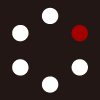
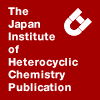
HETEROCYCLES
An International Journal for Reviews and Communications in Heterocyclic ChemistryWeb Edition ISSN: 1881-0942
Published online by The Japan Institute of Heterocyclic Chemistry
e-Journal
Full Text HTML
Received, 24th September, 2008, Accepted, 21st November, 2008, Published online, 28th November, 2008.
DOI: 10.3987/REV-08-SR(D)8
■ Enantioselective Radical Cyclization for the Synthesis of Cyclic Compounds
Eito Yoshioka, Shigeru Kohtani, and Hideto Miyabe*
Graduate School of Pharmaceutical Sciences, Kyoto University, 46-29 Yoshida-shimoadachi-machi, Sakyo-ku, Kyoto 606-8501, Japan
Abstract
This review highlights the enantioselective radical cyclization reactions catalyzed by chiral Lewis acid as well as organocatalyst. The results of the radical cyclization controlled by chiral Al, Ti, Mg, Yb and Zn reagents, the oxidative cyclization using chiral amine, the chiral complexing agent-catalyzed reductive cyclization, transfer of chirality in radical cyclization, and so on are summarized.1. INTRODUCTION
Free radical-mediated reactions have been developed as a powerful method for constructing the carbon-carbon bond. Particularly, the control of stereoselectivity in radical reactions has been of great importance to organic synthesis.1 Hence, new concepts and methods for controlling stereoselectivity of radical reactions are emerging continuously. In the last ten years, the chiral Lewis acid-catalyzed radical reaction has become a field of central importance for asymmetric synthesis. Significant progress has been made in enantioselective radical addition reaction, allylation, and H-atom transfer reactions.2-5 However, studies on enantioselective radical reactions have concentrated on intermolecular reactions, and stereocontrol in intramolecular reactions still remains a major challenge. This review highlights the recent remarkable success in enantioselective radical cyclization reactions catalyzed by chiral Lewis acid as well as organocatalyst or chiral complexing agent.
2. CHIRAL LEWIS ACID-CATALYZED CYCLIZATION
In recent years, studies on enantioselective intermolecular radical addition reactions and radical allylation reactions have been achieved remarkable success by using chiral Lewis acid.2-5 In contrast, only a handful of reports describes the chiral Lewis acid-mediated enantioselective radical cyclizations, which can be classified into three types by the nature of coordination with a chiral Lewis acid (Type I, Type II, and Type III, Figure 1).
Early work on chiral Lewis acid-mediated enantioselective radical type I cyclization was reported by Nishida’s group (Scheme 1).6 The cyclization of α,β-unsaturated ester 1a at -78 °C in the presence of 4 equiv of chiral aluminum Lewis acid 2 gave the cyclic compound 3a in 72% yield and with 36% ee, although the use of 1 equiv of 2 gave the low selectivity. The 6-exo cyclization of ester 1b was inefficient at -78 °C and the reduced product 4 was obtained as a major product. The cyclic product 3b was obtained in 63% yield with 48% ee by performing the reaction at 0 °C. These reactions would proceed via s-trans conformation A. In contrast to esters 1a and 1b giving (R)-products 3a and 3b, the amide 1c gave (S)-product 3c as a major isomer via s-cis conformation C.
Enantioselective cyclization of α-bromo-N-allylamides was studied by Ishii’s group (Scheme 2).7 Among several substrates evaluated, sulfonamide 5 with p-toluenesulfonyl substituent gave good enantioselectivity. When the reaction of 5 was carried out in CH2Cl2 at -78 °C with Bu3SnH (2 equiv) and Lewis acid (1 equiv) derived from Ti(Oi-Pr)4 (1 equiv) and chiral ligand 6 (1 equiv), the product 7 was obtained in 53% yield with 77% ee.
