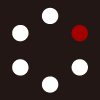
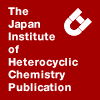
HETEROCYCLES
An International Journal for Reviews and Communications in Heterocyclic ChemistryWeb Edition ISSN: 1881-0942
Published online by The Japan Institute of Heterocyclic Chemistry
e-Journal
Full Text HTML
Received, 4th April, 2008, Accepted, 27th May, 2008, Published online, 2nd June, 2008.
DOI: 10.3987/REV-08-SR(F)1
■ Syntheses of Peptidyl Nucleoside Antibiotics
Hiroyuki Akita*
Department of Pharmaceutical Sciences, Nihon Pharmaceutical University, , Japan
Abstract
Polyoxins and nikkomycins are an important class of peptidyl nucleoside antibiotics isolated from the culture broths of Streptomyces cacaoi var. asoensis and Streptomyces tendae. For the syntheses of these antibiotics, efficient syntheses of 1-(5-amino-5-deoxy-β-D-allofurano-uronosyl)pyrimidines such as thymine polyoxin C, uracil polyoxin C and their congeners as a basic component corresponding to the right half were achieved based on the nucleophic 1,2-addition to methyl 2,3-O-isopropylidene-β-D-ribopentodialdo-1,4-furanoside. Then the syntheses of polyoxamic acid derivatives and their congeners corresponding to the left acid part were carried out based on 1,2-addition of carbon nucleophile to 4-O-protected-2,3-O-isopropylidene-L-threose. Coupling reaction of the activated ester derived from the left half acid part and amine part derived from the right half gave the N,O-protected peptidyl nucleoside congeners which were subjected to deprotection to afford polyoxins B, D, J, L, M, C and nikkomycin B.1. INTRODUCTION
Polyoxins are an important class of peptidyl nucleoside antibiotics isolated from the culture broths of Streptomyces cacaoi var. asoensis, which are potent competitive inhibitors of chitin synthetase of a variety of phytopathogenic fungi or Candida albicans, a medically important human fungal pathogen.1 Nikkomycins, peculiar peptidyl nucleoside antibiotics isolated from the culture broths Streptomyces tendae and Streptomyces cacaoi subsp. Asoensis exhibit fungicide and insecticide activities due to an inhibition of cell wall chitin biosynthesis.2,3 From the point of view of fungal infections, chitin synthetase inhibition seems to be a useful approach for the sake of safer antifungal agents and much effort has been devoted to the total synthesis of these antibiotics. For convenience, established structures of the polyoxins A (1)-I (13) and nikkomycins B (14) and Z (15) are shown in Scheme 1. Among them, the total syntheses of polyoxin J (8) starting from D-glucose4a or myo-inositol4b were reported and were achieved based on the stereoselective addition of 2-lithiofuran to the sugar nitrone.4c,d In this review, we summarize the syntheses of polyoxins B (2), D (3), J (8), L (10), M (11), C (12), and nikkomycin B (14).
2. Syntheses of polyoxins L (10), J (8), B (2) and D (3)
Retrosynthetically, the synthesis of polyoxins L (10), J (8), B (2) and D (3) can be achieved by amide formation between the 1-(5-amino-5-deoxy-β-D-allofuranouronosyl)pyrimidines derivatives {uracil polyoxin C (16), thymine polyoxin C (17), polyoxin C (12) and polyoxin C acid (18)} corresponding to the right-half and the polyoxamic acid (19) congener corresponding to the left-half as shown in Scheme 2.
