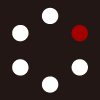
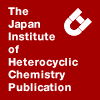
HETEROCYCLES
An International Journal for Reviews and Communications in Heterocyclic ChemistryWeb Edition ISSN: 1881-0942
Published online by The Japan Institute of Heterocyclic Chemistry
e-Journal
Full Text HTML
Received, 23rd January, 2009, Accepted, 24th February, 2009, Published online, 25th February, 2009.
DOI: 10.3987/REV-09-652
■ Recent Advances in the Syntheses of Biologically Active Natural Products Using Biocatalyst
Hiroyuki Akita*
School of Pharmaceutical Sciences, Toho University, 2-2-1, Miyama, Funabashi, Chiba 274-8510, Japan
Abstract
This review summarizes the chemoenzymatic synthesis of biologically active natural products based on a combination of chemical diastereoselectivity and enzymatic enantioselectivity using lipase. Diastereoselective synthesis of (±)-(2,3)-syn-2-methyl-3-hydroxy ester I or (±)-(2,3)-syn-3-methyl-2-hydroxy ester III was achieved based on diastereoselective reduction of (±)-2-methyl-3-keto ester IV or the reaction of (±)-trans-epoxybutanoate V and carbon-nucleophile, respectively. These racemic alcohols were subjected to enzymatic resolution to afford the corresponding enantiomers. Each enantiomerically pure compound was converted to biologically active natural products such as oudemansins, chuangxinmycin, asperlin, indolmycin, cystothiazoles, melithiazols and myxothiazols possessing antifungal and cytotoxic activities, inhibition of NADH oxidation, etc.1. INTRODUCTION
In connection with synthetic studies of polyketides such as polyoxomacrolide and polyether antibiotics, stereocontrolled reaction in acyclic systems have been extensively investigated. Among them, the stereoselective synthesis of these natural products has attracted the attention of many synthetic organic chemists, since the syn-moiety A or anti-moiety B repeatedly appears in the framework of the above antibiotics. Moreover, these moieties are an important building block for the synthesis of a complex array of methyl and oxygen functional groups involved in these natural products. Efforts have been focused mainly on the development of the regio- and stereocontrolled aldol reaction, and excellent results have been documented. Alternatively, we focused on the synthesis of chiral 2-methyl-3-hydroxy esters [(2R)-syn-I, (2S)-syn-I, (2S)-anti-II and (2R)-anti-II] and 3-methyl-2-hydroxy esters [(2S)-syn-III,
(2R)-syn-III] based on the stereoselective reduction of the corresponding (±)-2-methyl-3-keto ester IV and the stereoselective epoxy ring opening cleavage of trans-epoxybutanoate V with nucleophile (R3), respectively. Optically active 2-methyl-3-hydroxy esters could be obtained by the microbial reduction of the (±)-2-methyl-3-keto ester IV. The above-mentioned enantiomers [(2R)-syn-I and (2S)-syn-I] of 2-methyl-3-hydroxy esters could be obtained based on the lipase-catalyzed resolution of the racemic alcohol (±)-syn-I. Likewise, two coupled enantiomers [(2S)-anti-II and (2R)-anti-II, (2S)-syn-III and (2R)-syn-III] could be obtained by the same strategy from the racemic alcohols [(±)-anti-II, (±)-syn-III] respectively (Scheme 1). In this review, a highly stereoselective syntheses of the natural products such as oudemansins [A (1) and B (2)], (-)-chuangxinmycin (4), (+)-asperlin (5), indolmycins [(6) and (8)], cystothiazole A (9) and myxothiazols [A (10) and Z (11)] were achieved based on a combination of chemical diastereoselectivity and enzymatic enantioselectivity (Scheme 2).
2. SYNTHESES OF OUDEMANSINS6
The strobilurins,1,2 oudemansins [A (1)3, B (2)4, C (3)5] are naturally occurring fungidal substances possessing a β-methoxyacrylate moiety. These compounds are produced by various fungi and bacteria
and have attracted considerable interest from the viewpoint of not only their novel structure but also various kinds of biological activity. For example, they are able to control the growth of fungi, or exhibit insecticidal, antiviral or antitumor activity. Furthermore, they inhibit mitochondrial respiration by binding at a specific site on cytochrome b, and this biological characteristic has made them valuable biochemical tools.
2.1. Synthesis of oudemansin A6
Oudemansin A (1)3, an antibiotic isolated from mycelial cultures of Oudemansiella radicata exhibits strong antifungal activities. The structure and the relative configuration of 1 have been determined by X-ray analysis, but the absolute configuration of its two chiral centers is not known yet. The first synthetic approaches to racemic oudemansin A (1) have been described.7 The absolute structure of 1 was unequivocally determined as 9S, 10S by the synthesis of the optically active form. The most intriguing point of the present synthesis is the preparation of the optically active syn-β-hydroxy esters (12a or 12b). This was successfully achieved by the use of microbiological asymmetric reduction of the corresponding α-methyl-β-keto esters (13a,b). This type of microbiological reduction has been extensively studied by us in a related system.8 Reduction of the β-keto methyl ester (13a) with baker’s
yeast (Saccharomyces cerevisiae) gave the β-hydroxy methyl ester (12a), although the yield was poor (7%). The structure of the reduction product was assigned as 12a (9R, 10S) from the chemical conversion to the authentic sample.9 The reduction product (12a) was then converted to the corresponding (R)-α-methoxy-α-trifluoromethylphenyl acetate ((R)-MTPA ester),10 of which the optical purity was calculated as 95% ee. Then, the reduction of the β-keto ethyl ester (13b) using various yeasts was examined. Among them, Candida albicans was found to afford the syn-β-hydroxy ethyl ester (12b) (35% yield) along with 10% of the corresponding anti-ester and 32% of starting material (13b). These are readily separable by simple chromatography. The structure of the main product was assigned as 12b (9S,10R) and its optical purity (97% ee) was determined in the same way as described in the case of 12a. The remarkable feature of the present reduction is that the absolute configuration of (9S,10R)-12b derived from the ethyl ester (13b) is just opposite to that of (9R,10S)-12a derived from the methyl ester (13a), which shows that the stereochemistry of the reduction is strictly governed by the structure of the substrates and the species of yeasts. The (9S,10R)-β-hydroxy ester (12b) thus obtained was converted to the β-methoxy silyl ether (14) (68% overall yield from (9S,10R)-12b in three steps (a-c). Conversion of 14 to the methoxy ester (15) was achieved by the standard procedure (five steps, d-h) in overall 34% yield. Formylation of 15 with LDA and methyl formate in THF at -78 ˚C to 0 ˚C, followed by treatment with CH2N2-MeOH produced the optically active oudemansin A (1) (26% yield) after purification by HPLC. The spectral data ([α]D, mp and NMR) of the synthetic (-)-1 were identical with those of natural oudemansin A (1).3 The 9S, 10S absolute configuration of natural oudemansin A (1) was thus established. An alternative synthesis of (9S,10R)-17 corresponding to (9S,10R)-12b was achieved by a combination of a chemo-selective method and enzymatic resolution of the racemic β-acetoxy ester (±)-17. Zn(BH4)2-mediated reduction11 of β-keto ester (±)-13a gave predominantly the (±)-syn-β-hydroxy ester (12a), of which acetylation produced the corresponding (±)-acetate (17). This racemic acetate was subjected to the enantioselective hydrolysis using lipase Amano A-6 to provide (9R,10S)-12a (34%) and (9S,10R)-17 (51%) possessing 90% ee and 79% ee, respectively12 (Scheme 3).