In 2001, a high degree of stereocontrol was achieved in type II cyclizations by Yang and coworkers (Scheme 2).8 The α-radical, generated from β-keto ester, was introduced as a coordination site for the asymmetric cyclization. Atom-transfer cyclization of unsaturated β-keto esters 8a and 8b proceeded smoothly to give the cyclized products 10a and 10b, respectively. Good enantioselectivities were observed when chiral ligand 9 and Mg(ClO4)2 were employed (Table 1). As a solvent effect, toluene generally gave higher enantioselectivities than CH2Cl2 (entries 1 and 2). The addition of activated molecular sieves not only led to an increase in yields and enantioselectivities, but also made it possible for the use of catalytic amounts of the chiral Lewis acid (entries 3, 4 and 6). High enantioselectivity was attained even when using 0.3 equiv of the chiral Lewis acid catalyst (entry 4).
Enantioselective tandem or cascade radical cyclizations continue to attract much interest, since highly functionalized compounds with multiple stereocenter are available. The type II cyclization was applied to cascade cyclization. Yang reported the enantioselective atom-transfer cascade cyclization of α-bromo β-keto esters (Scheme 4).9 The 6-endo/6-exo cyclization of 11 was performed with ligand 9 and Mg(ClO4)2. Good enantioselectivity of the fused ring product 12 was observed when reaction was carried out in toluene at -20 °C, but the yield of the product 12 was low. The asymmetric 6-endo/5-exo cyclization of 13 was evaluated by using Yb(OTf)3. The combination of Pybox ligand 14 and Yb(OTf)3 in CH2Cl2 gave the best result.
Catalytic enantioselective group-transfer radical cyclization was also studied by Yang (Scheme 5),10 who investigated the PhSe-group-transfer cyclization of α-phenylseleno β-keto ester 16. Chiral Lewis acid, derived from Mg(ClO4)2 and box ligand 9, was the suitable catalyst for this cyclization. The addition of activated molecular sieves accelerated the reaction. The 6-exo product 17 was obtained with 89% ee, when β-keto ester (Z)-16 was employed as a substrate (Table 2, entry 1). When a catalytic amount (0.3 equiv) of chiral Lewis acid was used, the enantioselectivity slightly decreased to 84% ee (entry 2). Additionally, β-keto ester (E)-16 gave the same product 17 in comparable yields and enantioselectivities (entries 4 and 5).
Yang reported that PhSe-group-transfer reaction was ideal in terms of both reaction efficiency and enantioselectivity for the cascade cyclization (Scheme 6).10 Substrate 18 underwent the 6-endo/6-exo cyclization to give the fused ring product 19 with 97% ee albeit in low yield. In contrast to the low yield in the corresponding Br-transfer cascade cyclization,9 the fused ring product 21 was obtained from substrate 20 in 70% yield with 73% ee. Good chemical yield of 21 was attained even when 0.3 equiv of the chiral Lewis acid catalyst was used. They assume that the lower transfer rate of the PhSe group to the intermediate alkyl radical and its bulk cause the PhSe group abstraction to be more stereoselective than that of Br-transfer cascade cyclization.
Most radical reactions proceed through early transition states; thus, the geometry of substrates play an important role for asymmetric reaction. The control of the rotamer population would be crucial for achieving high enantioselectivity in radical cyclization reactions.11 We consider that the predominant formation of a single reactive rotamer must be achieved by the type III approach (Figure 1), which contains a coordination tether (X) between two acceptors.12 To develop a highly efficient and stereoselective method, we have studied new approaches to control stereoselectivity of cascade radical addition-cyclization reaction by taking advantage of hydroxamate ester 23 (Figure 2).13
There are no reports on enantioselective cascade reactions involving both inter- and intramolecular carbon-carbon bond forming processes. Therefore, the isopropyl radical addition-cyclization-trapping reaction of hydroxamate esters 24a-c was studied (Scheme 7). In this reaction, the hydroxamate ester moiety could control the rotamer population of substrates through a stable five-membered chelation.