2.1. Synthesis of right-half {Syntheses of uracil polyoxin C (16) and thymine polyoxin C (17)}5a,b
A variety of chemical syntheses of amino acid nucleosides (16 and 17) have been reported over the years.5c-f One of the most important intermediate for the general synthesis of them appeared to be (R)-α-hydroxy esters (20 or 21). The synthesis of 20 or 21 could be achieved based on the nucleophilic addition of carbon-nucleophile to methyl 2,3-O-isopropylidene-β-D-ribo-pentodialdo -1,4-furanoside (22)
derived from D-ribose. Nucleophilic 1,2-addition of allyl organometallics to 22 has been reported to give a preferential product A, as a result of re-face attack on the unchelated aldehyde (non-chelation) a preferential product A, as a result of re-face attack on the unchelated aldehyde (non-chelation) with a C4-C5 conformation depicted in the structure of 22.6 Taking into account the effect of coexisting metal halides, the β-chelation of the metal ion may give the re-face attack product A as a major component, while the α-chelation of the metal ion would afford the si-face attack product B as a major product (Scheme 3). The synthesis of uracil polyoxin C (16) and thymine polyoxin C (17) were shown in Scheme 4. Treatment of D-ribose with acetone in the presence of conc. HCl and MeOH followed by
Swern oxidation gave the 5-aldehyde (22) (60%). The reaction of 22 with (1-ethoxyvinyl)lithium followed by ozonolysis and subsequent treatment with dimethylsulfide gave a diastereomeric mixture of α-hydroxy esters, which were separated into the major α-hydroxy ester (23) (31% from 22) and the minor one (24) (10%). For the purpose of conversion of 23 into 24, treatment of 23 with trifluoromethanesulfonic anhydride (Tf2O) afforded the triflate (25) (83%), which was treated with benzoic acid in the presence of CsF to provide the α-benzoyloxy ester (26) (86%). Alcoholysis of 26 gave the C-5 inverted isomer (24) (65%) which was consistent with the minor component of α-hydroxy esters. In order to determine the stereochemistry of 24, the α-hydroxy ethyl ester 24 was converted to the reported (5S)-azide methyl ester 27.7 Transesterification of 24 with MeOH into the methyl ester (28) in the presence of Ti(O-iPr)4 was achieved in 84% yield. Triflation of 28 followed by treatment of the triflate (29) (74%) with NaN3 afforded the diastereomerically pure α-azide ester 27 (95%) whose spectral data were identical with those of the reported (5S)-27. Thus, the stereochemistry due to the C-5 position of α-hydroxy ethyl esters 23 and 24 was found to be (S)- and (R)-configurations, respectively. For the total synthesis of the target molecules 16 and 17, conversion of the ethyl ester group into the methyl ester group is not always essential process. The (R)-α-hydroxy ethyl ester (24) was converted to the (S)-α-azide ethyl ester (31) (55% from 24) via the triflate (30) (64%) by the same way as in the case of conversion of 29 to 27. Deisopropylidenation of 31 (Dowex 50W H+, EtOH, reflux) afforded the diol, which was acetylated directly (Ac2O, pyridine) to yield the diacetate (32) (87%). Anomeric acetolysis smoothly gave the triacetate (33) (88%) in which no C-5 epimerization could be detected. Reaction of the key triacetate (33) with 2,4-bis(trimethylsilyloxy)pyrimidine under the conditions reported by Vοrbrüggen {trimethylsilyl trifluoromethanesulfate (TMSOTf), ClCH2CH2Cl, reflux}8 afforded exclusively the β-nucleoside (34) (84%). Hydrogenation of the azide (34) in the presence of 5% Pd-BaSO4 followed by protection of the amino group with CbzCl in the presence of 7% aqueous NaHCO3 gave the (5S)-35 (74%). Alkaline hydrolysis of 35 followed by hydrogenation afforded uracil polyoxin C (16) (72%). Likewisw, reaction of 33 with 5-methyl-2,4-bis(trimethylsilyloxy) pyrimidine under similar conditions afforded exclusively the β-nucleoside (36) (84%). Hydrogenation of the azide (36) in the presence of 20% Pd(OH)2-C afforded the α-amino acid ester which was treated with benzyl chloroformate (CbzCl) in the presence of 7% aqueous NaHCO3 to provide the (5S)-37 (90%). Alkaline hydrolysis of 37 followed by hydrogenation gave thymine polyoxin C (17) (75%). The physical data of the synthetic material (17) were identical with those of authentic material (17).9a,b The synthesis described herein demonstrates the utility of (1-ethoxyvinyl)lithium for the short path synthesis of the α-hydroxy esters (23 and 24) from the aldehyde (22), which contributed to the total syntheses of uracil polyoxin C (16) and thymine polyoxin C (17).
