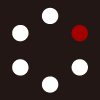
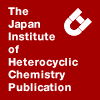
HETEROCYCLES
An International Journal for Reviews and Communications in Heterocyclic ChemistryWeb Edition ISSN: 1881-0942
Published online by The Japan Institute of Heterocyclic Chemistry
e-Journal
Full Text HTML
Received, 19th December, 2008, Accepted, 19th February, 2009, Published online, 20th February, 2009.
DOI: 10.3987/COM-08-11635
■ Diketopiperazine Supramolecule Derived from Hydroxyphenylglycine
Yosuke Ohta, Kayo Terada, Toshio Masuda, and Fumio Sanda*
Department of Polymer Chemistr, Graduate School of Engineering, Kyoto University, Kyoudai-katsura, Nishikyo, Kyoto 615-8510, Japan
Abstract
A diketopiperazine (DKP) having long alkyl chains was synthesized from D-p-hydroxyphenylglycine, and the formation of supramolecules was examined. The 1H NMR and UV-vis spectroscopic measurements and molecular modeling have suggested that the DKP molecules are aligned based on hydrogen bonding between the amide groups, and there is no stacking between the phenyl groups.INTRODUCTION
Supramolecules are molecular assemblies formed by noncovalent interaction such as hydrogen bonding, van der Waals interaction, and electrostatic interaction.1–4 Among a wide variety of supramolecules, polymeric assemblies formed by repeated intermolecular interactions between monomeric compounds are defined as supramolecular polymers. They attract much attention due to the unique properties such as self-healing and stimuli-responsiveness based on the supramolecular nature, and rheological and mechanical properties based on the polymeric architecture as well.5,6
Diketopiperazine (DKP, Figure 1), a cyclic amino acid dimer, is a typical by-product in peptide synthesis. It has two s-cis secondary amide groups, which can hydrogen bond horizontally along the ring plane. In fact, some DKPs form aggregates based on tandem hydrogen bonding between the amide groups in the solid state.7 Such aggregates show liquid crystallinity8 and form microcapsules.9 Phenylalanine-, aspartic and glutamic acid-based DKPs serve as oil gelators,10 wherein intermolecular hydrogen bonding plays a key role to form molecular networks. We have recently synthesized DKP-containing polyamides and polyesters with moderate molecular weights.11 We have also reported the acyclic diene metathesis polycondensation of glutamic acid DKP ω-alkenyl esters with ruthenium catalysts.12 The formed polymers are associated in the solid and solution states based on hydrogen bonding between the DKP moieties.
In the course of our study on DKP-based polymers, we have started investigating the synthesis of supramolecular polymers consisting of DKP derivatives. Herein, we wish to report the synthesis of a DKP from D-p-hydroxyphenylglycine, and formation of supramolecules.
RESULTS AND DISCUSSION
Common DKPs are poorly soluble in organic solvents due to the aggregation by intermolecular hydrogen bonding between the amide groups. This is important for DKPs to form supramolecular structures as described above, but solubility to a certain extent is preferable for examining the association property spectroscopically. We therefore designed a symmetrical DKP 4 having long alkyl chains substituted at the benzene ring via the ether linkage to enhance the solubility. Scheme 1 illustrates the synthetic route for 4 starting from D-p-hydroxyphenylglycine. DKP 4 was successfully synthesized by direct cyclization of precursor 3 through intramolecular ester-amide exchange reaction by heating at 80 °C for 5 days. The structure was confirmed by 1H NMR, 13C NMR, and IR spectroscopies besides high-resolution mass spectrometry. It was soluble in chloroform and 1,1,2,2-tetrachloroethane at high temperature. The long alkyl chains seem to be effective for enhancing the solubility of 4 as expected.
The intermolecular interaction of 4 was examined by 1H NMR spectroscopy. Figure 2 depicts the 1H NMR spectra measured at various concentrations (1–40 mM) in 1,1,2,2-tetrachloroethane-d2 at 65–100 °C. Since 4 was poorly soluble in chloroform at room temperature, 1,1,2,2-tetrachloroethane-d2 was used as the measurement solvent. The boiling point of 1,1,2,2-tetrachloroethane-d2 (147 °C) higher than that of chloroform-d (61 °C) was also suitable for temperature-variable NMR measurement. The chemical shift of the amide NH proton signal depended on both the concentration and temperature as shown in Figure 2. Increase in concentration and decrease in temperature resulted in downfield shift of the signal, both of which indicate the formation of intermolecular hydrogen bonding between the amide groups.13
The UV-vis spectra of 4 were also measured at various concentrations and temperatures. No significant
shift of absorption maximum wavelength was observed (Figure 3). This result suggests the absence of intermolecular π-stacking between the benzene rings. This is in good agreement with no change of the chemical shift of the phenyl proton in the 1H NMR spectra measured at various concentrations and temperatures.
