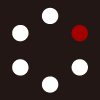
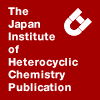
HETEROCYCLES
An International Journal for Reviews and Communications in Heterocyclic ChemistryWeb Edition ISSN: 1881-0942
Published online by The Japan Institute of Heterocyclic Chemistry
e-Journal
Full Text HTML
Received, 12th March, 2008, Accepted, 15th April, 2008, Published online, 17th April, 2008.
DOI: 10.3987/COM-08-S(F)6
■ An Oxidative Dearomatization Cyclization Model for Cortistatin A
Mingji Dai and Samuel J. Danishefsky*
Department of Chemistry, Columbia University, 3000 Broadway, New York, NY 10027, U.S.A.
Abstract
The feasibility of a hypervalent iodine-mediated oxidative dearomatization/cyclization to produce the oxabicyclo[3.2.1]octene of cortistatin A has been demonstrated through a model study. An interesting tandem cyclization via a Ritter intermediate has been observed when a slightly different substrate was used.The cortistatins, a novel class of steroidal alkaloids, were recently isolated by Kobayashi and coworkers from the marine sponge, Corticium simplex (Figure 1). Cortistatin A (1), in particular, was found to be a potent and selective inhibitor of angiogenesis, exhibiting cytostatic antiproliferative activity against human umbilical vein endothelial cells (HUVECs) at concentrations as low as 100 pM.1 The high selectivity observed for the endothelial (HUVEC) cell line, in comparison with other normal and cancerous cell lines, suggests that cortistatin A (1) is, in fact, a selective angiogenesis inhibitor. Angiogenesis, the process of forming new blood vessels, is essential to a variety of critical functions, from reproduction to wound repair. However, persistent and unregulated angiogenesis has been implicated in a range of maladies, including the progression of solid tumors, diabetic retinopathy, psoriasis, and rheumatoid arthritis.2 Since tumor growth and metastasis are highly dependent on angiogenesis, there is great interest in exploring the therapeutic potential of small molecule anti-angiogenesis agents, such as cortistatin A (1).
Structurally, the cortistatins are a unique family of 9(10-19)-abeo-androstane-type steroidal alkaloids possessing both an oxabicyclo[3.2.1]octene and an isoquinoline unit or its precursor. Intrigued by the therapeutic potential of a selective anti-angiogenic agent and the synthetic challenges presented by the cortistatin structural framework, we have initiated an effort directed toward the total synthesis of cortistatin A (1). Our convergent synthetic plan would be highly amenable to the preparation of a variety of analogs through diverted total synthesis.
As outlined in Scheme 1, a defining transformation of our proposed synthetic route would involve the use of a hypervalent iodine3–mediated oxidative dearomatization to produce the oxabicyclo[3.2.1]octene of cortistatin A (1). Under this plan, electrophilic intermediate (8) would be generated through PIFA-mediated oxidation of phenol 7. Intramolecular interception of 8 would yield the target compound (9). This intermediate could serve as a key launching point, from which we could prepare a range of cortistatin analogs through diverted total synthesis.
In designing our synthetic route, we were well aware of some potential liabilities associated with the proposed key transformation. Thus, although a variety of inter- and intramolecular nucleophiles have been used to trap electrophilic intermediates generated through PIFA activation of phenols,4 tertiary alcohols have rarely been used for this purpose.5 We were further mindful of the potential for exacerbating ring strain as a consequence of the proposed transformation. With these considerations in mind, we commenced our investigations toward cortistatin A with an admittedly early stage model study which would seek to learn something about the feasibility of the key oxidative dearomatization cyclization sequence.
We chose as our model substrates, 17 and 18. The synthesis of these compounds commenced with 7-methoxy-1-tetralone (10).6 Reduction of the latter, followed by acid promoted dehydration, afforded olefin (11) which, without further purification, was treated with bromoform and potassium tert-butoxide in pentane at –10 ºC to give adduct (12) in 83% yield for 3 steps.7 Solvolysis of 12 with 3 equiv of silver perchlorate in 95/5 (v/v) acetone-water gave a 74% yield of the allylic alcohol (13), which was immediately oxidized with MnO2 to afford ring expanded bromoenone (14). Treatment of the latter with methyllithium led to a mixture of debrominated enone (15) and the debrominated 1,2-addition product (16) in 34% and 30% yield respectively. Enone (15) could be transformed to allylic alcohol (16) by further treatment of methyllithium (quantitative yield based on recovering starting material).8 Demethylation in the presence of NaSEt gave the model substrate, phenol (17). Finally, Pd-C catalyzed hydrogenation of 17 afforded the second model compound (18).