2. 2. Improved synthesis of uracil polyoxin C (16)10
The α-hydroxy esters (5S)-23 and (5R)-24 are obtained from the reaction of 22 and 1-ethoxyvinyl lithium followed by ozonolysis and subsequent reductive treatment in overall yield of 31% and 10%, respectively. Although both (5S)-23 and (5R)-24 were converted to the desired α-azide ester (31), overall yield of 31 from 22 is quite low (13%). We report the improved synthesis of α-azido ester (5S)-27 from 22 and its application to the total synthesis of uracil polyoxin C (16) as shown in Scheme 5. The reaction of 22 with vinylmagnesium bromide was carried out and the reaction mixture was subjected to acetylation to gave a 3.7:1 diastereomeric mixture of acetoxy compounds 38 and 39 in 71% overall yield. Oxidative treatment of this mixture with RuCl4 and NaIO4 followed by consecutive esterification and hydrolysis afforded a mixture of α−hydroxy esters, which were separated to the more polar (5S)-40 (64% overall yield) and the less polar (5R)-28 (22% overall yield). Treatment of 40 with iodine and triphenylphosphine in the presence of imidazole gave the inverted α-iodo ester (41) (92%) which was treated with NaN3 to
afford the α-azido ester (27) (98%). Thus overall yield of the desired 27 from 22 was totally improved to 55% in comparison with the previous case (13%) or the reported procedure (38%) by Barrett et al.7
For the purpose of the improvement of the diastereoselectivity, effect of coexisting metal halide in addition to 22 was examined and the results are shown in Table 1. In comparison with no additive (entry 1), addition of metal ion (entries 2 and 3) decrease the overall yield of products (38 and 39) and additive CeCl3 (entry 2) may enhance the β-chelation effect. In every case, improvement of selectivity was not found. Thus obtained (5S)-27 was subjected to consecutive treatment with Dowex 50W H+ resin in MeOH and Ac2O in pyridine to afford the triacetate 42 (69% overall yield from 40) in which no C-5 epimerization could be detected. Reaction of the triacetate (42) with 2,4-bis(trimethylsilyloxy)- pyrimidine under the conditions reported by Vοrbrüggen (TMSOTf, ClCH2CH2Cl, reflux) gave exclusively the β-nucleoside (43) (89%). Hydrogenation of the azide (43) in the presence of 10% Pd-C afforded the α-amino acid ester which was treated with CbzCl in the presence of 7% aqueous NaHCO3 to provide the (5S)-44 (66%). Alkaline hydrolysis of 44 followed by hydrogenation gave uracil polyoxin C (16) (53%).
2. 3. Synthesis of polyoxin C (12) and polyoxin C acid (18)
By applying the Vorbrüggen procedure,8 the commercially available 5-hydroxymethyl uracil (45) was treated with 1,1,1,3,3,3-hexamethyldisilazane and trimethylsilyl chloride to give the 5-trimethylsiloxymethyl-2,4-bis(trimethylsiloxy)pyrimidine (46) which was reacted with the triacetate (42) in the presence of TMSOTf to afford exclusively the β-nucleoside (47) in 62% overall yield. Hydrogenation of the azide (47) in the presence of 10% Pd-C gave the α-amino acid ester which was treated with CbzCl in the presence of 7% NaHCO3 to provide the 5-amino-N-Cbz-derivative (48) in 61% overall yield. Alkaline hydrolysis of 48 followed by hydrogenation gave polyoxin C (12) in 40% overall yield, which was consistent with the reported 12.1b Likewise, ethyl uracil 5-carboxylate (49)11 was converted to the 2,4-bis(trimethylsiloxy) pyrimidine (50) which was condensed with the triacetate (42) to yield exclusively the β-nucleoside (51) in 98% overall yield. Conversion of 51 into the polyoxin C acid (18) via the Cbz-derivative (52) was achieved in 44% overall yield by the same way as for the preparation of 12 from 48.
2.4. Synthesis of left-half {Synthesis of polyoxamic acid congener (68)}12
A variety of chemical syntheses of 5-O-carbamoyl-polyoxamic acid derivatives have been reported over the years,4d, 13 one of the most important intermediate for the general synthesis of them appeared to be a (2R)-hydroxy ester such as 60 as shown in Scheme 7. We describe a convenient synthesis of the N-protected 5-O-carbamoyl-L-polyoxamic acid derivative (68) via 60 from 4-O-tert-butyldiphenylsilyl-