Figure 4 depicts a possible supramolecular structure consisting of 4 based on the results of 1H NMR and UV-vis spectroscopic measurements. The geometries were optimized by the molecular mechanics method using the MMFF94 forcefield.14 The molecules were aligned horizontally to the DKP rings by hydrogen bonding between the amide groups. The average distance between the benzene rings was 5.8 Å, coincident with the absence of π-stacking. It is implied that hydrophobic interaction between the dodecyl groups contributes to stabilize the supramolecular structure.
In summary, we have demonstrated the synthesis of novel DKP 4 having long alkyl chains. DKP 4 was assembled in tetrachloroethane by hydrogen bonding between the amide groups, while the benzene rings did not directly contribute to the aggregation via π-stacking, which was supported by 1H NMR and UV-vis spectroscopies along with the conformational analysis by the molecular mechanics calculation. It is expected that non-aromatic DKPs substituted with branched alkyl chains show solubility higher than the one in the present study, leading to the development of supramolecules that show chiroptical properties such as liquid crystallinity. Further molecular design of DKP-based supramolecules is under progress.
EXPERIMENTAL
Measurements. 1H and 13C NMR spectra were recorded on a JEOL EX-400 spectrometer. Melting points (mp) were measured on a Yanaco micro melting point apparatus. UV-vis spectra were measured in a quartz cell (thickness: 1 cm) at room temperature using a JASCO J-800 spectropolarimeter.
Materials. All the reagents in monomer synthesis were used as purchased without purification. DMF was distilled over calcium hydride.
Synthesis. D-p-Hydroxyphenylglycine methyl ester hydrochloride (1) Thionyl chloride (13 mL) was added to MeOH (50 mL) at –10 °C and the solution was stirred for 10 min. D-p-Hydroxyphenylglycine (8.36 g, 50.0 mmol) was added to the solution and the resulting mixture was stirred at rt overnight. It was concentrated and the residual mass was washed with Et2O, and recrystallized with Et2O/MeOH to obtain 1 as a colorless solid in 94%. 1H NMR (400 MHz, DMSO-d6): δ 3.70 [s, 3H, –CO2CH3], 5.10 [s, 1H, –NCH<], 6.83–7.30 [m, 4H, Ar], 8.94 [s, 3H, –NH3], 9.93 [s, 1H, –ArOH].
N-tert-Butoxycarbonyl-D-p-hydroxyphenylglycine-O-methyl ester (2) Di-tert-butylcarbonate [(Boc)2O, 10.3 g, 47.2 mmol] and triethylamine (67.0 mL, 481 mmol) were added to a solution of 1 (10.2 g, 46.9 mmol) in CH2Cl2 (400 mL) at 0 °C and the solution was stirred at rt overnight. It was concentrated, and then the residue was dissolved in Et2O and washed with water twice. The organic layer was dried over anhydrous MgSO4 and concentrated to obtain 2 as a yellow solid in 87%. 1H NMR (400 MHz, CDCl3): δ 1.43 [s, 9H, –OC(CH3)3], 3.71 [s, 3H, –CO2CH3], 5.05 [s, 1H, OH], 5.23 [s, 1H, –NCH<], 5.49 [s, 3H, NH], 6.73–7.18 [m, 4H, Ar].
N-tert-Butoxycarbonyl-D-p-dodecoxyphenylglycine methyl ester (3) Dodecyl bromide (9.8 mL, 40.9 mmol) was added to a mixture of 2 (11.6 g, 41.2 mmol) and K2CO3 (5.67 g, 41.0 mmol) in distilled DMF using a dropping funnel at rt under nitrogen. The resulting mixture was heated to 60 °C to be transparent and stirred for 21 h. The precipitate formed was filtered off and the filtrate was concentrated in vacuo. It was purified by column chromatography eluted with EtOAc to obtain 9 as a viscous liquid in 76%. 1H NMR (400 MHz, CDCl3): δ 0.88 [t, J = 6.6 Hz, 3H, –OCH2CH2(CH2)9CH3], 1.26 [m, 18H, –OCH2CH2(CH2)9CH3], 1.43 [s, 9H, –OC(CH3)3], 1.76 [t, J = 7.2 Hz, 2H, –OCH2CH2(CH2)9CH3], 3.71 [s, 3H, –CO2CH3], 3.93 [t, J = 6.6 Hz, 2H, –OCH2CH2(CH2)9CH3], 5.23 [s, 1H, –NCH<], 5.48 [s, 1H, >NH], 6.85–7.27 [m, 4H, Ar].
