 1,8-Diazabicyclo[5.4.0]undec-7-ene; TMSCl, Trimethylsilyl chloride; TBDPS, tert-Butyl(chloro)diphenylsilane; PCC, Pyridinium chlorochromate; MOM, Methoxymethyl; TsOH, p-Toluenesulfonic acid; AcOH, Acetic acid; TFAA, Trifluoroacetic anhydride; LiAlH4, Lithium aluminium hydride; NaBH4, Sodium borohydride; NaCNBH3, Sodium cyanoborohydride; Cp2TiMe2, Dicyclopentadienyl dimethyl titanocene; MeCN, Acetonitrile; TFA, Trifluoroacetic acid; Dppp, Diphenyl phosphinopropane; NMO, N-methyl morpholine oxide; CM, Cross metathesis; RRM , ring rarrangement metathesis; dRRM, diastereoselsective ring rearrangement metathesis; TCQ, tetrachloroquinone.
References
1. (a) G. C. Fu and R. H. Grubbs, J. Am. Chem. Soc., 1992, 114, 5426; CrossRef (b) G. C. Fu, S. T. Nguyen, and R. H. Grubbs, J. Am. Chem. Soc., 1993, 115, 9856. CrossRef
2. N. Calderon, Acc. Chem. Res., 1972, 5, 127. CrossRef
3. J.-L. Herisson and Y. Chauvin, Makromol. Chem., 1971, 141, 161. CrossRef
4. R. H. Grubbs, D. D. Carr, C. Hoppin, and P. L. Burk, J. Am. Chem. Soc., 1976, 98, 3478. CrossRef
5. G. C. Lloyd-Jones, R. G. Margue, and J. G. de Vries, Angew. Chem. Int. Ed., 2005, 44, 7442. CrossRef
6. K. C. Majumdar, R. Islam, H. Rahaman, and B. Roy, Org. Biomol. Chem., 2006, 4, 2393. CrossRef
7. K. C. Majumdar, H. Rahaman, S. Muhuri, and B. Roy, Synlett, 2006, 466. CrossRef
8. K. C. Majumdar, H. Rahaman, R. Islam, and B. Roy, Tetrahedron Lett., 2006, 47, 2111. CrossRef
9. D. J. Wallace, Angew. Chem. Int. Ed., 2005, 44, 1912. CrossRef
10. B. Kang, D.-H. Kim, Y. Do, and S. Chang, Org Lett., 2003, 5, 3041. CrossRef
11. F. Dolhem, C. Lièvre, and G. Demailly, Eur. J. Org. Chem., 2003, 2336. CrossRef
12. C. S. Poulsen and R. Madsen, J. Org. Chem., 2002, 67, 4441. CrossRef
13. N. Saito, Y. Sato, and M. Mori, Org. Lett., 2002, 4, 803. CrossRef
14. D. L. Wright, J. P. Schulte, and M. A. Page, Org. Lett., 2000, 2, 1847. CrossRef
15. R. J. Davoille, D. T. Rutherford, and S. D. R. Christie, Tetrahedron Lett., 2000, 41, 1255. CrossRef
16. G. Spagnol, M.-P. Heck, S. P. Nolan, and C. Mioskowski, Org. Lett., 2002, 4, 1767. CrossRef
17. A. Whitehead, J. D. Moore, and P. R. Hanson, Tetrahedron Lett., 2003, 44, 4275. CrossRef
18. S. T. Diver and A. J. Giessert, Chem. Rev., 2004, 104, 1317. CrossRef
19. A. H. Hoveyda, D. G. Gillingham, J. J. van Veldhuizen, O. Kataoka, S. B. Garber, J. S. Kingsbury, and J. P. Harrity, Org. Biomol. Chem., 2004, 2, 8. CrossRef
20. A. Gradillas and J. Pèrez-Castells, Angew. Chem. Int. Ed., 2006, 45, 6086. CrossRef
21. K. C. Nicolaou, P. G. Bulger, and D. Sarlah, Angew. Chem. Int. Ed., 2005, 44, 4490. CrossRef
22. D. Astruc, New J. Chem., 2005, 29, 42. CrossRef