2.2. Synthesis of oudemansin B13
Oudemansin B (2) is an antibiotic isolated from mycelial cultures of Xerula mlanotricha and inhibits the growth of a wide variety of saprophytic and phytopathogenic fungi at very low concentration.4 The structure has been deduced by spectroscopic methods and relative structure has been confirmed by the synthesis of (±)-2 by Kallmerten et al.14 However, the absolute configurations of these two chiral centers remain unknown. The absolute structure of 2 was established as 9S, 10S by the total synthesis starting from the chiral intermediate of the known absolute structure. It has been reported that silyl acetylide attacks preferentially the C(3)-position of (±)-trans-(2,3)-epoxybutyrate 18a producing (±)-syn-C(2)-OH, C(3)-Me ester 19,15 from which (±)-2 is expected to be derived by following a similar route already used in oudemansin A (1) synthesis.6 Thus, initially we focused our attention to the synthesis of optically active 18a. We intended to synthesize (2R,3S)-epoxide 18b by a microbial enantioselective reduction of (±)-2-chloro-3-oxobutanoate 20 followed by base-catalyzed epoxide formation.16 The main drawback of this biological method is that along with the desired optically pure anti-compound 21, the isomeric syn-compound 22 is presumed to be produced in almost equal quantity, because we have encountered this difficulty in all biological reductions producing two chiral centers. However, in this particular case, formation of the syn-isomer 22 can not be a serious obstacle, since Mukaiyama et al. have already shown that sodium ethoxide promoted epoxide formation of a mixture of (±)-syn- and anti-2-chloro-3-hydroxy ester affords (±)-trans-epoxide preferentially (cis/trans =
15/85~1/99).17 In fact, reduction of commercially available 20 with baker’s yeast (Saccharomyces cerevisiae) afforded a 1:1 mixture of chlorohydrin 21 and 22 in 68% yield. The mixture was treated with NaOEt giving a mixture of cis and trans epoxy acid (18b) in 84% yield, whose ratio was, from NMR data, found to be 85:15, trans epoxide 18b being predominant as expected. In order to eliminate the contaminated cis-epoxide, the 85:15 mixture was converted to brucine salt. On one recrystallization of the crude salt followed by base treatment, the isomer-free, optically pure (>99% ee) (2R,3S)-epoxy carboxylic acid was obtained. This acid was treated with CH2N2 to give the desired pure 18a in overall 37% yield from a mixture of 21 and 22. The absolute structure of (2R,3S)-18a was confirmed by the conversion to the known (2R,3S)-indolmycenic methyl ester.18 By applying Roush’s method,15 18a was then converted to the acetylene (19) in 60% yield. Partial hydrogenation of 19 using Lindlar catalyst followed by consecutive methylation, hydroboration with disiamylborane in THF and silylation gave silyl ether (23) in 30% overall yield from 19. DIBAL reduction of 23 afforded aldehyde 24, which was allowed to react with phosphonium salt (25) in the presence of n-BuLi giving a 1:1 mixture of the condensation products (cis-26 and trans-26) in 70% yield from 23. After desilylation with the fluoride ion (Bu4N+F-·3H2O), the mixture was separated by silica-gel column chromatography to cis-alcohol (45.2%) and trans-alcohol (35.3%). The trans-syn-alcohol was oxidized with CrO3 and the resulting acid was esterified with CH2N2 giving methyl ester (27) in 75% yield. Formylation of 27 with methyl formate in the presence of LDA in THF at -78 ˚ (then to 0 ˚C) followed by CH2N2-MeOH treatment afforded (-)-oudemansin B (2) in 31% yield after purification by HPLC. The physical data (CD, [α]D, MS, NMR and UV of the synthetic (-)-2 were identical with those of natural oudemansin B (2).4 The absolute configuration of natural oudemansin B (2) was thus established as shown in 2. Chemoenzymatic synthesis of oudemansin X (3)19, 20 and formal syntheses of oudemansins A (1), B (2), X (3)21,22 were also achieved (Scheme 4).