2,3-O-isopropylidene-L-threose (56) derived from dimethyl L-tartrate by employing an addition of vinylmagnesium bromide (Scheme 7). In seeking a practical route to 68, use of dimethyl L-tartrate with its inherent C-2 axis symmetry appeared to be the most promising. A useful synthesis of 19 utilizing L-tartaric acid has been described by Mukaiyama et al.,13f the crucial step in which was stereoselective addition of titanium silylacetylide species to the 4-O-benzyl-2,3-isopropylidene-L-threose. Our own strategy for the introduction of the α-hydroxy ester functionality involved an addition of vinylmagnesium bromide to 56 followed by oxidative cleavage of the terminal double bond as key steps. Reduction of the commercially available acetonide (53) with NaBH4 gave the diol (54) (92%), which was treated with tert-butyldipheylsilyl (TBDPS) chloride in the presence of NaH14 to afford the monosilyl ether (55) (95%). Swern oxidation of 55 provided the L-threose derivative (56) (96%) which reacted with vinylmagnesium bromide followed by acetylation to give a 53:47 diastereomeric mixture of the acetates (57) in 73% overall yield. Ozonolysis of 57 followed by treatment with Jones reagent and diazomethane afforded a diastereomeric mixture of the α-acetoxy esters (58) in 59% overall yield. This mixture was hydrolysed to a diastereomeric mixture of the α-hydroxy esters, which were separated to the less polar alcohol (2S)-59 (45%) and the more polar one (2R)-60 (54%). For the purpose of conversion of 59 into 60, treatment of 59 with trifluoromethanesulfonic anhydride afforded the triflate (61) (90%) which was treated with cesium acetate to provide the (2R)-acetoxy ester (62) (93%). Alcoholysis of (2R)-62 gave the inverted (2R)-hydroxy ester (60) (87%). Triflation of 60 followed by treatment with NaN3 afforded the diastereomerically pure (2S)-azide ester (63) (98% overall yield) which was subjected to hydrogenation and subsequent N-Boc derivation to provide the (2S)-N-Boc ester (64) (81% overall yield). Treatment of 64 with HF in pyridine gave the desilylated alcohol (65) (94%) which was subjected to carbamoylation by the reported procedure15 to furnish the ultimately desired N-protected 5-O-carbamoyl- L-polyoxamic acid ester (66) (97%). Physical data ( [α]D and NMR) of the present 66 were identical with those ([α]D and NMR) of the reported (2S,3S,4S)-66.13c Thus, the configurations of the newly generated chiral centers of α-hydroxy esters 60 and 59 were found to be (R)- and (S)-configuration, respectively. In the nucleophilic addition of vinylmagnesium bromide to the aldehyde 56, the low diastereoselectivity (53:47) was observed. For the purpose of the improvement of the diastereoselectivity, effect of coexisting metal halides in addition to 56 was examined and the results are shown in Table 2.
The anti-selective addition of nucleophile to 56 is explainable by the Felkin-Anh model16 as depicted in Figure 1. The β-chelation of metal ion enhances the Felkin selectivity. The addition of nucleophile to 56 may be controlled by the above mentioned reason since the TBDPSOCH2-group is located trans to the reacting formyl group on the dioxolane ring. The addition of vinyl magnesium bromide to 56 in the presence of ZnBr2 followed by acetylation raised anti-selective and afforded (3,4)-anti-57 (entries 4-6) as shown in Table 2. When this reaction was carried out at –78 °C, ratio of anti/syn was enhanced up to 8:1. Without ZnBr2, on the contrary, the addition was non-selective to give a 53:47 mixture of (3,4)-anti- and (3,4)-syn-57 (entry 1). For the purpose of mass production of (2R)-60, the 5:1 mixture of (3,4)-anti-57 (entry 6) was converted to 60 (52% overall yield from (3,4)-anti-57) as a main product by the same way as stated above. From viewpoint of synthetic effectiveness (diastereoselectivity and conversion yield), the present synthetic route to the desired α-azide ester (2S)-63 by way of (2S)-59 and (2R)-60 seemed to be useful because both (2S)-59 and (2R)-60 were finally converted to the important intermediate 63 for the synthesis of the N-protected (2S)-5-O-carbamoyl-L-polyoxamic acid derivative (68). For the purpose of conversion of ester group in 66 to carboxylic acid under mild conditions, transesterification of 66 with benzyl alcohol into the benzyl ester (67) in the presence of Ti(O-i-Pr)4 was achieved in 82% yield. Catalytic deprotection of benzyl group in 67 gave the desired 68 in quantitative yield, which is consistent with the reported 6813c ([α]D and NMR).
2.5. Syntheses of polyoxins L (10), J (8), B (2) and D (3)12,17
Successful coupling of uracil polyoxin C (16) with 68 was carried out by the N,N-dicyclohexylcarbodiimide-N-hydroxysuccinimide (DCC-HOSu) active ester method4a in DMSO and N,N-diisopropylethylamine as the base. Treatment of polyoxamic acid derivative (68) with DCC-HOSu gave the active ester (69) which was condensed with 16 to afford the dipeptide (70) (74% from 68). Removal of the N-Boc and O-isopropylidene protecting groups by acid hydrolysis provided polyoxin L (10) {mp 180-183 °C (decomp), ([α]D +35.0° (c=1.215, H2O) } in 94% yield. The physical properties of the present 10 were in good agreement with the literature of natural polyoxin L (10)18 {[α]D +34.4° (c=1, H2O) }.