23. R. H. Grubbs, Tetrahedron, 2004, 60, 7117. CrossRef
24. A. Deiters and S. F. Martin, Chem. Rev., 2004, 104, 2199. CrossRef
25. M. D. McReynolds, J. M. Dougherty, and P. R. Hanson, Chem. Rev., 2004, 104, 2239. CrossRef
26. G. C. Bazan, J. H. Oskam, H.-N. Cho, L. Y. Park, and R. R. Schrock, J. Am. Chem. Soc., 1991, 113, 6899. CrossRef
27. W. A. Herrmann, Angew. Chem. Int. Ed., 2002, 41, 1290. CrossRef
28. T. Weskamp, W. C. Schattenmann, and W. A. Herrmann, Angew. Chem. Int. Ed., 1998, 37, 2490. CrossRef
29. J. Huang, E. D. Stevens, S. P. Nolan, and J. L. Petersen, J. Am. Chem. Soc., 1999, 121, 2674. CrossRef
30. A. Furstner, L. Ackermann, B. Gabor, R. Goddard, C. W. Lehmann, R. Mynott, F. Stelzer, and O. R. Thiel, Chem. Eur. J., 2001, 7, 3236. CrossRef
31. J. S. Kingsbury, J. P. A. Harrity, P. J. Bonitatebus, and A. H. Hoveyda, J. Am. Chem. Soc., 1999, 121, 791. CrossRef
32. M. A. O. Volland, S. M. Hansen, F. Rominger, and P. Hofmann, Organometallics, 2004, 23, 800. CrossRef
33. S. J. Connon, A. M. Dunne, and S. Blechert, Angew. Chem. Int. Ed., 2002, 41, 3835. CrossRef
34. H. Wakamatsu and S. Blechert, Angew. Chem. Int. Ed., 2002, 41, 794. CrossRef
35. J. O. Krause, M. T. Zarka, U. Anders, R. Weberskirch, O. Nuyken, and M. R. Buchmeiser, Angew. Chem. Int. Ed., 2003, 42, 5965. CrossRef
36. T. Honda, H. Namiki, K. Kaneda, and H. Misutani, Org. Lett., 2004, 6, 87. CrossRef
37. A. Michrowska, R. Bujok, S. Harutyunyan, V. Sashuk, G. Dolgonos, and K. Grela, J. Am. Chem. Soc., 2004, 126, 9318. CrossRef
38. M. Bieniek, R. Bujok, H. Stepowska, A. Jacobi, R. Hagenkötter, D. Arlt, K. Jarzembska, A. Makal, K. Woźniak, and K. Grela, J. Organomet. Chem., 2006, 691, 5289. CrossRef
39. D. Ri, H. Clavier, Y. Coutard, L. Gulajski, K. Grela, and M. Mauduit, J. Organomet. Chem., 2006, 691, 5397. CrossRef
40. J. Yun, E. R. Marinez, and R. H. Grubbs, Organometallics, 2004, 23, 4172. CrossRef
41. T. Ung, A. Hejl, R. H. Grubbs, and Y. Schrodi, Organometallics, 2004, 23, 5399. CrossRef
42. T. W. Funk, J. M. Berlin, and R. H. Grubbs, J. Am. Chem. Soc., 2006, 128, 1840. CrossRef
43. L. Yang, M. Mayr, K. Wurst, and M. R. Buchmeiser, Chem. Eur. J., 2004, 10, 5761. CrossRef
44. H. Clavier, J. L. Petersen, and S. P. Nolan, J. Organomet. Chem., 2006, 691, 5444. CrossRef
45. J. C. Conrad, H. H. Parnas, J. L. Snelgrove, and D. E. Fogg, J. Am. Chem. Soc., 2005, 127, 11882. CrossRef
46. S. H. Hong and R. H. Grubbs, J. Am. Chem. Soc., 2006, 128, 3508. CrossRef
47. Q. Yao and M. Sheets, J. Organomet. Chem., 2005, 690, 3577. CrossRef
48. V. Martinez, J.-C. Blais, G. Bravis, and D. Austruc, Organometallics, 2004, 23, 861. CrossRef
49. B. S. Lee, S. K. Namgoong, and S.-G. Lee, Tetrahedron Lett., 2005, 46, 4501. CrossRef
