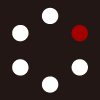
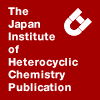
HETEROCYCLES
An International Journal for Reviews and Communications in Heterocyclic ChemistryWeb Edition ISSN: 1881-0942
Published online by The Japan Institute of Heterocyclic Chemistry
e-Journal
Full Text HTML
Received, 6th November, 2008, Accepted, 18th December, 2008, Published online, 22nd December, 2008.
DOI: 10.3987/COM-08-11592
■ Kinetic Study on Huisgen Reaction Catalyzed by Copper(I): Triazol Formation from Water-Soluble Alkyne and Alkyl Azide
Yosuke Kasuga, Mamoru Ito, Wataru Onoda, Yosuke Nakamura, Seiichi Inokuma, Takehisa Matsuda, and Jun Nishimura*
Department of Chemistry, Gunma University, 1-5-1 Tenjin-cho, Kiryu 376-8515, Japan
Abstract
The kinetics was examined for the copper(I)-catalyzed Huisgen reaction. Despite the complex reaction pathway, it was successfully analyzed by the second-order kinetics with equal amounts of two organic substrates in the presence of 0.1 equivalent of copper catalyst, after dividing the time course into two stages, the early and late ones. Both stages gave almost the same activation parameters. The reaction of water-soluble alkyl azide (1) was 60 times as fast as that of water-soluble aromatic azide (4) at the early stage and surprisingly 822 times after 15 min reaction time at 30 ˚C.INTRODUCTION
Click chemistry has been attracting much attention in not only organic and biological chemistry field but also polymer and materials science filed.1-7 In the chemistry, copper(I)-catalyzed Huisgen reaction8-11 between alkynes and alkyl azides has been successfully utilized, because of its simplicity, high performance, and selectivity. In fact this catalyzed reaction usually affords only anti-triazole among two possible regioisomers.8-11
Despite a variety of reports on the reaction, only a few research on its kinetics has been so far disclosed, because of the rather complicated reaction mechanism.12,13 Sharpless and his coworkers reported a theoretical computational chemical approach to solve the mechanism.12 They showed the cyclization by the C-N bond formation on alkyne-alkyl azide-Cu(I) complex as the rate-determining step. The mechanism seems to be simple for a kinetic approach using the second-order kinetic equation of alkyne and alkyl azide, although the previous research13 showed that the reaction is first-order in benzyl azide and 1.3 ± 0.2 order in phenylacetylene in the presence of excess copper in DMSO-H2O 4:1 mixed solution.
In the materials science, moreover, fast reactions are always being seeked for.14 Therefore, it is rather important to know how faster an organic azide reacts with acetylene derivatives than another organic azide. If a facile, general or even apparent kinetic approach would be established for the reaction and then related data would be accumulated, some important guidelines would be given to solve individual problems. Thus we had been prompted to carry out a kinetic examination of the reactions between water-soluble organic substrates.
RESULTS
Design of Substrates
In order to simplify the reaction system, water-soluble materials were employed as copper catalyst, reductant, alkyne, and alkyl azide. Then the reaction media can be pure water, but not mixed solvents such as DMSO-water. As shown in Scheme 1, we designed and prepared material 1 having a hydroxy group and an oligooxyethylene moiety as alkyl azide and chose propargyl alcohol (2) as alkyne. Moreover we prepared water-soluble ester (4) as an aromatic azide for comparison. These materials are considerably soluble in water. Actually we carried out the kinetic measurement on the reaction in water with 0.1 M of each substrate concentration as shown in Scheme 1 (the last processes using reaction condition c).
Catalyst Concentration for Kinetic Measurement
The reaction rate considerably depends on the copper(I) catalyst concentration, so that we first determined the catalyst concentration suitable for the kinetic study. From the data obtained by changing the catalyst concentration, 0.1 equivalent relative to the organic substrates was selected because the rate observed was reasonably fast to be determined. The initial rate constant divided by the catalyst concentration was constant, kapp/[cat] = (2.5 ± 0.1) x 102 M-2 s-1 when the catalyst concentration was 0.02 to 0.1 equivalent to the substrates at 30 ˚C. Therefore, the catalyst concentration was kept constant during the measurement.