Likewise, condensation of the active ester (69) with thymine polyoxin C (17) afforded the dipeptide (71) (74% from 68) which was converted to polyoxin J (8) {mp 195-200°C (decomp), ([α]D +35.7° (c=0.68, H2O) } in 86% yield. The physical properties of the present 8 were in good agreement with the reported polyoxin J (8) {mp 200 °C (decomp),4c [α]D +35.0° (c=0.8, H2O)4b}. Likewise, condensation of active ester (69) with polyoxin C (12) afforded the dipeptide 72 (81% from 68). Removal of the N-Boc and O-isopropylidene protecting groups by acid hydrolysis provided polyoxin B (2) {[α]D +36.0° (c=0.52, H2O), mp }150-153 °C (dec.)} in 73% yield. The physical properties of the present 2 were in good agreement with the literature of natural polyoxin B (2) ([α]D +34.0° (c=1, H2O)).1b Likewise, condensation of the active ester (69) with polyoxin C acid (18) afforded the dipeptide (73) (50% from 68) which was converted to polyoxin D (3) {([α]D +30.6° (c=0.16, H2O), mp 173-175 °C (dec.)) in 90% yield. The physical properties ([α]D , 1H-NMR and 13C-NMR) of the present 3 were identical with those {[α]D +30° (c=1, H2O),1b 1H-NMR and 13C-NMR )} of natural polyoxin D (3) given by Dr. H. Osada. The syntheses described herein demonstrate an applicable synthesis of other components of polyoxin families.1a
3. Synthesis of polyoxin M (11)19
Retrosynthetically, the synthesis of polyoxin M (11) can be achieved by amide formation between the left-half α-amino acid congener 74 and the right-half 16 as shown in Scheme 9. We describe the first synthesis of polyoxin M (11) based on the electrophilic azide transfer to chiral enolate (Scheme 9). For the synthesis of 74, (2S,4R)-2-azido-(4-protected hydroxymethyl)-4-butanolide congeners (77, 78) are thought to be an important intermediate. These azide compounds (77, 78) could be obtained by the diastereoselective azide transfer to chiral enolate derived from the (4R)-(protected hydroxymethyl)-4-butanolides (75, 76), respectively. By applying the reported method,20 the synthesis of (4R)-75 or (4R)-76 was achieved by tritylation or silylation of (4R)-γ-hydroxymethyl-γ-butyrolactone derived from D-glutamic acid. On the other hand, treatment of chiral enolate derived from N-acyloxazolidone (C) with 2,4,6-triisopropylbenzenesulfonyl azide (trisyl azide), followed by addition of AcOH was reported to give (2S)-azido carboximides (D) with high diastereoselectivity21 as shown in Scheme 9. On consideration of this report, our attention was focused only on the electrophilic azide transfer to the (4R)-4-butanolides (75, 76) (Table 3). Chiral enolate derived from (4R)-75 with lithium hexamethyldisilyazide (LiHMDS) was treated with trisyl azide, followed by addition of AcOH to give (2S,4R)-77 (37%) and (2R,4R)-77 (12%) (entry 1). Change of the counter metal cation to sodium or potassium caused decrease of the yield of (2S,4R)-77 (entries 2 and 3). Treatment of chiral enolate derived from (4R)-76 with trisyl azide, followed by addition of AcOH provided (2S,4R)-78 (33%) and (2R,4R)-78 (13%) (entry 4), while change of AcOH to trimethylsilyl chloride (TMSCl) brought about a remarkable increase of the yield of (2S,4R)-78 (53%) along with (2R,4R)-78 (28%) (entry 5). In the case of the electrophilic azide transfer to an enolate, the quench reagent was found to be an essential ingredient for successful azide transfer.21 Surprisingly, AcOH proved to be superior to the silylating agents, TMSCl or TMSOTf, or strong acid, trifluoroacetic acid, while TMSCl was found to be a more effective quench agent in the present case. The structure of (2S,4R)-78 was determined by NMR analysis including
NOE experiment. Then conversion of (2S,4R)-78 to the left-half congener (85) corresponding to 74 was carried out. Reduction of (2S,4R)-78 with Ph3P and H2O gave the amine (79) (97%), which was subjected to consecutive alkaline hydrolysis and acetal formation with formaldehyde to afford the 1,3-oxadinane derivative (80) in 70% overall yield. Protection of the secondary amino group of 80 with a Boc group gave 81 (81%), which was subjected to consecutive trans-esterification and desilylation to afford the alcohol (82) in 86% yield. Conversion of 82 to the carbamoyl compound (83) (80%), followed by catalytic hydrogenation yielded the desired carboxylic acid (84) in 98% yield. Treatment of 84 with N-hydroxysuccinimide in the presence of DCC in DMSO4a provided an active ester (85), which was coupled with uracil polyoxin C (16) in the presence of (i-Pr)2NEt to give the dipeptide (86) in 74% yield from 84. Removal of the N-Boc and N,O-acetal protecting groups upon acid hydrolysis provided polyoxin M (11) {[α]D25 +46.9° (c 0.29, H2O), mp 215–220 °C (dec) } in 47% yield. The specific rotation of synthetic 11 was in good agreement with that {[α]D +49.9° (H2O) } of the reported natural product (11)1b (Scheme 9).