3. SYNTHESIS OF (-)-AND (+)-CHUANGXINMYCIN23, 24, 25
Chuangxinmycin (4), isolated from Actinoplanes tsinanensis. sp. in China, exhibits in vitro an antibacterial spectrum that includes a number of Gram-positive and Gram-negative bacteria. This material was reported to be active in mice against Escherichia coli and Shigella dysenteria infections in vivo, and effective in the treatment of septicaemia, urinary, and biliary infections caused by E. coli in preliminary clinical results.26 The relative structure of 4 was confirmed by synthesis26 and the absolute configurations were determined as 4S, 5R based on the degradation study of the natural product (4)26 and
optical resolution of (±)-4 with S-(-)-α-phenylethyl amine.26 Synthetic attempts were made using two published routes. One is an internal Knoevenagel condensation of 4-substituted-3-acetyl indole 29 and the subsequent reduction of 30 gave a mixture of (±)-cis-methyl ester 28 of 4 and trans-28 via pathway B.26 In the other route, treatment of 31 with the fluoride ion liberated the indol-1-yl anion by desilylation, and Michael addition of the ambident C-3 anion to the powerful α-thioacrylate acceptor could bring about the required cyclization via pathway C.26 (Scheme 5) But these routes were found to be unacceptable for the synthesis of the desirable optically active form of 4. Stereoselective synthesis of (-)-4 via pathway A was directed toward chiral synthesis starting with the requisite (2R,3S)-syn-mercapto ester (32) possessing two definite absolute configurations at the C(2)- and C(3)-positions. The synthesis of (2R,3S)-32 could be performed from (2R,3S)-4’-iodoindolmycenate (33) along with retention at C(2) stereochemistry in 33. The synthesis of indolmycenate, being an important intermediate for the synthesis of indolmycin,18 was achieved by the reaction of indole and (±)-trans-(2,3)-epoxy butanoate 18a in the presence of SnCl4 along with nucleophilic displacement with inversion at the C(3) carbon of the coordinated epoxide.18 This strategy appeared to be the most promising from a stereochemical standpoint for the stereoselective construction of C(2)- and C(3)-configurations of 33 by the reaction of 4-iodoindole 3426 and (2R,3S)-18a in the presence of SnCl4 (Scheme 5).
3.1. Synthesis of (2R,3S)- and (2S,3R)-epoxybutanoates18, 24, 25
The synthesis of (2R,3S)-18a based on the microbial reduction of α-chloroacetoacetate was already described in 2.2. In this section, the synthesis of both enantiomers of the (2,3)-anti-2-hydroxy- 3-substituted butanoate congener based on the lipase-catalyzed enantioselective hydrolysis and the synthesis of (2R,3S)- and (2S,3R)-18a were described as shown in Scheme 6. The reaction of (±)-trans-(2,3)-epoxy butanoate 18a derived from methyl crotonate with the chloride ion, benzyl alcohol and p-methoxyphenol in the presence of Lewis acid gave (2,3)-anti-2-hydroxy- 3-substituted butanoate ((±)-35a (39%), (±)-35b (27%), (±)-35c (46%)), respectively, which were subjected to acetylation to afford the corresponding acetates ((±)-36a,b,c), respectively18 (Scheme 6). Initially, three kinds of (±)-acetates (36a,b,c) were subjected to screening experiments using several kinds of commercially available lipases in phosphate buffer (pH 7.25) or water-saturated isopropyl ether. The results are shown in Table 1. When the substrate (±)-36a was subjected to enzymatic hydrolysis using lipase "Amano P" from Pseudomonas sp. in water-saturated isopropyl ether, the (2R,3S)-35a (40%, 89% ee) and the unchanged (2S,3R)-36a (45%, 87% ee) were obtained (entry 4, Table 1). The E-value27 of this resolution was estimated to be 16.2. The (2S,3R)-36a having 87% ee was again subjected to enzymatic hydrolysis to afford the enantiomerically pure (2S,3R)-36a in 84% yield (entry 5, Table 1). On the contrary, the 89% enantiomeric excess of (2R,3S)-36a was subjected to enzymatic hydrolysis to provide
the enantiomerically pure (2R,3S)-35a in 68% yield (entry 6, Table 1). The enantiomeric excess of (2S,3R)-36a and (2R,3S)-35a was calculated based on NMR (400 MHz) data of the corresponding (R)-MTPA ester. The thus-obtained (2S,3R)-36a was treated with NaOMe followed by acid treatment and esterification with CH2N2 to provide the enantiomerically pure (2R,3S)-epoxy butanoate (18a) in 67% overall yield. Likewise, the enantiomerically pure (2R,3S)-35a was also converted to (2S,3R)-18a in 69% overall yield.
3.2. Synthesis of (-)- and (+)-chuangxinmycins24, 25
The reaction of 4-iodoindole (34) and (2R,3S)-18a in the presence of SnCl4 afforded (2R,3S)-33 (32% yield) along with a mixture of (2S,3R)-35a and 4’-iodoindole dimer. Treatment of (2R,3S)-33 with MsCl in pyridine followed by treatment with CsSAc provided (2R,3S)-2-thioacetoxy ester (38) in 73%
overall yield with complete retention of C(2)-stereochemistry. Deacetylation of (2R,3S)-38 with K2CO3 in MeOH provided (2R,3S)-2-mercapto ester 32 (70% yield), which was subjected to Pd(PPh3)4-mediated cyclization in the presence of Et3N to give the (4S,5R)-cis-methyl ester (28) in 74% yield. An alkaline hydrolysis of (4S,5R)-28 was carried out by the reported procedure26 to provide the natural chuangxinmycin (4S,5R)-4, which is consistent with the reported (4S,5R)-4. Likewise, the enantiomerically pure (2S,3R)-18a was converted to the (4R,5S)-4 via (2S,3R)-33, (2S,3R)-38, (2S,3R)-32 and (4R,5S)-28. The physical data of the present (4R,5S)-4 was identical with those of the reported (4R,5S)-4.26 As shown in Scheme 7, it is proposed that neighboring group participation involving the electron-rich C(3) of the indole ring accounts for the stereoselective conversion of (2R,3S)-37 to (2R,3S)-38. The preferred conformation of the mesylate 37 derived from (2R,3S)-33, in which steric interactions are minimized, is shown in 37. In this rotamer, the mesyloxy group is trans to the indol C(3), so that ready displacement can occur. Nucleophilic attack by the thioacetoxy ion takes place at the C(2)-position because the charge in the cyclopropylium ion intermediate is still predominantly centered on the C(2) carbon atom. Since this is essentially a double SN2 mechanism, the syn-stereochemistry of the (2R,3S)-syn-2-hydroxy ester (33) is retained in the (2R,3S)-syn-2-thioacetoxy ester (38) (Scheme 7).