Quantitative Analysis of Triazoles Produced
Among several spectroscopic and chromatographic methods examined for the quantitative analysis of the product triazoles (3) and (5), HPLC equipped with an ODS column (methanol eluent) was found the most practically useful. Using the chromatogram peak areas of the triazoles, calibration curves were obtained with the correlation coefficient r > 0.999.
Kinetic Measurements
In the equimolar case of substrates 1 and 2 with 0.1 equivalent copper(I) catalyst determined as above, the kinetics was analyzed using equation (1);
x/a(a-x) = k2t (1)
where a is the initial concentration of each organic substrate, x is the concentration at time t or (a-x) is the concentration of triazole obtained at time t, k2 is the apparent rate constant, and t is the reaction time.
All of time-course plots show a flection region as recognized in Figure 1a (2400 s at 0 ˚C; 600 s at 10 ˚C; 240 s at 20 ˚C; 120 s at 30 ˚C) so that the overall second-order treatment failed to give any straightforward rate constant with a high correlation coefficient r. Eventually the time-course plots were divided into two stages; the first one as the early stage (stage E) and the second one as the late stage (stage L). Both stages now give fine linear plots with r > 0.92 as depicted in Figure 1b and c. The rate constants obtained are listed in Table 1.
As mentioned above, the equimolar reaction between substrates 4 and 2 in the presence of 0.1 equivalent copper(I) catalyst was also analyzed. The reaction was quite slow compared with that between 1 and 2, so that no flection point was recognized within 15 min at 0 to 30 ˚C as shown in Figure 2. The reaction could be analyzed by the second-order kinetics throughout its process to the 20% triazole yield. The rate constants obtained are listed in Table 2, which are considered for stage E.
Activation Parameters
For the early and late stages of the reaction between 1 and 2, activation parameters were calculated by using Arrhenius and Eyring equations as shown in Figures 3 and 4, respectively. Both stages gave almost the same activation parameters, as shown in Tables 3 and 4.
For the reaction between 4 and 2, the activation parameters were also calculated by using Arrhenius and Eyring equations as shown in Figures 5 and 6, respectively. The obtained values are listed in Table 5.
DISCUSSION
Sharpless and his coworkers reported a plausible detailed mechanism for the copper-catalyzed Huisgen reaction12 by using the MO calculation. The catalytic cycle begins with the coordination of the alkyne to the Cu(I) as shown in Scheme 2. This step was calculated exothermic by ca. 49.0 kJ mol-1.12 When acetonitrile is a ligand, the displacement process is slightly endothermic by 2.5 kJ mol-1. This is in good agreement with the experimental observation that the reaction proceeds much faster in aqueous solution. The conversion of alkyne I to acetylide II is well known reaction. The azide replaces one of the ligands and binds to the copper atom via the nitrogen proximal to carbon, forming intermediate III (step B in Scheme 2). This ligand exchange step was calculated slightly exothermic (8.4 kJ mol-1 when L is water).12 Next, the distal nitrogen of the azide in III attacks the C-2 carbon of the acetylide, forming the unusual six-membered copper(III) metallacycle IV. This step is rate-determing one, endothermic by 52.7 kJ mol-1 when L is water, and the calculated barrier is 78.3 kJ mol-1, which is closely similar to that of the present species formed from the reaction of 1 with 2 (see Table 3). This calculated value of 78.3 kJ mol-1 is considerably lower than the barrier for the uncatalyzed reaction (108.8 kJ mol-1).12 This explains the enormous rate acceleration of the copper(I)-catalyzed process, 7 to 8 oders of magnitude, as compared to the purely thermal cycloaddition. The barrier for ring contraction of IV forming triazole-copper derivarive V is very low. With water as ligand, the transition state is 13.4 kJ mol-1 higher than the intermediate. Species V releases the triazole.
Essentially, an alkyne initially reacts with copper(I) species and alkyl azide coordinates on acetylide-copper(I) atom with a low activation energy, and then both ligands cyclize to give the corresponding triazole with a high activation energy. Kinetically the overall processes with equal amounts of substrates could be analyzed by the second-order kinetic equation (1) on the two organic substrates as the present work shows.