4. Formal synthesis of nikkomycin B (14)22
Synthesis of nikkomycin B (14) could be achieved by the coupling of two structural units, the N-terminal amino acid (87) and the C-terminal nucleoside amino acid, uracil polyoxin C (16) as shown in Scheme 10.
The presence of three consecutive stereogenic centers in 87 is the greater synthetic challenge, and several approaches to this amino acid and its congener in either racemic or optically active form,22 have been recently reported. Barrett's intermediate (2S,3S,4S)-95 corresponding to 87 could be synthesized from chiral (2S,3S)-monoacetate 88, while uracil polyoxin C congener 97 corresponding to 16 could be obtained from the above-mentioned 43 as shown in Scheme 11.
The chiral (2S,3S)-88 was obtained by the lipase-assisted enantioselective hydrolysis of racemic 88, which was obtained by the following procedure. Reformatsky reaction of p-silyloxybenzaldehyde derived from p-hydroxybenzaldehyde and α-bromopropionate gave α-methyl-β-hydroxy ester which was subjected to Jones oxidation to give the corresponding β-keto ester. Reduction of the β-keto ester with n-Bu4NBH423 gave selectively (±)-anti-α-methyl-β-hydroxy ester (anti/syn =15/1), which was subjected to consecutive reduction and monoacetylation to afford (±)-88. Thus obtained optically pure (2S,3S)-88 was subjected to consecutive silylation, reductive deacetylation and Swern oxidation to provide the aldehyde (89). By applying Barrett's procedure,24 89 was subjected to the Felkin Ahn controlled addition of lithiated ethyl vinyl ether at -78 °C. The generated vinyl ether was directly ozonolyzed and subsequently treated with dimethyl sulfide to yield a 4.3:1 mixture of ethyl α-hydroxy esters, which was separated to 90 (47% overall yield from 88) and its diastereomer (11% overall yield from 88). Conversion of 90 to the iodide (91) (77%) followed by nucleophilic displacement with NaN3 provided the desired ethyl (2S)-α-azido ester (92) (88%) as a single diastereoisomer. Formation of the activated ester 95 was carried out in the same way as Barrett's procedure.24 Transesterification of 92 in the presence of 3-buten-1-ol and Ti(O-i-Pr)4 gave the corresponding ester 93 (90%). Ozonolysis of the butenyl moiety followed by treatment with DBU in stiu afforded the α-azido acid (94). Without further purification, treatment of 94 with p-nitrophenol in the presence of DCC gave the activated ester (95) (43%). On the other hand, uracil polyoxin C congener 97 corresponding to the C-terminal nucleoside amino acid part of nikkomycin B (14) was synthesized from 43. Hydrogenation of the azide (43) in the presence of 10% Pd-C afforded the α-amino acid ester which was treated with di-tert-butyldicarbonate (Boc2O) in the presence of triethylamine to provide the N-protected amino acid ester (96) (65%). Transesterification of 96 in the presence of benzyl alcohol and Ti(O-i-Pr)4 provided the corresponding benzyl ester (47%) along with the selective deacetylation. Benzyl ester was treated with trifluoroacetic acid to give the corresponding ammonium trifluoroacetate (97) which was directly subjected to the amide formation reaction with the activated ester 95 using N-methylmorpholine in DMF to afford the amide (98) {[α]D -10.9° (c=1.26, CHCl3), 53%}. The spectral data ([α]D, 1H-NMR, 13C-NMR and FAB-MS) of 98 were identical with those {[α]D -10.2° (c=0.98, CHCl3) } of authentic 98 reported by Barrett.24 The total synthesis of nikkomycin B (14) from 98 has already been achieved by Barrett.24
5. Conclusion
Total syntheses of polyoxins B (2), D (3), J (8), L (10), M (11), C (12) and formal synthesis of nikkomycin B (14) were summarized in this review. The efficient syntheses of 1-(5-amino-5-deoxy-β-D-allofurano-uronosyl) pyrimidines such as thymine polyoxin C (16), uracil polyoxin C (17) and polyoxin C acid (61) corresponding to the right half were achieved based on the nucleophic 1,2-addition of 1-ethoxyvinyl lithium or vinylmagnesium bromide to methyl 2,3-O- isopropylidene-β-D-ribopentodialdo-1,4-furanoside. Then the syntheses of polyoxamic acid derivatives and their congeners corresponding to the left acid part were carried out based on 1,2-addition of vinylmagnesium bromide to 4-O-protected-2,3-O-isopropylidene-L-threose. The left half acid parts were led to the corresponding activated esters, which were coupled with amine part derived from the right half to give the N,O-protected peptidyl nucleoside congeners. Deprotection of the coupled compounds afforded polyoxins B (2), D (3), J (8), L (10), M (11) and nikkomycin B (14).