4. SYNTHESIS OF (+)-ASPERLIN28
(+)-Asperlin (5), isolated from Aspergillus nidulans and Aspergillus caespiyosus, has been shown to exhibit antitumour and antibacterial activity. Its structure, including the absolute configuration, was determined by spectroscopic and chemical studies.29-31 Because of its interesting bioactivity, the synthesis of natural product (5) and its related compounds has been reported by several groups.32 The syntheses of (+)-5 from natural products such as L-rhamnose,33 (S,S)-tartaric acid,34 and D-glucose35 has been reported. Recently, the convenient syntheses of (+)-5 based on the Sharpless asymmetric epoxidation of unsymmetrical divinylmethnol congeners have been reported.36 On the other hand, we reported the lipase-catalyzeded resolution of racemic α-acetoxy ester (±)-36a, a key intermediate in the synthesis of (+)-asperlin, to give the optically pure (2R,3S)-35a (>99% ee) and (2S,3R)-36a (>99% ee) as shown in Table 1. The two concise syntheses of (+)-asperlin (5) based on the stereoselective addition of carbon-nucleophile to the chiral α-silyloxy aldehyde, (2R,3S)-3-chloro-2-tert-butyldimethyl-silyloxy-butanal (41), derived from (2R,3S)-35a was shown in Schemes 8 and 10.
4.1. Formal synthesis of (+)-asperlin (5)
The formal synthesis of (+)-asperlin (5) from (2R,3S)-35a is shown in Scheme 8. Silylation of (2R,3S)-35a with tert-butyldimethylsilyl chloride (TBDMSCl) gave the corresponding silyl ether (2R,3S)-39 (92%), which was reduced with diisobutylaluminum hydride (Dibal-H) to afford an alcohol (2R,3S)-40 in 88% yield. Pyridinium chlorochromate (PCC) oxidation of (2R,3S)-40 gave the desired aldehyde (2R,3S)-41 (69%), which was reacted with α-furyl anion to afford the (1S,2R,3S)-secondary alcohol (42) stereoselectively in 72% yield. To confirm the stereochemistry of (1S,2R,3S)-42, it was converted to the known chiral intermediate, epoxy-alcohol (-)-(1S,2R,3R)-43,36a,b for the synthesis of (+)-5. Protection of the secondary alcohol group of (1S,2R,3S)-42 as tetrahydropyranyl (THP) group
followed by consecutive desilylation and K2CO3 treatment gave an epoxide, which was subjected to deprotection of the THP group to provide the desired epoxy-alcohol (43) in 83% overall yield from 42. Spectral data (1H- and 13C-NMR) of the synthetic 43 were identical with those of the reported (-)-(1S,2R,3R)-43.36a The synthesis of (+)-5 from (-)-(1S,2R,3R)-43 was already achieved by Honda et al.36a The stereoselective formation of (-)-(1S,2R,3S)-42 from (2R,3S)-41 could be explained by Felkin-Anh model as shown in Figure 1.
4.2. Concise synthesis of (+)-asperlin (5)
A concise synthesis of (+)-asperlin (5) from (2R,3S)-41 based on a combination of the indium (In)-assisted stereoselective addition of 3-bromopropenyl acetate (44)37 to the α-silyloxy aldehyde, (2R,3S)-41 and ring closing metathesis (RCM) using Grubbs catalyst38 is shown in Scheme 10. Recently, M. Lombardo et al. reported that α-hydroxyallylation reaction of Garner aldehyde (45) using 44 in the presence of in gave predominantly a C(4)-C(1’)-anti-C(1’)-C(2’)-anti-diol (47) via 46 as shown in Scheme 9.39 This strategy could be promising for the construction of the four contiguous chiral centers in asperlin (5) (Schemes 9 and 10).
The reaction of (2R,3S)-41 with 3-bromopropenyl acetate (44) in the presence of In gave predominantly alcohol 48 in 75% yield, which was converted to the epoxyalcohol 49. Protection of the secondary alcohol group of 48 as a THP group followed by consecutive desilylation and K2CO3 treatment gave an epoxide, which was subjected to deprotection of the THP group to provide the desired epoxyalcohol (49) in 83% overall yield from 48. Treatment of 49 and acryloyl chloride gave the corresponding acrylate (50) in 71% yield, which was subjected to RCM reaction using Grubbs catalyst 2nd generation40 to afford (-)-epi-asperlin (51) in 49% yield. Spectral data (1H- and 13C-NMR) of the synthetic (-)-51 were identical with those of the reported (-)-51.32b Based on the conversion of 48 to (-)-51, the
stereochemistry of 48 was determined to be 3R, 4R, 5R, 6S-configurations. When hydrolysis of acetyl group in (-)-51 was carried out using K2CO3 in MeOH, the desired product (52) was not obtained. The lipase PL (from Alcaligenesis sp.)-catalyzed hydrolysis of (-)-51 in H2O saturated (i-Pr)2O gave the desired alcohol (52, 50%) along with the starting material (-)-51 (32% recovery). Finally, the alcohol 52 was treated with AcOH in the presence of triphenylphosphine (Ph3P) and diethyl azodicarboxylate (DEAD) to provide (+)-asperlin (5) in 72% yield. Spectral data (1H- and 13C-NMR) of the synthetic (+)-5 were identical with those of the reported (+)-5.36a Stereoselective formation of 48 from (2R,3S)-41 could be explained by insights reviewed by Lombardo et al.41 Among four possible twist-boat transition states (TSs), TS-A, -B, -C and –D as shown in Figure 2, TS-C (or TS-D) might be favored than TS-A (or
TS-B) because steric repulsion between the aldehyde substituent (R) and acetoxyl group appears to be small. This insight might imply the formation of C(3)-C(4)-anti-48. On the other hand, the C(4)-C(5)-anti-stereoselection of 48 could be explained by Paquette et al.42 who showed that 1,2-addition of the allylindium reagents to α-oxygenated aldehydes gave the non-chelation-controlled product, which is corresponding to the 1,2-anti product by the Felkin-Anh model (Figure 2).