On this kinetic study, the concentration of copper(I) catalyst generated in situ by sodium ascorbate is mainly concerned with whether copper(II) in situ is reduced completely and the reduced form copper(I) is sustained during the reaction time. Otherwise the concentration prescribed could not be proved. Hence, in order to keep the concentration constant, we took the Huisgen reaction time as short as possible, carried out the reaction carefully under a nitrogen atmosphere, and used a plenty of time for the reduction of copper(II) by sodium ascorbate until the copper ion color changed completely from blue to brown. The Huisgen reaction time was set by using the appropriate concentration of the catalyst as mentioned above, and preliminary experiments showed that its 0.1 equivalent relative to the organic substrates is sufficient and the reaction can be fast enough to be monitored within 15 min at 0 to 30 ˚C.
As shown in Figure 1, all of time-course plots have a flection region. The origin would not be due to the continuous increase of copper(I) catalyst concentration or any other continuously changing events, because such a change could be reflected as a rather smooth plot, not as that with a flection region. After the reaction was completed, equimolar alkyl azide and alkyne were again poured into the same reaction vessel. We observed almost the same rate constant for this second run as that of stage L.
For the reaction between 4 and 2, no flection region could be observed within 1 h. Since Sharpless and his coworkers reported that tristriazole (6) enhances the copper(I)-catalyzed Huisgen reaction by its coordination on copper catalyst,15 the flection point seems to be due to the enhanced activity of the catalyst by the coordination of triazole (3) produced. After the yield of the triazole becomes more than 20-30%, most of azide coordination is assumed to occur on the copper(I) having the triazole as a ligand in aqueous media due to a kind of hydrophobic effect or micro micelle formation related to a phenomenon such as critical micelle concentration which is not of a continuously changing phenomenon. Then the cyclization between substrate ligands occurs under the enhanced catalytic environment. Both of the advantages, the higher concentration of substrates in a micelle and the enhancement of catalytic activity, are believed to work cooperatively to result in the rate enhancement and making the flection point. Of course the former phenomenon is believed more significant. Now the origin for the enhancement is being investigated and will be reported elsewhere in a near future.
It is also reasonable that the product-forming step on both aquocomplex and enhanced triazole-coordinated copper(I) catalysts has the same activation parameters, because the cyclization occurs at the C and N ends of the substrate ligands and the step microscopically is the rate-determining one, then followed by the fast release of triazole produced to the aqueous media. It is stressed, however, that the complex sequence of events became the second-order kinetic figure as analyzed here experimentally.
From the kinetic examination of the copper(I)-catalyzed Huisgen reaction, the following remarks are stressed:
1) The importance of copper(I) catalyst for the Huisgen reaction is again confirmed by changing its concentration. For this kinetic experiment, 0.1 equivalent of the catalyst works as desired.
2) The time course of the reaction in aqueous media shows a flection region around the 20-30% triazole formation. This seems to be due to the reaction enhancement by the micelle formation or other coagulation in water as well as the triazole coordination to copper(I) catalyst. The reaction was successfully analyzed separately before and after the flection region.
3) The activation parameters for both early and late stages are the same as each other. This is because the thermodynamic aspects of both transition complexes are the same due to passing through the same rate-determining step from the microscopic viewpoint, although their ligands seem different from each other, aquo or triazole.
4) For preparing functional materials, the reaction can be adjusted fast enough so that it can complete within several minutes, by choosing the substrates, catalyst concentration, reaction media, temperature, and so forth. The reaction of alkyl azide (1) as a substrate is faster than that of aromatic azide (4) by a factor of 60 (between the early stages) to 822 (the early one vs. the late one) at 30 ˚C.
5) Of course when the materials are applied to a medical use, the reaction for the preparation has a considerable drawback because of toxic copper as the necessary catalyst.16
EXPERIMENTAL
General
Elementary analysis was done at Gunma Industrial Technology Center. 1H NMR spectra were taken with a JEOL AL-300 FT NMR spectrometer and ESI-Mass spectra were recorded by a PARKIN ELMER API-100.