50. Q. Yao and Y. Zhang, J. Am. Chem. Soc., 2004, 126, 74. CrossRef
51. D. E. A. Brittain, B. L. Gray, and S. L. Schreiber, Chem. Eur. J., 2005, 11, 508. CrossRef
52. F. Michalek, D. Madge, J. Ruhe, and W. Bannwarth, J. Organomet. Chem., 2006, 691, 5172. CrossRef
53. S. J. Connon, A. M. Dunee, and S. Blechert, Angew. Chem. Int. Ed., 2002, 41, 3835. CrossRef
54. M. R. Buchmeiser, D. Wang, S. Naumov, and K. Wurst, J. Organomet. Chem., 2006, 691, 5391. CrossRef
55. J. H. Cho and B. M. Kim, Org. Lett., 2003, 5, 531. CrossRef
56. K. Grela and M. Kim, Eur. J. Org. Chem., 2003, 963. CrossRef
57. A. Michrowska, L. Gulajski, and K. Grela, Chem. Commun., 2006, 841. CrossRef
58. V. Dragutan and I. Dragutan, J. Organomet. Chem., 2006, 691, 5129, and references cited therein. CrossRef
59. S. H. Hong, M. W. Day, and R. H. Grubbs, J. Am. Chem. Soc., 2004, 126, 7414. CrossRef
60. B. Schmidt, Eur. J. Org. Chem., 2004, 1865. CrossRef
61. Y. Terada, M. Arisawa, and A. Nishida, Angew. Chem. Int. Ed., 2004, 43, 4063. CrossRef
62. T. J. Donohoe, A. J. Orr, K. Gosby, and M. Bingham, Eur. J. Org. Chem., 2005, 1969. CrossRef
63. W. A. L. van Otterlo, G. L. Morgans, L. G. Madeley, S. Kuzvidza, S. S. Moleele, N. Thornton, and C. B. de Koning, Tetrahedron, 2005, 61, 7746. CrossRef
64. W. A. L. van Otterlo, E. L. Ngidi, and C. B. de Koning, Tetrahedron Lett., 2003, 44, 6483. CrossRef
65. I. Sánchez and M. D. Pujol, Synthesis, 2006, 1823. CrossRef
66. G. Prestat, C. Baylon, M.-P. Heck, G. A. Grasa, S. P. Nolan, and C. Mioskowski, J. Org. Chem., 2004, 69, 5770. CrossRef
67. K. J. Quinn, A. G. Smith, and C. M. Cammarano, Tetrahedron, 2007, 63, 4881. CrossRef
68. P. A. Evans, D. K. Leahy, W. J. Andrews, and D. Uraguchi, Angew. Chem. Int. Ed., 2004, 43, 4788. CrossRef
69. G. C. H. Chiang, A. D. Bond, A. Ayscough, G. Pain, S. Ducki, and A. B. Holmes, Chem. Commun., 2005, 1860. CrossRef
70. M. Etheve-Quelquejeu, J. Xie, and J.-M. Valéry, Synlett, 2004, 1089. CrossRef
71. S. Chang and R. H. Grubbs, J. Org. Chem., 1998, 63, 864. CrossRef
72. W. A. L. van Otterlo, E. L. Ngidi, S. Kuzvidza, G. L. Morgans, S. S. Moleele, and C. B. de Koning, Tetrahedron, 2005, 61, 9996. CrossRef
73. W. A. L. van Otterlo, E. L. Ngidi, and C. B. de Koning, Tetrahedron Lett., 2003, 44, 6483. CrossRef
74. W. A. L. van Otterlo, R. Pathak, and C. B. de Koning, Synlett, 2003, 1859. CrossRef
75. T. N. Van and N. De Kimpe, Tetrahedron Lett., 2004, 45, 3443. CrossRef
76. J. M. Adam, L. de Fays, M. Laguerre, and L. Ghosez, Tetrahedron, 2004, 60, 7325. CrossRef
77. A. K. Chaterjee, J. P. Morgan, M. Scholl, and R. H. Grubbs, J. Am. Chem. Soc., 2000, 122, 3783. CrossRef