ACKNOWLEDGMENT
I am grateful to Assoc. Prof. K. Kato, Dr. M. Fujii, Dr. C. Y. Chen, M.S(c). K. Uchida and M.S(c). Y. Shiro for their supporting the present works.
This paper is dedicated to Professor Emeritus Keiichiro Fukumoto on the occasion of his 75th birthday.
References
1. a) K. Isono, K. Asahi, and S. Suzuki, J. Am. Chem. Soc., 1969, 91, 7490; CrossRef b) K. Isono and S. Suzuki, Heterocycles, 1979, 13, 333. CrossRef
2. a) U. Dähn, H. Hagenmaier, M. Höhne, W. A. König, G. Wolf, and H. Zähner. Arch. Microbiol., 1976, 107, 249; CrossRef b) H. Hagenmaier, A. Keckeisen, H. Zähner, and W. A. König, Liebigs Ann. Chem., 1979, 1494; CrossRef c) W. A. König, W. Hass, W. Dehler, H. P. Fiedler, and H. Zähner, Liebigs Ann. Chem., 1980, 622; CrossRef d) H. Hagenmaier, A. Keckeisen, W. Dehler, H. P. Fiedler, H. Zähner, and W. A. König, Liebigs Ann. Chem., 1981, 1018. CrossRef
3. a) K. Kobinata, M. Uramoto, M. Nishii, H. Kusakabe, G. Nakamura, and K. Isono, Agric. Biol. Chem., 1980, 44, 1709; b) M. Uramoto, K. Kobinata, K. Isono, T. Higashijima, T. Miyazawa, E. E. Jenkins, and J. A. McCloskey, Tetrahedron Lett., 1980, 21, 3395; CrossRef c) M. Uramoto, K. Kobinata, K. Isono, T. Higashijima, T. Miyazawa, E. E. Jenkins, and J. A. McCloskey, Tetrahedron, 1982, 38, 1599. CrossRef
4. a) H. Kuzuhara, H. Ohrui, and S. Emoto, Tetrahedron Lett., 1973, 5055; CrossRef b) N. Chida, K. Koizumi, Y. Kitada, C. Yokoyama, and S. Ogawa, J. Chem. Soc., Chem. Commun., 1994, 111; CrossRef c) A. Dondoni, F Junquera, F. L. Merchan, P. Merino, and T. Tejero, J. Chem. Soc., Chem. Commun. 1995, 2127; CrossRef d) A. Dondoni, S. Franco, F. Junquera, F. L. Merchan, P. Merino, and T. Tejero, J. Org. Chem., 1997, 62, 5497. CrossRef
5. a) H. Akita, K. Uchida, and C. Y. Chen, Heterocycles, 1997, 46, 87; CrossRef b) H. Akita, K. Uchida, C. Y. Chen, and K. Kato, Chem. Pharm. Bull., 1998, 46, 1034; c) P. Merino, S. F. L. Merchan, and T. Tejero, J. Org. Chem., 2000, 65, 5575; CrossRef d) F. Li, J. B. Brogan, J. L. Gage, L. Jennifer, D. Zhang, and M. J. Miller, J. Org. Chem., 2004, 69, 4538; CrossRef e) K. E. Harding, and J. M. Southard, Tetrahedron: Asymmetry, 2005, 16, 1845; CrossRef f) T. Nishiyama, S. S. Mohile, T. Kajimoto, and M. Node, Heterocycles, 2007, 71, 1397. CrossRef
6. S. J. Danishefsky, M. P. DeNinno, G. B. Phillips, R. E. Zelle, and P. A. Lartey, Tetrahedron, 1986, 42, 2809. CrossRef
7. A. G. M. Barrett and S. A. Lebold, J. Org. Chem., 1990, 55, 3853. CrossRef
8. H. Vorbrüggen, K. Krolikiewicz, and B. Bennua, Chem. Ber., 1981, 114, 1234. CrossRef
9. a) H. Ohrui, H. Kuzuhara, and S. Emoto, Tetrahedron Lett., 1971, 4267; CrossRef b) P. Garner, and J. M. Park, J. Org. Chem., 1990, 55, 3772. CrossRef