For HPLC analysis a Shimadzu LC-9A liquid chromatograph and Shimadzu SPD-6 UV spectrometer were used, equipped with a Shim-pack PREP-ODS column. For the isolation of products a JAI LC-918 recycling preparative HPLC was used equipped with JAIGEL-1H column. For a column chromatography silica gel 60 N (mesh 63-210 mm) was used, obtained from Kanto Kagaku Co. Ltd. Distilled water was supplied by an IWAKI ASK-2DS automatic still.
Solvents, THF and toluene, were dried over molecular sieves 4A provided by Wako Pure Reagent Co. Ltd. Acetonitrile was distilled before use. Dichloromethane was used as received.
Pure reagents like 4-azidobenzoic acid, tetraethylene glycohol, sodium ascorbate, p-toluenesulfonyl chloride, p-toluensulfonic acid, CuSO4-5H2O, NaOH, NaN3, and anhydrous Na2SO4 were commercially available and used without purification.
Preparation of Alkyl azide
Tetraethylene glycol monotosylate (52.6 g, 1.51 x 10-1 mol)17 and sodium azide (29.5 g, 4.53 x 10-1 mol) was dissolved in dry distilled MeCN (315 mL) and heated for 36 h under reflux. After cooling down to rt, the reaction mixture was extracted three times by CH2Cl2 (3 x 300 mL). The extracted solution was washed by water (3 x 300 mL) and dried over anhydrous Na2SO4. After the solution was evaporated carefully and the residue was purified by silica gel column chromatography using a gradient mixed solvent of toluene and EtOAc, desired azide (1)18 was obtained as yellowish viscous liquid in 21.4 g (65% yield). The treatment of the azide at storage, weighing, and so forth, was done by extra order of care.
1H NMR (CDCl3, 300 MHz) δ = 3.40 (2H, m), 3.60-3.74 (14H, m).
By the same procedure aromatic azide 4 was obtained as yellowish viscous liquid in 57% yield. 1H NMR (CDCl3, 300 MHz) δ= 3.59-3.73 (12H, m), 3.83 (2H, m), 4.47 (2H, m), 7.07 (2H, d, J = 8.7 Hz), 8.05 (2H, d, J = 11.4 Hz).
Preparation of Triazole
Authentic triazoles were obtained as followed: In an aqueous solution (30 mL) of alkyl azide 1 (1.0 g, 4.56 x 10-3 mol) obtained as above was dissolved copper sulfate pentahydrates (0.114 g, 0.46 x 10-3 mol) and then sodium ascorbate (0.446 g, 2.28 x 10-3 mol). After the complete dissolving the reductant, aqueous solution (15 mL) of propargyl alcohol (2) (0.256 g, 4.56 x 10-3 mol) was added to the above solution. Under a nitrogen atmosphere the reaction mixture was stirred for 1 h and then evaporated. The remaining residue was extracted by CH2Cl2 (100 mL). The extracted solution was evaporated, and purified by silica gel column chromatography using a gradient mixed solvent of toluene and EtOAc and GPC. The desired yellowish viscous triazole (3) was characterized by 1H NMR and ESI-MS experiments. The material recorded at least 95% purity. 1H NMR (CDCl3, 300 MHz) δ = 3.57-3.74 (14H, m), 3.87 (2H, m), 4.56 (2H, m), 4.79 (2H, s), 7.86 (1H, s). Anal. Calcd for C11H24N3O5.5 (3‧1.5H2O): C 42.44, H 8.09, N 13.50. Found: C 42.94, H 8.34, N 13.71. (+) ESI/MS: calcd for 297.14 (M + Na) +, m/z = 298.2 (M + Na)+.
Authentic yellowish viscous aromatic triazole (5) was also obtained as above in 58% yield. 1H NMR (CDCl3, 300 MHz) δ = 3.58-3.73 (12H, m), 3.86 (2H, m), 4.52 (2H, m), 4.90 (2H, s), 7.83 (2H, d, J = 8.7 Hz), 8.10 (1H, s), 8.22 (2H, d, J = 8.7 Hz). Anal. Calcd for C18H29N3O9 (5‧2H2O): C 51.49, H 6.91, N 9.01. Found: C 51.35, H 6.66, N 9.58. (+) ESI/MS: calcd for 417.16 (M + Na)+, m/z = 418.1 (M + Na)+.