78. W. A. L. van Otterlo, E. L. Ngidi, E. M. Coyanis, and C. B. de Koning, Tetrahedron Lett., 2003, 44, 311. CrossRef
79. A.-L. Lee, S. J. Malcolmson, A. Puglisi, R. R. Schrock, and A. H. Hoveyda, J. Am. Chem. Soc., 2006, 128, 5153. CrossRef
80. S. Basu and H. Waldmann, J. Org. Chem., 2006, 71, 3977. CrossRef
81. J. Liu and R. P. Hsung, Org. Lett., 2005, 7, 2273. CrossRef
82. S. K. Ghosh, C. Ko, J. Liu, J. Wang, and R. P. Hsung, Tetrahedron, 2006, 62, 10485. CrossRef
83. M. H. D. Postema, J. L. Piper, V. Komanduri, and L. Liu, Angew. Chem. Int. Ed., 2004, 43, 2915. CrossRef
84. M. H. D. Postema, J. L. Piper, R. L. Betts, F. A. Valeriote, and H. Pietraszkewicz, J. Org. Chem., 2005, 70, 829. CrossRef
85. S. Sahabuddin, A. Roy, M. G. B. Drew, B. G. Roy, B.Achari, and S. B. Mandal, J. Org. Chem., 2006, 71, 5980. CrossRef
86. K. Ohmori, Y. Ogawa, T. Obitsu, Y. Ishikawa, S. Nishiyama, and S. Yamamura, Angew. Chem. Int. Ed., 2000, 39, 2290. CrossRef
87. E. A. Voight, H. Seradj, P. A. Roethle, and S. D. Burke, Org. Lett., 2004, 6, 4045. CrossRef
88. M. T. Crimmins, Y. Zhang, and F. A. Diaz, Org. Lett., 2006, 8, 2369. CrossRef
89. B. Schmidt and S. Nave, J. Org. Chem., 2006, 71, 7364. CrossRef
90. B. Schmidt, J. Org. Chem., 2004, 69, 7672. CrossRef
91. J. S. Clark, Chem. Commun., 2006, 3571. CrossRef
92. U. Majumder, J. M. Cox, H. W. B. Johnson, and J. D. Rainier, Chem. Eur. J., 2006, 12, 1736. CrossRef
93. H. W. B. Johnson, U. Majumder, and J. D. Rainier, Chem. Eur. J., 2006, 12, 1747. CrossRef
94. J. S. Yadav, S. J. Prakash, and Y. Gangadhar, Tetrahedron:Asymmetry, 2005, 16, 2722. CrossRef
95. M. Bassetti, A. D. Annibale, A. Fanfoni, and F. Minissi, Org. Lett., 2005, 7, 1805. CrossRef
96. M. Fujii, M. Fukumura, Y. Hori, Y. Hirai, H. Akita, K. Nakamura, K. Toriizuka, and Y. Ida, Tetrahedron:Asymmetry, 2006, 17, 2292. CrossRef
97. A. K. Chaterjee, F. D. Toste, S. D. Goldberg, and R. H. Grubbs, Pure Appl. Chem., 2003, 75, 421. CrossRef
98. T. N. Van, S. Debenedetti, and N. De Kimpe, Tetrahedron Lett., 2003, 44, 4199. CrossRef
99. L. Polito, M. Cravini, L. Poletti, and L. Lay, Synth. Commun.,, 2006, 36, 2203. CrossRef
100. M. Marhold, A. Buer, H. Hiemstra, J. H. van Maarseveen, and G. Haufe, Tetrahedron Lett., 2004, 45, 57. CrossRef
101. S. Gowrisankar, K. Y. Lee, and J. N. Kim, Tetrahedron, 2006, 62, 4052. CrossRef
102. D.-W. Hahn, D.-M. Byun, and J. Tae, Eur. J. Org. Chem., 2005, 63. CrossRef
103. S. Michaelis and S. Blechert, Org. Lett., 2005, 5, 5513. CrossRef
104. J. Cossy, F. Bargiggia, and S. BouzBouz, Org. Lett., 2003, 5, 459. CrossRef
105. K. A. Hood, L. M. West, B. Rouwé, P. T. Northcote, M. V. Berridge, S. J. Wakefield, and J. H. Miller, Cancer Res., 2002, 62, 3356.