With both 17 and 18 in hand, we were prepared to explore the key oxidative dearomatization cyclization. As shown in Scheme 3, we found that, when a solution of 17 in acetonitrile was treated with 1.0 eq. of PIFA in the presence of 2.0 eq. K2CO3, the desired cyclized product (19) was obtained in approximately 30% yield, with 30% of the starting material recycled. In addition to the 1H NMR and 13C NMR, the structure of 19 was further verified by X-ray crystallography. Efforts to optimize the reaction through an increase in the amount of oxidizing agent were unsuccessful, as the use of 2.0 eq. of PIFA provided a lower yield of the desired product (19). Interestingly, when 18 was submitted to the same reaction conditions, none of the desired product (20) was observed. Instead, a 30% yield of 23 was obtained. A proposed mechanism for the formation of 23 is shown in Scheme 3. It appears that the electrophilic intermediate (21) produced through PIFA activation of 18, is attacked by acetonitrile in a Ritter reaction,9 to form 22, which is further trapped by the intramolecularly situated tertiary hydroxyl group, to afford 23.10 The divergent pathways observed in the oxidative cyclization of 17 and 18 may be attributed to conformational constraints imposed by the double bond in 17. A similar observation has in fact been reported by Pelter and co-workers.5a In order to avoid the participation of acetonitrile, other solvents such as toluene, CH2Cl2 and HFIP (hexafluoroisopropanol), were also explored. Unfortunately, each suffered from low solubility of the phenols (17 and 18) or low reaction yield. However, although only modest yields were achieved in our model study, we have demonstrated the viability of the oxidative dearomatization cyclization sequence in generating an element of the core structure of cortistatin A. Conceivably, means can be uncovered to deal with the solubility issues which have compromised our efforts in the model system.
In summary, the feasibility of our oxidative dearomatization cyclization approach to cortistatin A has been demonstrated through a model study. An interesting tandem cyclization via Ritter intermediate has been observed. Efforts are currently underway to apply the oxidative dearomatization cyclization sequence to an efficient synthesis of cortistatin A (1).
ACKNOWLEDGEMENTS
We are grateful to the National Institutes of Health (HL25848) for financial support of this research. We thank Ms. Daniela Buccella for crystal structure analysis. M. D. thanks the Guthikonda family for a graduate fellowship (2007-2008).
Dedication – We dedicate this manuscript to Professor Emeritus Keiichiro Fukumoto for his continuing studies in organic chemistry.
References
1. a) S. Aoki, Y. Watanabe, M. Sanagawa, A. Setiawan, N. Kotoku, and M. Kobayashi, J. Am. Chem. Soc., 2006, 128, 3148; CrossRef b) Y. Watanabe, S. Aoki, D. Tanabe, A. Setiawan, and M. Kobayashi, Tetrahedron, 2007, 63, 4074; CrossRef c) S. Aoki, Y. Watanabe, D. Tanabe, A. Setiawan, M. Arai, and M. Kobayashi, Tetrahedron Lett., 2007, 48, 4485. CrossRef
2. a) J. Folkman, New Engl. J. Med., 1995, 333, 1757; CrossRef b) J. Folkman and Y. Shing, J. Biol. Chem., 1992, 267, 10931.
3. For reviews: a) P. J. Stang and V. V. Zhdankin, Chem. Rev., 1996, 96, 1123; CrossRef b) V. V. Zhdankin and P. J. Stang, Chem. Rev., 2002, 102, 2523. CrossRef
4. a) Y. Tamura, T. Yakura, J.-I. Haruta, and Y. Kita, J. Org. Chem., 1987, 52, 3927; CrossRef b) A. Pelter and S. Elgendy, Tetrahedron Lett., 1988, 29, 677; CrossRef c) A. Pelter and R. S. Ward, Tetrahedron, 2001, 57, 273; CrossRef d) C. Hoarau and T. R. R. Pettus, Org. Lett., 2006, 8, 2843. CrossRef
5. a) A. Pelter, A. Hussain, G. Smith, and R. S. Ward, Tetrahedron, 1997, 53, 3879; CrossRef b) R. Venkateswarlu, C. Kamakshi, S. G. A. Moinuddin, P. V. Subhash, R. S. Ward, A. Pelter, S. J. Coles, M. B. Hursthouse, and M. E. Light, Tetrahedron, 2001, 57, 5625; CrossRef c) S. Quideau, M. Lebon, and A.-M. Lamidey, Org. Lett., 2002, 4, 3975. CrossRef
6. W. E. Bachmann and W. J. Horton, J. Am. Chem. Soc., 1947, 69, 58. CrossRef
7. J. E. McMurry and S. J. Isser, J. Am. Chem. Soc., 1972, 94, 7132. CrossRef
8. M. Dai and S. J. Danishefsky, J. Am. Chem. Soc., 2007, 129, 3498. CrossRef
9. a) I. Drutu, J. T. Njardarson, and J. L. Wood, Org. Lett., 2002, 4, 493; CrossRef b) S. Canesi, D. Bouchu, and M. A. Ciufolini, Org. Lett., 2005, 7, 175. CrossRef
10. L. Castellanos, C. Dupue, J. Rodríguez, and C. Jiménez, Tetrahedron, 2007, 63, 1544. CrossRef