5. SYNTHESES OF INDOLMYCINS (6 AND 8)43
(-)-Indolmycin (6), an antibiotic isolated from an African strain of Streptomyces albus, exhibits an antibacterial activity against Staphylococci.44 Indolmycin congeners 7 and 8, which possess a hydroxyl or methoxyl group in the 5’-position of the indole skeleton, have been obtained by the addition of indole precursors such as 5-hydroxy- and 5-methoxyindoles to growing cultures of Streptomyces griseus ATCC 12648.45 These congeners show a moderate increase in antimicrobial activity compared to 6. In a recent study, 6 was reported to exhibit potent antibacterial activity against Helicobactor pylori (H. pylori), and is thus a promising anti-H. pylori agent46 (Scheme 11). Racemic syntheses of 6 have been developed by two groups.47 The first asymmetric synthesis of (-)-6 was achieved based on the reaction of (2R,3S)-6-alkyliden-3,4-dimethyl-2-phenylperhydro-1,4-oxaazepine-5,7-dione with Grignard reagent.48
Syntheses of optically active indolmycin (6) have been carried out using resolution method49 or based on the reaction of indolyl magnesium bromide with (2S,3R)-epoxy butanoate, affording (2S,3R)-indolmycenate derivative (A).50 Palladium-catalyzed heteroannulation of internal alkynes (C) using
o-iodoaniline or its derivatives (B) has been reported to give indole derivatives (E) via a vinylpalladium intermediate (D) as shown in Scheme 12,51 and this procedure (the Larock indole synthesis) seems to be promising for synthesis of optically active indolmycenate derivatives (A). The concise synthesis of (-)-indolmycin (6) and (-)-5-methoxyindolmycin (8) based on a palladium-catalyzed reaction of o-iodoaniline congeners and (2S,3R)-2-hydroxy-3-methyl-5-trimethylsilyl-4-pentynoate (53) or its acetate (54) was shown in Scheme 13. The optically active (2S,3R)-53 or (2R,3S)-53 could be obtained based on the enantioselective acetylation of (±)-53 using lipase as shown in Table 2.
5.1. Lipase-catalyzed enantioselective acetylation of (±)-53
Using a previously reported procedure,15 substrate (±)-53 was obtained by reaction of (±)-trans-(2,3)-epoxy butanoate (18) (Scheme 6) and trimethylsilylacetylide. For the purpose of determining the enantiomeric excess (ee) of the enzymatic reaction products, racemate (±)-53 was converted to two benzoates (±)-55 and (±)-56, which gave two well separated peaks of enantiomeric isomers, respectively, in HPLC analysis, thus allowing determination of the ee of the enzymatic reaction products. A screening experiment for finding a suitable enzyme showed that the most effective lipase was found to be Amano P or Amano PS from Pseudomonas sp. When (±)-53 was subjected to enantioselective acetylation using “Amano P” in the presence of isopropenyl acetate as an acylating reagent for 7 days, acetate 54 (28%, 82% ee) and unreacted alcohol 53 (59%, 44% ee) were obtained (Table 2, entry 1). The 44% ee of unreacted alcohol 53 was subjected again to enzymatic acetylation using Amano PS in the presence of vinyl acetate for 3 days, giving acetate 54 (23%, 86% ee) and unreacted alcohol 53 (60%, 95% ee) (Table 2, entry 2). Enzymatic acetylation of (±)-53 using Amano PS in the presence of vinyl acetate for 5 days gave acetate (+)-54 (48%, 95% ee) and unreacted alcohol (-)-53 (50%, 94% ee) (Table 2, entry 3). The enantiomeric excess (ee) for each enzymatic product was calculated by HPLC analysis after conversion of the enzymatic product to the corresponding benzoate. The E-value of this enzymatic reaction (entry 3) was estimated to be 139. The absolute structure of the enzymatic reaction product (-)-53 was determined by direct comparison with previously reported sample52 (2R,3S)-53 (>99% ee), and thence the absolute configurations of acetate (54) were to be 2S, 3R (Table 2).
5.2. Synthesis of (-)-indolmycin (6) and (-)-5-methoxyindolmycin (8)
Syntheses of (-)-indolmycin (6) and (-)-5-methoxyindolmycin (8) from (2S,3R)-54 are shown in Scheme 13. At first, the reaction of o-iodoaniline and (±)-53 in the presence of [1,1-bis(diphenylphosphono)ferrocene]palladium(II) dichloride dichloromethane complex, LiCl, and Na2CO3 in DMF followed by desilylation did not give the desired indolmycenate (±)-61 (Scheme 13). Then, two types of o-iodoaniline congeners, N-(tert-butoxycarbonyl)-2-iodoaniline (57) (Scheme 14) and N-(tert-Butoxycarbonyl)-2-iodo-4-methoxyaniline (58) (Scheme 14), were synthesized by N-tert-butoxycarbonylation of o-iodoaniline and the known 2-iodo-4-methoxyaniline,53 respectively. Reaction of (2S,3R)-54 and 57 using Pd(OAc)2 in the presence of Ph3P, Et4N+Cl and i-Pr2NEt gave the indole congener 59 in 85% yield, this was treated with CF3COOH to afford 60 in 90% yield. Alcoholysis of 60 with K2CO3 in MeOH gave (+)-indolmycenate (61, 87% yield), whose physical data were consistent with those of the reported (+)-61,18,54 including the sign of the specific rotation. The reaction of (+)-61 with guanidine hydrochloride in the presence of tert-BuOK using a previously reported procedure50 gave 2-amino-4(5H)-oxazolone congener 62, which was reacted with 40% methylamine to afford (-)-indolmycin (6, 68% yield from 61). The physical data of synthetic (-)-6 were consistent with those of previously reported indolmycin 6.48 From NMR study of synthetic (-)-6, (-)-6 was found to be a 2:1 mixture of two tautomers 6 and its tautomer. The main tautomer was the desired (-)-6. Isolation of two tautomers was found to be difficult and this tendency was found in the previous case50 Similarly, the reaction of
(2S,3R)-54 and 58 using Pd(OAc)2 in the presence of Ph3P, Et4N+Cl and i-Pr2NEt gave the indole congener 63 (88% yield), which was treated with CF3COOH to afford 64 in 64% yield. Alcoholysis of 64 with K2CO3 in MeOH gave (-)-5-methoxyindolmycenate (65) in 99% yield. The reaction of (-)-65 with guanidine hydrochloride in the presence of tert-BuOK provided 2-amino-4(5H)-oxazolone congener (66), which was reacted with 40% methylamine to afford (-)-5-methoxyindolmycin (8, 88% yield from 65). From NMR study of synthetic (-)-8, (-)-8 was also found to be a 2:1 mixture of two tautomers 8 and its tautomer.