106. E. Roulland and M. S. Ermolenko, Org. Lett., 2005, 7, 2225. CrossRef
107. G. V. M. Sharma, A. Begum, K. R. Kumar, P. R. Krishna, A. Prabhakar, and A. C. Kunwar, Tetrahedron Lett., 2005, 46, 4131. CrossRef
108. J. S. Yadav, I. Prathap, and B. P. Tadi, Tetrahedron Lett., 2006, 47, 3773. CrossRef
109. J. A. Marco, M. Carda, S. Rodriguez, E. Castillo, and M. N. Kneeteman, Tetrahedron, 2003, 59, 4085. CrossRef
110. S. Bouz and J. Cossy, Tetrahedron Lett., 2003, 44, 4471. CrossRef
111. S. Raghavan and V. Krishnaiah, Tetrahedron Lett., 2006, 47, 7611. CrossRef
112. G. S. C. Srikanth, U. M. Krishna, G. K. Trivedi, and J. F. Cannon, Tetrahedron, 2006, 62, 11165. CrossRef
113. E. Falomir, J. Murga, M. Carda, and J. A. Macro, Tetrahedron Lett., 2003, 44, 539. CrossRef
114. S. Chandrasekhar, C. Narsihmulu, S. S. Sultana, and M. S. Reddy, Tetrahedron Lett., 2004, 45, 9299. CrossRef
115. P. Kumar, P. Gupta, and S. V. Naidu, Chem. Eur. J., 2006, 12, 1397. CrossRef
116. J. Pospíśil and I. E. Markό, Tetraheron Lett., 2006, 47, 5933. CrossRef
117. R. Grigg, A. Hodgson, J. Morris, and V. Sridharan, Tetrahedron Lett., 2003, 44, 1023. CrossRef
118. T. J. Donohoe, A. J. Orr, and M. Bingham, Angew. Chem. Int. Ed., 2006, 45, 2664. CrossRef
119. T. J. Donohoe, A. J. Orr, K. Gosby, and M. Bingham, Eur. J. Org. Chem., 2005, 1969. CrossRef
120. N. Dieltiens, C. V. Stevens, D. D. Vos, B. Allaert, R. Drozdzak, and F. Verpoort, Tetrahedron Lett., 2004, 45, 8995. CrossRef