While practically no reaction occurred in the absence of Lewis acid additive, the use of chiral Lewis acid led to an enhancement in chemical yield even at -78 °C. In the presence of stoichiometric amounts of the chiral Lewis acid prepared from Zn(OTf)2 and box ligand 25, cascade reaction of 24a proceeded smoothly to give the 5-exo cyclization product 26a in 81% yield with good enantioselectivity and high cis diastereoselectivity. We were also interested in probing the effect of the fluxional substituent (R) of 24 on the stereochemistry. 14 Reaction of 24b, which has a small methoxy group, led to high enantioselectivity. Interestingly, the size of the fluxional substituent (R) had an impact on enantioselectivity, with larger groups leading to lower ee values. The use of substrate 24c with a diphenylmethyl group gave the racemic product 26c, probably as a result of dissonance between the chiral Lewis acid and bulky substituent. Cyclization of 24a-c leading to the major cis diastereomer occurs via the conformer D, in which two olefin units adopt a cis arrangement probably due to the effect of orbital symmetry reported by Beckwith and Houk.15
Outstanding level of enantioselectivity was obtained in the cascade reaction of acrylated substrate 27. In marked contrast to methacrylated substrates 24a-c, the trans diastereoselectivity was observed. The trans-selectivity in reaction of 27 was regarded as the result of steric repulsion between two olefin units and the reaction proceeded via the stable conformer E.
Next, the cascade reaction of chiral substrate (R)-29 was investigated (Scheme 8). The reaction of (R)-29 (81% ee) gave a 63% yield of (S)-cis-30 with 99% ee, accompanied by a small amount of trans-30 with low enantiomeric excess. The enhanced enantioselectivity of cis-30 can be explained by kinetic resolution of an intermediate chiral radical. The major cyclization proceeded via the favorable conformer F minimizing the allylic 1,3-strain effect.
Chiral Lewis acid promoted the reaction of substrates 31a and 31b having carbon-carbon triple bond to afford the cyclized products 32a and 32b in high yields and with good enantioselectivities (Scheme 9). The high Z/E selectivity of products indicates that the iodine atom-transfer from i-PrI to reactive intermediate vinyl radical was effective and directed the Z selectivity.
Nothing has been known about the enantioselective radical cyclization of imines;16 thus, we next explored the cascade reaction of oxime ether 33 (Scheme 10).17 Interestingly, the amount of triethylborane and the reaction time had an impact on enantioselectivity (Table 3). Increasing the amount of triethylborane from 2.5 equiv to 20 equiv improved the enantioselectivity (compare entry 1 with entry 2). Additionally, a prolonged reaction led to lower selectivity (entry 3). Improvement of enantioselectivity was also observed by changing the reaction time from 3 h to 1 h (entry 4). Result from these studies shows that the slow trapping reaction of an aminyl radical H with triethylborane would allow the reversibility between intermediate G and aminyl radical H, leading to erosion of enantioselectivity.
3 CYCLIZATION USING ORGANOCATALYST OR CHIRAL COMPLEXING AGENT
MacMillan has studied the oxidative radical reactions using chiral organocatalyst.18 Enantioselective cyclization of unsaturated aldehyde 35 was investigated in the presence of ceric ammonium nitrate (CAN) as the stoichiometric oxidant (Scheme 11). In this reaction, chiral amine catalyst 36 activates aldehyde 35 by the formation of enamine, which gives a transient radical cation intermediate by single-electron oxidation. In the presence of LiCl, aldehyde 35 underwent the 5-exo cyclization to give the halogenated product 37 with 95% ee with good diastereoselectivity.
The enantioselective radical cyclization was also achieved by Bach’s group, who relied on an unique hydrogen-bonding chiral complexing agent (Scheme 12).19 Chiral complexing agent 39 features a hydrogen bond donor (NH) site and a hydrogen bond acceptor (C=O) site. The reductive radical cyclization of piperidin-2-one 38 was investigated in the presence of Bu3SnH and Et3B. When chiral complexing agent 39 (2.5 equiv) was employed in toluene at -78 °C, the cyclic product 40 was obtained in 81% yield with 84% ee. In nonpolar solvents, chiral complexing agent 39 binds lactam moiety in 38 and 40, facilitating a differentiation of enantiotopic faces. However, this reaction can not be categorized to direct stereocontrol in radical cyclizations, because the stereodetermining step is hydrogen transfer from Bu3SnH to intermediate radical.