10. K. Kato, C. Y. Chen, and H. Akita, Synthesis, 1998, 1527. CrossRef
11. H. L. Wheeler, T. B. Johnson, and C. O. Johns, Am. Chem. J., 1907, 37, 392.
12. a) H. Akita, K. Uchida, and K. Kato, Heterocycles, 1998, 47, 157; CrossRef b) K. Uchida, K. Kato, and H. Akita, Synthesis, 1999, 1678. CrossRef
13. a) H. Kuzuhara, H. Ohrui, and S. Emoto, Agr. Biol. Chem., 1973, 37, 949. ; b) H. Kuzuhara, and S. Emoto, Tetrahedron Lett., 1973, 5051; CrossRef c) A. K. Saksena, R. G. Lovey, V. M. Girijavallabham, and A. K. Ganguly, J. Org. Chem., 1986, 51, 5024; CrossRef d) P. Garner and J. M. Park. J. Org. Chem., 1988, 53, 2979; CrossRef e) I. Savage and E. J. Thomas, J. Chem. Soc., Chem. Commun., 1989, 717; CrossRef f) T. Mukaiyama, K. Suzuk, T. Yamada, and F. Tabusa, Tetrahedron, 1990, 46, 265; CrossRef g) A. Dureault, F. Carreaux, and J. C. Depezay, Synthesis, 1991, 150; CrossRef h) A. Dondoni, S. Franco, F. L. Merchan, P. Merino, and T. Tejero, Tetrahedron Lett., 1993, 34, 5479; CrossRef i) J. A. Marshall, B. M. Seletsky, and P. S. Coan, J. Org. Chem., 1994, 59, 5139; CrossRef j) F. Matsuura, Y. Hamada, and T. Shioiri, Tetrahedron Lett., 1994, 35, 733; CrossRef k) R. F. W. Jackson, N. J. Palmer, M. J. Wythes, W. Clegg, and M. R. J. Elsegood, J. Org. Chem., 1995, 60, 6431; CrossRef l) B. M. Trost, A. C. Krueger, R. C. Bunt, and J. Zambrano, J. Am. Chem. Soc., 1996, 118, 6520. CrossRef
14. P. G. McDouga, J. G. Rico, Y. -I. Oh, and B. D. Condon, J. Org. Chem., 1986, 51, 3388. CrossRef
15. F. Tabusa, T. Yamada, K. Suzuki K, and T. Mukaiyama, Chem. Lett., 1984, 405. CrossRef
16. M. Cherest, H. Felkin, and N. Prudent, Tetrahedron Lett., 1968, 2199. CrossRef
17. K. Uchida, K. Kato, K. Yamaguchi, and H. Akita, Heterocycles, 2000, 53, 2253. CrossRef
18. K. Isono, K. Kobinata, and S. Suzuki, Agr. Biol. Chem., 1968, 32, 792.
19. Y. Shiro, K. Kata, M. Fujii, Y. Ida, and H. Akita, Tetrahedron, 2006, 62, 8687. CrossRef
20. M. Taniguchi, K. Koga, and S. Yamada, Tetrahedron, 1974, 30, 3547. CrossRef
21. D. A. Evans and T. C. Britton, J. Am. Chem. Soc., 1987, 109, 6881. CrossRef
22. a) H. Akita, Cheng Yu Chen, and K. Uchida, Tetrahedron: Asymmetry, 1995, 6, 2131; CrossRef b) H. Akita, C. Y. Chen, and K. Kato, Tetrahedron, 1998, 54, 11011. CrossRef
23. M. Taniguchi, H. Fujii, K. Oshima, and K. Uchimoto, Tetrahedron, 1993, 49, 11169. CrossRef
24. A. G. M. Barrett and S. A. Lebold, J. Org. Chem., 1991, 56, 4875. CrossRef