cyclo(D-p-Dodecoxyphenylglycinyl-D-p-dodecoxyphenylglycinyl) (4) Trifluoroacetic acid (TFA, 12 mL, 162 mmol) was added to a solution of 3 (14.1 g, 31.4 mmol) in CH2Cl2 (300 mL) using a dropping funnel at 0 °C, and the resulting mixture was stirred at rt overnight. CH2Cl2 and TFA were distilled off in vacuo, and triethylamine (0.2 mL, 1.44 mmol) was added to a solution of the residual mass (446 mg, 1 mmol) in toluene (1 mL) and the reaction mixture was heated to 80 °C for 5 days. After cooling to rt, the precipitate formed was separated by filtration to obtain 4 as a colorless solid in 41%. 1H NMR (400 MHz, 1,1,2,2-tetrachloroethane-d2): δ 0.87 [t, J = 6.6 Hz, 6H, –OCH2CH2(CH2)9CH3], 1.26–1.43 [m, 36H, –OCH2CH2(CH2)9CH3], 1.78 [t, J = 7.2 Hz, 4H, –OCH2CH2(CH2)9CH3], 3.95 [t, J = 6.6 Hz, 4H, –OCH2CH2(CH2)9CH3], 5.06 [s, 2H, >NCH<], 5.94 [s, 2H, >NH], 6.86–7.30 [m, 8H, Ar]. 13C NMR (100 MHz, 1,1,2,2-tetrachloroethane-d2): δ 14.37 [–OCH2CH2(CH2)9CH3], 22.92, 26.28, 29.55, 29.85, and 32.14 [–OCH2CH2(CH2)9CH3], 68.69 [–OCH2CH2(CH2)9CH3], 74.77 [>NCH<], 115.65 [Ar], 128.53 [Ar], 128.65 [Ar], 160.11 [Ar], 167.01 [C=O]. IR (cm-1, KBr): 3198 (NH), 3094 (Ar), 2955 (CH), 2918 (CH), 2850 (CH), 1667 (NHCO), 1515, 1457, 1258, 822. Mp 218–220 °C (decomp). HRMS. Calcd for C24H32N2O4 (m/z) 634.4710. Found: 634.4709.
References
1. A. R. A. Palmans and E. W. Meijer, Angew. Chem. Int. Ed., 2007, 46, 8948. CrossRef
2. R. P. Sijbesma and E. W. Meijer, Chem. Commun., 2003, 5. CrossRef
3. M. A. Mateos-Timoneda, M. Crego-Calama, and D. N. Reinhoudt, Chem. Soc. Rev., 2004, 33, 363. CrossRef
4. W. H. Binder, Monat. Chem., 2005, 136, 1. CrossRef
5. L. Brunsveld, B. J. B. Folmer, E. W. Meijer, and R. P. Sijbesma, Chem. Rev., 2001, 101, 4071. CrossRef
6. L. Bouteiller, Adv. Polym. Sci., 2007, 207, 79. CrossRef
7. Y. Du, J. C. Creighton, B. A. Tounge, and A. B. Reitz, Org. Lett., 2004, 6, 309. CrossRef
8. R. A. Kloster, M. D. Carducci, and E. A. Mash, Org. Lett., 2003, 5, 3683. CrossRef
9. R. J. Bergeron, O. Phanstiel IV, G. W. Yao, S. Milstein, and W. R. Weimar, J. Am. Chem. Soc., 1994, 116, 8479. CrossRef
10. K. Hanabusa, H. Fukui, M. Suzuki, and H. Shirai, Langmuir, 2005, 21, 10383. CrossRef
11. K. Terada, F. Sanda, and T. Masuda, J. Macromol. Sci., Part A: Pure Appl. Chem., 2007, 44, 789. CrossRef
12. K. Terada, E. B. Berda, K. B. Wagener, F. Sanda, and T. Masuda, Macromolecules, 2008, 41, 6041. CrossRef
13. R. A. Weatherhead-Kloster, H. D. Selby, W. B. Miller III, and E. A. Mash, J. Org. Chem., 2005, 70, 8693. CrossRef
14. T. A. Halgren, J. Comput. Chem., 1996, 17, 490; CrossRef The molecular mechanics calculation was carried out with Wavefunction Inc., Spartan ’06 Windows.