Kinetic Measurement
The calibration curves for authentic triazoles were obtained for HPLC chromatograph. Six samples were prepared with different triazole concentration. The peak area for 20 µL injection vs. concentration plots show the straight line with r more than 0.999
The procedure for the kinetic measurement was as follows:
1) The reaction vessel #1 having 0.1 mol L-1 aqueous solution (30 mL) of azide (1 or 4) was set in a temperature-controlled bath. Into the solution the prescribed amount of CuSO4‧5H2O was completely dissolved by magnetic stirring. After dissolving the prescribed amount of sodium ascorbate (5 equivalent to copper) was dispersed in the reaction solution.
2) Another container #2 having 0.1 mol L-1 aqueous solution (15 mL) of propargyl alcohol (2) was also set in the temperature-controlled bath.
3) After certified the color change from blue to brown in the vessel #1, the propargyl alcohol aqueous solution was added all at once by using a hypodermic syringe.
4) Aliquots at the prescribed periods were taken from the reaction mixture and poured into the 0.1 mol L-1 hydrogen peroxide solution in order to cease the reaction.
5) Precipitated some solid material by centrifugation, each sample (20 µL injection) was analyzed by HPLC equipped with an ODS column and eluted by MeOH.
References
1. H. C. Kolb, M. G. Finn, and K. B. Sharpless, Angew. Chem. Int. Ed., 2001, 40, 2004. CrossRef
2. H. C. Kolb and K. B. Sharpless, DDT, 2003, 8, 24.
3. Q. Wang, S. Chittaboina, and H. N. Barnhill, Lett. Org. Chem., 2005, 2, 293. CrossRef
4. V. D. Bock, H. Hiemstra, and J. H. Maarseveen, Eur. J. Org. Chem., 2006, 51. CrossRef
5. J. F. Lutz, Angew. Chem. Int. Ed., 2007, 46, 1018. CrossRef
6. I. Aprahamian, O. S. Miljanic, W. R. Dichtel, K. Isoda, T. Yasuda, T. Kato, and J. F. Stoddart, Bull. Chem. Soc. Jpn., 2007, 80, 1856. CrossRef
7. M. V. Gil, M. J. Arevalo, and O. Lopez, Synthesis, 2007, 11, 1589. CrossRef
8. V. V. Rostovtsev, L. G. Green, V. V. Fokin, and K. B. Sharpless, Angew. Chem. Int. Ed., 2002, 41, 2596. CrossRef
9. C. W. Tornøe, C. Christiensen, and M. Meldal, J. Org. Chem., 2002, 67, 3057. CrossRef
10. For Ru-catalyzed reaction, see A. H. Yap and S. M. Weinreb, Tetrahedron Lett., 2006, 47, 3035. CrossRef
11. For original Huisgen reaction, see R. Huisgen, In 1,3-Dipolar Cycloadditional Chemistry, ed. by A Padwa, Wiely, New York, 1984, 1.
12. F. Himo, T. Lovell, R. Hilgraf, V. V. Rostovstev, L. Noodleman, and K. B. Sharpless, J. Am. Chem. Soc., 2005, 127, 210. CrossRef
13. V. O. Rodionov, V. V. Fokin, and M. G. Finn, Angew. Chem. Int. Ed., 2005, 44, 2210. CrossRef
14. T. Matsuda and T. Ito, Jpn Tokkyo Koho JP2003-38634.
15. T. R. Chan, R, Hilgraf, K. B. Sharpless, and V. V. Fokin, Org. Lett., 2004, 6, 2853. CrossRef
16. J. A. Codelli, J. M. Baskin, N. J. Agard, and C. R. Bertozzi, J. Am. Chem. Soc., 2008, 130, 11486. CrossRef
17. J. G. Heffernan, W. M. MacKenzie, and D. C. Sherrington, J. Chem. Soc., Perkin Trans. 2, Phys. Org. Chem., 1981, 514. CrossRef
18. T. Gartiser, C. Selve, and J. J. Delpuech, Tetrahedron Lett., 1983, 24, 1609. CrossRef