121. Q. Yang, W.-J. Xiao, and Z. Yu, Org. Lett., 2005, 7, 871. CrossRef
122. K. Moonen, N. Dieltiens, and C. V. Stevens, J. Org. Chem., 2006, 71, 4006. CrossRef
123. A. S. Davis, N. J. Gates, K. B. Lindsay, M. Tang, and S. G. Pyne, Synlett, 2004, 49. CrossRef
124. D. Balan and H. Adolfsson, Tetrahedron Lett., 2004, 45, 3089. CrossRef
125. C. Bressy, C. Menant, and O. Piva, Synlett, 2005, 577. CrossRef
126. M.-Y. Chang, R.-T. Hsu, T.-W. Tseng, P.-P. Sun, and N.-C. Chang, Tetrahedron, 2004, 60, 5545. CrossRef
127. S. Kim, T. Lee, E. Lee, J. Lee, G.-J. Fan, S. K. Lee, and D. Kim, J. Org. Chem., 2004, 69, 3144. CrossRef
128. N. S. Karanjule, S. D. Markad, and D. D. Dhavale, J. Org. Chem., 2006, 71, 6273. CrossRef
129. T. Kitamura, Y. Sato, and M. Mori, Tetrahedron, 2004, 60, 9649. CrossRef
130. M. Murakami, S. Kadowaki, and T. Matsuda, Org. Lett., 2005, 7, 3953. CrossRef
131. C. Theeraladanon, M. Arisawa, A. Nishida, and M. Nakagawa, Tetrahedron, 2004, 60, 3017. CrossRef
132. M. Arisawa, Y. Terada, K. Takahashi, M. Nakagawa, and A. Nishida, J. Org. Chem., 2006, 71, 4255. CrossRef
133. S. Kotha and K. Singh, Tetrahedron Lett., 2004, 45, 9607. CrossRef
134. J. Perron, B. Joseph, and J.-Y. Mérour, Tetrahedron, 2004, 60, 10099. CrossRef
135. A. Núńez, A. M. Cuadro, J. Alvarez-Builla, and J. J. Vaquero, Org. Lett., 2004, 6, 4125. CrossRef
136. J. Barluenga, C. Mateos, F. Aznar, and C. Valdés, J. Org. Chem., 2004, 69, 7114. CrossRef
137. I. Y. C. Lee, J. Y. Hong, M. H. Jung, and H. W. Lee, Tetrahedron Lett., 2004, 45, 285. CrossRef
138. N. N. Mateeva, L. L. Winfield, and K. K. Redda, Curr. Med. Chem., 2005, 12, 551.
139. X.-F. Zhu, J. Lan, and O. Kwon, J. Am. Chem. Soc., 2003, 125, 4716. CrossRef
140. P. V. Ramachandran, T. E. Burghardt, and L. Bland-Berry, J. Org. Chem., 2005, 70, 7911. CrossRef
141. G. Guanti and R. Riva, Tetrahedron Lett., 2003, 44, 357. CrossRef
142. L. Banafi, G. Guanti, M. Paravidino, and R. Riva, Org. Biomol. Chem., 2005, 3, 1729. CrossRef
143. A. M. Chippindale, S. G. Davies, K. Iwamoto, R. M. Parkin, C. A. P. Smethurst, A. D. Smith, and H. Rodriguez-Solla, Tetrahedron, 2003, 59, 3253. CrossRef
144. Y. Chen, H. V. R. Dias, and C. J. Lovely, Tetrahedron Lett., 2003, 44, 1379. CrossRef
145. A. M. C. H. van den Nieuwendijk, A. B. T. Ghisaidoobe, H. S. Overkleeft, J. Brussee, and A. van der Gen, Tetrahedron, 2004, 60, 10385. CrossRef
146. M. E. Krafft, E.-H. Song, and R. J. Davoile, Tetrahedron Lett., 2005, 46, 6359. CrossRef
147. M.-L. Bennasar, T. Roca, M. Monerris, and D. Garcia-Diaz, Tetrahedron Lett., 2005, 46, 4035. CrossRef
148. J. P. Michael, Nat. Prod. Rep., 2004, 21, 625. CrossRef
149. J. G. Sośnicki, Tetrahedron Lett., 2006, 47, 6809. CrossRef
150. V. Gracias, A. F. Gasiecki, J. D. Moore, I. Akritopoulou-Zanze, and S. W. Djuric, Tetrahedron Lett., 2006, 47, 8977. CrossRef
151. V. D. Matteis, F. L. van Delft, H. Jakobi, S. Lindell, J. Tiebes, and F. P. J. T. Rutjes, J. Org. Chem., 2006, 71, 7527. CrossRef
152. M. Nath, R. Mukhopadhyay, and A. Bhattacharyya, Org. Lett., 2006, 8, 317. CrossRef
153. M. Arisawa, A. Nishida, and M. Nakagawa, J. Organomet. Chem., 2006, 691, 5109. CrossRef
154. M.-Y. Chang, Y.-H. Kung, and S.-T. Chen, Tetrahedron, 2006, 62, 10843. CrossRef
155. W. Chao, Y. R. Mahajan, and S. M. Weinreb, Tetrahedron Lett., 2006, 47, 3815. CrossRef
156. S. P. Chavan, P. Sharma, R. Sivappa, and U. R. Kalkote, Tetrahdron Lett., 2006, 47, 9301. CrossRef
157. N. Desroy, F. Robert-Peillard, J. Toueg, C. Hénaut, R. Duboc, M.-N. Rager, M. Savignac, and J.-P. Genêt, Synthesis, 2004, 2665. CrossRef
158. M. Rosillo, G. Domínguez, L. Casarrubios, U. Amador, and J. Pérez-Castells, J. Org. Chem., 2004, 69, 2084. CrossRef
159. A. Hercouet, C. Baudet, and B. Carboni, Tetrahedron Lett., 2004, 45, 8749. CrossRef
160. M. A. Ciufolini and N. Xi, Chem. Soc. Rev., 1998, 27, 437. CrossRef
161. J. Tae and D.-W. Hahn, Tetrahedron Lett., 2004, 45, 3757. CrossRef
162. M. Mori, H. Wakamatsu, N. Saito, Y. Sato, R. Narita, Y. Sato, and R. Fujita, Tetrahedron, 2006, 62, 3872. CrossRef
163. A. Núńez, A. M. Cuadro, J. Alvarez-Builla, and J. J. Vaquero, Chem. Commun., 2006, 2690. CrossRef
164. G. Magueur, J. Legros, F. Meyer, M. Ourévitch, B. Crousse, and D. Bonnet-Delpon, Eur. J. Org. Chem., 2005, 1258. CrossRef
165. P. Cieplak, P. A. Kollmann, and J. P. Radomski, Molecular Design of Fluorine-Containing Peptide Mimetics, In Biomedicinal Frontiers of Flourine Chemistry, ACS Symposium Series, American Chemical Society, Washington, 1996, Vol. 639, pp. 143-156.