The formation of indole congener (59 and 63) by a palladium(II)-catalyzed reaction of internal alkyne (2S,3R)-54 with o-iodoaniline derivative 57 and 58, respectively, and the corresponding catalytic cycle may be explained as shown in Scheme 14. First, palladium (II) is converted to palladium (0) under the specified reaction conditions. Oxidative addition of o-iodoaniline congener 57 or 58 to Pd(0) gives intermediate F, which undergoes syn insertion of internal alkyne (2S,3R)-54 into the arylpalladium bond to afford intermediate G. Next, nitrogen displacement of the palladium in the resulting vinylpalladium intermediate G results in the formation of a six-membered-ring intermediate H along with elimination of hydrogen iodide (HI); finally, reductive elimination of palladium from intermediate H gives indole congener 59 or 63 and Pd(0). Annulation of unsymmertrical alkynes has proved to be highly regioselective, providing only the appropriate regioisomer. The more sterically bulky group is located nearer the nitrogen atom in the indole product51 (Scheme 14).
6. SYNTHESES OF CYSTOTHIAZOLES AND MELITHIAZOLS
In 1998, Sakagami and co-workers reported the isolation of six secondary metabolites, named cystothiazoles A (67)-G (72) from a culture broth of the myxobacterium, Cystobacter fuscus.55 On the other hand, in 1999, G. Höfle and co-workers reported the isolation of thirteen new β-methoxyacrylate (MOA) fungicides, melithiazols A-N related to myxothiazol A (10)56 from cultures of Melittangium lichenicola, Archangium gephyra and Myxococcus stipitatus.57 They are related to the oudemansins A(1),3 B(2),4 X(3),5 which are naturally occurring congeners of β-methoxyacrylic acid. Myxothiazol A (10), cystothiazoles and melithiazols possess a bis-thiazole skeleton as well as a β-methoxyacrylate moiety. Cystothiazoles have indicated potent antifungal activity against the phytopathogenic fungus, Phytophthora capsici (0.05-5 µg/disk), and have shown activity against a broad range of additional fungi with no effect on bacterial growth. Furthermore, cystothiazole A (67) was examined for the in vitro cytotoxicity using human colon carcinoma HCT-116 and human leukaemia K562 cells. The IC50 values of cystothiazole A (67) were 110~130 ng/ml, which were significantly higher than those of myxothiazol A. The fungicidal activity of these β-methoxyacrylate (MOA) inhibitors has been shown to be due to their ability to inhibit mitochondrial respiration by blocking electron transfer between cytochrome b and cytochrome c. The absolute structure of cystothiazole A (67) was established by a combination of spectroscopic analysis and chemical degradation of the natural product. The structures of melithiazols
were established on the basis of spectroscopic data, and confirmed in the case of melithiazol E (74), including its relative configuration, by an X-ray structure analysis. The absolute configuration of melithiazols A and B (73) was determined by degradation and CD spectroscopy. Biological activities including antifungal and cytotoxic activities, inhibition of NADH oxidation, etc., were also examined (Scheme 15).
6.1. Retrosynthesis of cystothiazole and melithiazols58
Total synthesis of a diastereomeric mixture of myxothiazol A (10) has been achieved,59 but chiral synthesis of the left-half part possessing two chiral centers has not been carried out. Another racemic synthesis of the left-half part of 10 starting from benzyloxyacetaldehyde is also reported.60 The first enantiocontrolled synthesis of cystothiazole A (67) was achieved based on the preparation of a bis-thiazole core for the application of the asymmetric Evans aldol methodology for the development of the C(4)/C(5) vicinal stereochemistry.61 Semisynthesis of melithiazols B (73) and M (79) was achieved based on the chemical conversion from myxothiazol A (10) possessing (4R,5S,14S)-absolute configurations.62 After our synthesis of cystothiazole A (67) and B (68) was reported, syntheses of cystothiazole A (67),63-67 B (68),65 C (69),61,63 and E (112, see Scheme 20)68 were reported. Our retrosynthetic strategy of cystothiazoles and melithiazols is illustrated in Scheme 16 (Scheme 16). Retrosynthetically, the synthesis of cystothiazoles and melithiazols can be achieved by Wittig condensation of the left-half aldehyde A (=81) and the right-half phosphonium iodide B. The aldehyde (81) can be derived from the tetrahydro-2-furylidene acetate (82), which can be obtained by oxidative cyclization-methoxycarbonylation of (2R,3S)-3-methylpenta-4-yn-1,2-diol (83) in the presence of Pd(II) /p-benzoquinone in MeOH under a carbon monoxide atmosphere. The synthesis of the chiral diol 83 can be achieved by the reaction of (2R,3S)-epoxy butanoate (18a)16,18,25 and silyl-acetylide followed by reduction. An important chiral synthon (2R,3S)-18a has been synthesized based on the lipase-catalysed asymmetric hydrolysis of (±)-(2,3)-trans-3-acetoxy-2-chlor-butanoate (36a) as described in 3.1.18,25 A catalytic conversion of 83 to 82 is a key step in this total synthesis, and this type reaction was previously reported as shown in Scheme 16.69 The oxidative cyclization-methoxy-carbonylation of acyclic-4-yne-1-ols E affords (E)-cyclic-β-alkoxyacrylates F in good to excellent yields.69 In this reaction, p-benzoquinone is found to be a very efficient reagent for trapping a proton of hydrogen chloride arising from the catalytic cycle and oxidative transfer of the generated Pd(0) species to a Pd(II) species. The synthesis of right-half phosphonium salt B could be achieved from an amide D as shown in Scheme 16. The thioamide derived from amide D was first subjected to Hantzsch reaction with ethyl bromopyruvate leading to the thiazole ester intermediate C. Following manipulation of the ester functionality in C to the corresponding thioamide, a second Hantzsch reaction with ethyl bromopyruvate then gave the bis-thiazole ester, which could be converted to the corresponding phosphonium salt B.