Chiral complexing agent-mediated direct stereocontrol in radical cyclizations was achieved by using quinolones as substrates (Scheme 13).20 The reaction of the unsubstituted iodide 41 gave 6-endo cyclization product 42. When chiral complexing agent 39 (2.5 equiv) was employed in PhCF3 at 0 °C, the cyclic product 42 was obtained with 99% ee. In addition, the cyclized product 42 was obtained with 55% ee even in the presence of only 0.1 equiv of chiral complexing agent. The cyclization of substituted iodide 43 occurred with regioselectivity different from that of 41. When chiral complexing agent 39 (2.5 equiv) was employed in PhCF3 at 0 °C, the 5-exo cyclization product 44 was formed predominantly with excellent enantioselectivity.
Bach studied the catalytic enantioselective radical cyclization driven by photoinduced electron transfer (PET).21 The electron accepting chiral catalyst 46 was explored for enantioselective radical cyclization of quinolone 45 (Scheme 14). The chiral catalyst 46 serves not only as a PET agent, but also as stereocontrolling device. By employing catalyst 46, the desired reaction proceeded to give the spirocyclic product 47 in good yield. The enantiomeric excess reached 70% for a catalyst loading of 30 mol%. This performance suggests that photochemical reactions may find use in general asymmetric synthesis.22
As unique cyclization procedure, transfer of chirality was investigated by Curran’s group (Scheme 15).23 Even though the chiral axis is destroyed in cyclization process, the products faithfully remember the configuration of the precursors. The axial chirality of 48 was transferred into a new stereocenter of 49 with retention of chirality as a result of the almost complete absence of racemization of radical intermediates.
4 CONCLUSION AND OUTLOOK
Synthetic strategies involving radical cyclizations would be desirable tools for preparing organic compounds. Enantioselective radical cyclizations continue to attract much interest, since highly functionalized compounds with multiple stereocenter can be provided. The new concept and approach for controlling stereochemistry of radical reactions offer opportunities for further exploration with intriguing possibilities in enantioselective radical cyclization.
ACKNOWLEDGEMENTS
This work was supported in part by a Grant-in-Aid for Young Scientists (B) (to E.Y.) and for Scientific Research (C) (to H.M.) from the Ministry of Education, Culture, Sports, Science and Technology of Japan, and Hyogo Science and Technology Association (to H.M.).
References
1. D. P. Curran, N. A. Porter, and B. Giese, ‘Stereochemistry of Radical Reactions: Concepts, Guidelines, and Synthetic Applications’, VCH: Weinheim, 1996; ‘Radicals in Organic Synthesis’ Vols. 1 and 2, ed. by P. Renaud and M. P. Sibi, Wiley-VCH, Weinheim, 2001.
2. For selected examples of enantioselective radical addition reactions and allylations, see: M. P. Sibi, G. Petrovic, and J. Zimmerman, J. Am. Chem. Soc., 2005, 127, 2390; CrossRef M. P. Sibi, J. Zimmerman, and T. Rheault, Angew. Chem. Int. Ed., 2003, 42, 4521; CrossRef Y. Watanabe, N. Mase, R. Furue, and T. Toru, Tetrahedron Lett., 2001, 42, 2981; CrossRef U. Iserloh, D. P. Curran, and S. Kanemasa, Tetrahedron: Asymmetry, 1999, 10, 2417; CrossRef U. Iserloh, D. P. Curran, and S. Kanemasa, J. Am. Chem. Soc., 1997, 119, 11713; CrossRef M. P. Sibi, J. Ji, J. H. Wu, S. Gürtler, and N. A. Porter, J. Am. Chem. Soc., 1996, 118, 9200; CrossRef J. H. Wu, R. Radinov, and N. A. Porter, J. Am. Chem. Soc., 1995, 117, 11029. CrossRef