166. S. Fustero, M. Sánchez-Rosellό, D. Jiménez, J. F. Sanz-Cervera, C. del Pozo, and J. L. Aceña, J. Org. Chem., 2006, 71, 2706. CrossRef
167. A. K. Chaterjee, J. P. Morgan, M. Scholl, and R. H. Grubbs, J. Am. Chem. Soc., 2000, 122, 3783. CrossRef
168. L. Manzoni, M. Colombo, and C. Scolastico, Tetrahedon Lett., 2004, 45, 2623. CrossRef
169. S. S. Kinderman, M. M. T. Wekking, J. H. van Maarseveen, H. E. Schoemaker, H. Hiemstra, and F. P. J. T. Rutjes, J. Org. Chem., 2005, 70, 5519. CrossRef
170. M. A. Wijdeven, P. N. M. Botman, R. Witjmans, H. E. Schoemaker, F. P. J. T. Rutjes, and R. H. Blaauw, Org. Lett., 2005, 7, 4005. CrossRef
171. A. B. Smith III and D.-S. Kim, Org. Lett., 2005, 7, 3247. CrossRef
172. K. Takahashi, H. Nakano, and R. Fujita, Tetrahedron Lett., 2005, 46, 8927. CrossRef
173. A. Fürstner, L. Ackermann, B. Gabor, R. Goddard, C. W. Lehmann, R. Mynott, F. Stelzer, and O. R. Thiel, Chem. Eur. J., 2001, 7, 3236. CrossRef
174. A. Deiters, M. Pattersson, and S. F. Martin, J. Org. Chem., 2006, 71, 6547. CrossRef
175. A. Alijarilla, M. C. Murcia, and J. Plumet, Synlett, 2006, 831. CrossRef
176. V. Böhrsch and S. Blechert, Chem. Commun., 2006, 1968. CrossRef
177. V. Böhrsch, J. Neidhöfer, and S. Blechert, Angew. Chem. Int. Ed., 2006, 45, 1302. CrossRef
178. Z. Liu and J. D. Rainier, Org. Lett., 2006, 8, 459. CrossRef
179. A. Rückert, P. H. Deshmukh, and S. Blechert, Tetrahedron Lett., 2006, 47, 7977. CrossRef
180. J. D. Winkler, S. M. Asselin, S. Shepard, and J. Yuan, Org. Lett., 2004, 6, 3821. CrossRef
181. T. Kitamura and M. Mori, Org. Lett., 2001, 3, 1161. CrossRef
182. M. Mori, Y. Kuzuba, T. Kitamura, and Y. Sato, Org. Lett., 2002, 4, 3855. CrossRef
183. M. Mori, H. Wakamatsu, K. Tonogaki, R. Fujita, T. Kitamura, and Y. Sato, J. Org. Chem., 2005, 70, 1066. CrossRef
184. M.-A. Virolleaud and O. Piva, Synlett, 2004, 2087. CrossRef
185. M.-A. Virolleaud, C. Bressy, and O. Piva, Tetrahedron Lett., 2003, 44, 8081. CrossRef
186. M. Tsubuki, K. Kanai, H. Magase, and T. Honda, Tetrahedron, 1999, 55, 2493. CrossRef
187. K. P. Kaliappan and R. S. Nandurdikar, Chem. Commun., 2004, 2506. CrossRef
188. B. Schmidt and Hermanns, J. Curr. Org. Chem., 2006, 1363. CrossRef
189. D. J. Wallace, Tetrahedron Lett., 2003, 44, 2145. CrossRef