6.2. Synthesis of left-half aldehyde (81)58
As we previously described the synthesis of oudemansin B (2) in 2.2, the reaction of (2R,3S)-epoxy butanoate (18a) and lithium silyl-acetylide in the presence of Et2AlCl followed by consecutive desilylation and reduction gave (-)-83 in 76% overall yield. The oxidative cyclization- methoxycarbonylation of (-)-83 in the presence of PdCl2 (5 mol %)/p-benzoquinone (1.1 equiv.) in MeOH at 0 °C under a carbon monoxide atmosphere (balloon) gave a crude secondary alcohol (82), which was immediately subjected to methylation using MeI in the presence of Ag2O to afford the methoxy compound (-)-84 in 51% overall yield. Acid treatment of (-)-84 provided a hemiketal 86 (68%) along with the isomerized product (+)-85 (5%). The geometry of (+)-85 was confirmed to be Z-form because of n.O.e. enhancement for the olefinic proton and methine proton (8%); thence, that of (-)-84 was deduced to be E-form. Silylation of 86 in DMF at 80 °C gave a silyl ether (-)-87 in 84% yield, which was subjected to consecutive methylation (Me2SO4/K2CO3) and desilylation (Et3N(HF)3) to afford an alcohol (+)-88 in 50% overall yield. The (E)-geometry of (+)-88 was confirmed by the n.O.e. enhancement for the olefinic proton and the methoxyl group (6%). Dess-Martin periodinane oxidation of (+)-88 afforded the desired aldehyde (+)-81 in 89% yield, whose NMR spectra were identical with those of the reported (±)-81.60 As the reaction mechanism, the following path is plausible based on the
precedents (Scheme17). Coordination of the triple bond of the substrate (-)-83 to the Pd(II) species
enables the inner primary hydroxyl group to attack the unsaturated bond, giving rise to σ-Pd complex H. It is well documented that the C-Pd bond undergoes CO insertion to generate intermediate I, which is reacted with MeOH to provide the product 82. It is a well-established feature of the reactions initiated by intramolecular nucleophilic attack on the triple bond coordinated to Pd(II) that the attack is anti with respect to palladium. The exclusive occurrence of E stereochemistry in product 82 is clearly in agreement with this mechanistic hypothesis (Scheme 17).
6.3. Syntheses of cystothiazoles A (67),58 B (68)70 and melithiazol B (73) 71
Antifungal substances named cystothiazoles A (67) and B (68) were isolated from the myxobacterium Cystobacter fuscus strain AJ-13278 by using an inhibition assay against the phytopathogenic fungus Phytophthora capsici. 55 The structure of cystothiazole B (68) was deduced based on similar 1H-NMR data (H-4 and H-5) and specific rotations of cystothiazole A (67). Melithiazols B (73) and M (79) have been isolated from myxobacterium Archangium gephyra, strain Ar 7747, and exhibit antifungal and cytotoxic activity, inhibition of NADH oxidation. The structures of B (73) and M (79) were established on the basis of spectroscopic analysis and the absolute configuration of B (73) was determined by CD spectroscopy.57 Semisynthesis of B (73) and M (79) was achieved based on the chemical conversion from myxothiazol A (10) possessing (4R,5S,14S)-absolute configuration.62 The synthesis of three phosphonium iodides (B=89, 90 and 91) was achieved as shown in Scheme 18.
Treatment of isopropylamide (92) with P4S10 gave an isopropylthioamide (93), which was reacted with bromopyruvate to afford a mono-thiazole ester (94) in 82% overall yield from 92. Treatment of 94 with NH3 / MeOH followed by thioamidation with Lawesson’s reagent yielded a thioamide (96), which was reacted with bromopyruvate to afford a bithiazole ester (97) in 81% overall yield from 94. LiBH4 reduction of 97 followed by treatment with I2/Ph3P/imidazole provided an iodide (99) in 70% overall yield from 97. The reaction of 99 and triphenylphosphine gave a phosphonium salt (89) in 80% yield. Treatment of 97 with N-bromosuccinimide (NBS) in the presence of 2,2’-azobisisobutyronitrile (AIBN) gave a bromo compound, which was treated with saturated NaHCO3 aqueous solution to give an alcohol (100) in 91% overall yield from 97. Diisobutylaluminium hydride (Dibal-H) reduction of 100 followed by treatment with I2/Ph3P/imidazole provided an iodide (102) in 76% overall yield. The reaction of 102 and triphenylphosphine gave a phosphonium salt (90) in 90% yield. Treatment of alcohol (100) with p-TsOH gave an olefin (103) in 88% yield, which was reacted with Dibal-H to afford a primary alcohol (104) in 65% yield. Treatment of 104 with I2/Ph3P/imidazole provided an iodide (105) in 86% yield. The reaction of 105 with triphenylphosphine gave a phosphonium salt (91) in 98% yield.