3. For selected examples of enantioselective H-atom transfer reactions, see: M. P. Sibi and K. Patil, Angew. Chem. Int. Ed., 2004, 43, 1235; CrossRef M. P. Sibi, Y. Asano, and J. B. Sausker, Angew. Chem. Int. Ed., 2001, 40, 1293; CrossRef M. Murakata, H. Tsutsui, N. Takeuchi, and O. Hoshino, Tetrahedron, 1999, 55, 10295. CrossRef
4. For selective examples of enantioselective reductions using chiral H-atom transfer reagents, see: Y. Cai, B. P. Roberts, and D. A. Tocher, J. Chem. Soc., Perkin Trans. 1, 2002, 1376; CrossRef D. Dakternieks and C. H. Schiesser, Aust. J. Chem., 2001, 54, 89; CrossRef M. Blumenstein, K. Schwarzkopf, and J. O. Metzger, Angew. Chem. Int. Ed., 1997, 36, 235; CrossRef D. Nanni and D. P. Curran, Tetrahedron: Asymmetry, 1996, 7, 2417. CrossRef
5. For general information details for enantioselective radical reactions, see: P. Renaud and M. Gerster, Angew. Chem. Int. Ed., 1998, 37, 2562; CrossRef M. P. Sibi and N. A. Porter, Acc. Chem. Res., 1999, 32, 163; CrossRef G. Bar and A. F. Parsons, Chem. Soc. Rev., 2003, 32, 251; CrossRef M. P. Sibi, S. Manyem, and J. Zimmerman, Chem. Rev., 2003, 103, 3263. CrossRef
6. M. Nishida, H. Hayashi, A. Nishida, and N. Kawahara, Chem. Commun., 1996, 579. CrossRef
7. K. Hiroi and M. Ishii, Tetrahedron Lett., 2000, 41, 7071. CrossRef
8. D. Yang, S. Gu, Y.-L. Yan, N.-Y. Zhu, and K.-K. Cheung, J. Am. Chem. Soc., 2001, 123, 8612; CrossRef D. Yang, Y.-L. Yan, K.-L. Law, and N.-Y. Zhu, Tetrahedron, 2003, 59, 10465. CrossRef
9. D. Yang, S. Gu, Y.-L. Yan, H.-W. Zhao, and N.-Y. Zhu, Angew. Chem. Int. Ed., 2002, 41, 3014. CrossRef
10. D. Yang, B.-F. Zheng, Q. Gao, S. Gu, and N.-Y. Zhu, Angew. Chem. Int. Ed., 2006, 45, 255. CrossRef
11. M. P. Sibi and J. Ji, J. Am. Chem. Soc., 1996, 118, 3063. CrossRef
12. H. Miyabe, R. Asada, A. Toyoda, and Y. Takemoto, Angew. Chem. Int. Ed., 2006, 45, 5863; CrossRef H. Miyabe and Y. Takemoto, Chem. Eur. J., 2007, 13, 7280. CrossRef
13. O. Corminboeuf and P. Renaud, Org. Lett., 2002, 4, 1731; CrossRef O. Corminboeuf and P. Renaud, Org. Lett., 2002, 4, 1735. CrossRef
14. O. Corminboeuf, L. Quaranta, P. Renaud, M. Liu, C. P. Jasperse, and M. P. Sibi, Chem. Eur. J., 2003, 9, 28. CrossRef
15. A. L. J. Beckwith, Tetrahedron, 1981, 37, 3073; CrossRef D. C. Spellmeyer and K. N. Houk, J. Org. Chem., 1987, 52, 959. CrossRef
16. For reviews, see: T. Naito, Heterocycles, 1999, 50, 505; CrossRef A. G. Fallis and I. M. Brinza, Tetrahedron, 1997, 53, 17543; CrossRef G. K. Friestad, Tetrahedron, 2001, 57, 5461; CrossRef H. Miyabe, M. Ueda, and T. Naito, Synlett, 2004, 1140. CrossRef
17. H. Miyabe, A. Toyoda, and Y. Takemoto, Synlett, 2007, 1885. CrossRef
18. T. D. Beeson, A. Mastracchio, J.-B. Hong, K. Ashton, and D. W. C. MacMillan, Science, 2007, 316, 582. CrossRef
19. T. Aechtner, M. Dressel, and T. Bach, Angew. Chem. Int. Ed., 2004, 43, 5849; CrossRef M. Dressel, T. Aechtner, and T. Bach, Synthesis, 2006, 2206. CrossRef
20. M. Dressel and T. Bach, Org. Lett., 2006, 8, 3145. CrossRef
21. A. Bauer, F. Westkämper, S. Grimme, and T. Bach, Nature, 2005, 436, 1139. CrossRef
22. P. Wessig, Angew. Chem. Int. Ed., 2006, 45, 2168. CrossRef
23. D. P. Curran, W. Liu, and C. H.-T. Chen, J. Am. Chem. Soc., 1999, 121, 11012. CrossRef