190. T. Honda, M. Ushiwata, and H. Mizutani, Tetrahedron Lett., 2006, 47, 6251. CrossRef
191. R. A. J. Wybrow, A. S. Edwards, N. G. Stevenson, H. Adams, C. Johnstone, and J. P. A. Harrity, Tetrahedron, 2004, 60, 8869. CrossRef
192. T. Honda, H. Namiki, K. Kaneda, and H. Mizutani, Org. Lett., 2004, 6, 87. CrossRef
193. J. R. Liddel, Nat. Prod. Rep., 1997, 14, 653. CrossRef
194. S. Ma, B. Ni, and Z. Liang, J. Org. Chem., 2004, 69, 6305. CrossRef
195. H. Fukumoto, K. Takahashi, J. Ishihara, and S. Hatakeyama, Angew. Chem. Int. Ed., 2006, 45, 2731. CrossRef
196. K. Shimizu, M. Takimoto, and M. Mori, Org. Lett., 2003, 5, 2323. CrossRef
197. H. Fukumoto, T. Esumi, J. Ishihara, and S. Hatakeyama, Tetrahedron Lett., 2003, 44, 8047. CrossRef
198. W. Chao, M. L. Meketa, and S. M. Weinreb, Synthesis, 2004, 2058. CrossRef
199. J. Louie, C. W. Bielawski, and R. H. Grubbs, J. Am. Chem. Soc., 2001, 123, 11312. CrossRef
200. A. E. Sutton, B. A. Seigal, D. F. Finnegan, and M. L. Snapper, J. Am. Chem. Soc., 2002, 124, 13390. CrossRef
201. B. Schmidt, Eur. J. Org. Chem., 2003, 816. CrossRef
202. S. Beligny, S. Eibauer, S. Maechling, and S. Blechert, Angew. Chem. Int. Ed., 2006, 45, 1900. CrossRef
203. D. Gagnon, S. Lauzon, C. Godbout, and C. Spino, Org. Lett., 2005, 7, 4769. CrossRef
204. J. M. Kim, K. Y. Lee, S. Lee, and J. N. Kim, Tetrahedron Lett., 2004, 45, 2805. CrossRef
205. W. A. L. van Otterlo, G. L. Morgans, S. D. Khanye, B. A. A. Aderibigbe, J. P. Michael, and D. G. Billing, Tetrahedron Lett., 2004, 45, 9171. CrossRef
206. Y.-K. Yang, J.-H. Choi, and J. Tae, J. Org Chem., 2005, 70, 6995. CrossRef
207. C. D. Edlin, J. Faulkner, D. Fengas, C. K. Knight, J. Parker, I. Preece, P. Quayle, and S. N. Richards, Synlett, 2005, 572. CrossRef
208. S. V. Maifeld, M. N. Tran, and D. Lee, Tetrahedron Lett., 2005, 46, 105. CrossRef
209. D.-W. Hahn, D.-M. Byun, and J. Tae, Eur. J. Org. Chem., 2005, 63. CrossRef
210. C. D. Edlin, J. Faulkner, and P. Quayle, Tetrahedron Lett., 2006, 47, 1145. CrossRef
211. J. Faulkner, C. D. Edlin, D. Fengas, I. Preece, P. Quayle, and S. N. Richards, Tetrahedron Lett., 2005, 46, 2381. CrossRef
212. B. Schmidt and M. Pohler, J. Organomet. Chem., 2005, 690, 5552. CrossRef
213. B. A. Seigal, C. Fajardo, and M. L. Snapper, J. Am. Chem. Soc., 2005, 127, 16329. CrossRef