Then, Wittig olefination of the aldehyde (81) with phosphonium salts (89, 90 and 91) was shown in Scheme 18. The reaction of 81 and 89 in the presence of lithium bis(trimethylsilyl)amide (LHMDS) in THF gave a mixture (E/Z = 4/1) of cystothiazole A (67) and (Z)-cystothiazole A in 82% yield. Both isomers were isolated by preparative silica-gel thin-layer chromatography to provide (+)-67 and (Z)-isomer. The reaction of 81 and 90 in the presence of LHMDS in THF gave a mixture (E/Z = 3/1) of cystothiazole B (68) and (Z)-cystothiazole B in 69% yield. The condensation reaction conditions were not optimized at this stage. This mixture was subjected to preparative thin-layer chromatography (silica gel) to provide a colorless oil (+)-68 and a colorless oil (Z)-isomer. The physical data of the synthetic (+)-68 were identical with those of the natural cystothiazole B (+)-68.55 The stereochemistry of cystothiazole B (68) was proved to be (4R, 5S, 6(E)). The reaction of 81 and 91 in the presence of LHMDS in THF gave a 3:1 ((E) / (Z)) mixture of olefins (73) in 39% yield. A part of this mixture was subjected to purification by means of preparative silica-gel thin-layer chromatography (CH2Cl2) to provide (+)-73 as a colorless oil. The condensation yield was found to be unsatisfactory, but reaction conditions were not optimized at this time. The physical data of the synthetic (+)-73 were identical with those of the reported melithiazol B (73).56 Moreover, the physical data of the synthetic (+)-73 were identical with those of melithiazol B (73) from the myxobacterium Cystobacter fuscus strain AJ-13278.71
6.4. Syntheses of cystothiazole G (72)72 and melithiazols F (75),73 I (78),73 M (79)71 and C (111)74
Relative structures of cystothiazole G (72) and melithiazols F (75), I (78), M (79) and C (111) were reported although the absolute structures of these natural products were not yet. For the purpose of determination of the absolute structures of these natural products, the reactions of (+)-81 and phosphonium salts (106, 107 and 108) in the presence of LHMDS was carried out to give cystothiazole G (72) and melithiazols F (75), I (78), respectively. Moreover, the reactions of (+)-81 and phosphonium salt (110), and sulfone (109) in the presence of LHMDS afforded two condensation products, which were converted to melithiazols C (111) and M (79), respectively. The spectral data (1H- and 13C-NMR) of the synthetic compounds (72, 75, 78, 79 and 111) were identical to those of the natural products (72, 75, 78, 79 and 111) including the sign of specific rotation, respectively, and the absolute structures of these natural products were determined as shown in Scheme 19.
7. SYNTHESIS OF CYSTOTHIAZOLE E (112)74
Cystothiazole E (112)55 was isolated from Cystobacter fuscus strain AJ-13278. The absolute structure of cystothiazole E (112) was confirmed by the synthesis of antipodal 112.68 Retrosynthetically, the synthesis of 112 can be achieved by the double bond formation between the left-half aldehyde A and the right-half sulfone (113) as shown in Scheme 20. By applying the previously reported procedure,58 the reaction of (2R,3S)-epoxy butanoate 18a and lithium silyl-acetylide in the presence of Et2AlCl gave 53 in 71% yield. Desilylation of 53 followed by hydrolysis gave an acetylenic α-hydroxy-carboxylic acid 115, which was treated with 5 mol % of Pd(MeCN)2Cl2 and Et3N in MeOH to give methyl ketone (116) in 58% yield from 115. Methylation of 116 gave α-methoxy ester 117 (73%), which was subjected reduction to afford a diastereomeric mixture of diol (118) in 73% yield. Selective protection of primary
hydroxyl group in 118 followed by silylation gave a diastereomeric mixture of 120 in 96% yield. Reductive deprotection of pivaloyl group in 120 gave a diastereomeric mixture of 121 in 98% yield, which was subjected to the Dess-Martin oxidation to afford a diastereomeric mixture of aldehyde (122 =A) in 84% yield. The sulfone (113) corresponding left-half was obtained by treatment of alcohol (98) (see Scheme 18) with 2-mercaptobenzothiazole (BTSH)/Ph3P/diethylazodicarboxylate (DEAD) followed by oxidation with 30% H2O2 in the presence of Mo7O24(NH4)6·4H2O in 77% yield. The Julia’s coupling75 of the sulfone(113) and aldehyde (122) in the presence of LHMDS in THF to afford a mixture of olefins in 96% yield.
Deprotection of silyl group of the olefinic mixture gave the less polar alcohol (35%) and the more polar alcohol (65%). The geometry of the less polar alcohol was found to be Z-form by the coupling constant (J=12.0 Hz) due to olefinic proton and thence that of the more polar alcohol was deduced to be (E)-form. Dess-Martin oxidation of (E)-alcohol afforded methyl ketones (E)-112 (80%). The specific rotation and NMR data of the synthetic (E)-112 were identical with those of natural cystothiazole E (2).55b
8. IMPROVED SYNTHESIS OF CYSTOTHIAZOLE A (67) 52
In this section, a new chiral synthesis of cystothiazole A (67) for the purpose of improvement of the overall yield and the (E)-selectivity of the C(6)/C(7) double bond was carried out as shown in Scheme 21. Methylation of 53 (Scheme 20) followed by consecutive reduction and silylation afforded the silyl ether 125 possessing the terminal acetylene group in 52% overall yield from 53. By applying Tsuji’s procedure,76 the acetylenic compound 125 was converted to acetylenecarboxylate 126 in 87% yield under atmospheric pressure (balloon) of carbon monoxide at room temperature using 5 mol % of PdCl2 and a stoichiomeric amount of CuCl2 in MeOH. By applying the reported procedures,77 conjugate addition of MeOH to acetylenecarboxylate 126 in the presence of a catalytic amount of Bu3P afforded the corresponding (Z)-β-methoxy-α,β-unsaturated ester 127 in 86% yield. The (Z)-geometry of 127 was confirmed by the n.O.e. enhancement for the olefinic proton and the methine proton (8.6%). The formation of (Z)-127 from 126 could be explained as shown in Scheme 22. Addition of tributyl phosphine to 126 followed by MeOH attack would give intermediates TS-A and TS-B. Intermediate TS-B could lead to (Z)-127 because TS-B is more stable than TS-A due to stereochemical repulsion in TS-A. Isomerization of (Z)-127 to (E)-127 was carried out by the following procedure. When a solution of
(Z)-127 in CDCl3 (chloroform-d + 1% v/v TMS (D, 99.8%) + SILVER FOIL) from Cambridge IsotopeLaboratories, Inc.) was stood for 3 d at room temperature, (E)-127 was exclusively obtained. The crude (E)-127 was treated with Et3N·(HF)3 in CH2Cl2 to give the primary alcohol (+)-88 in 89% overall yield from (Z)-127, which was identical with the reported (+)-88.58b Another CDCl3 (99.8% atom % D from Aldrich) was also effective for this selective isomerization. On the other hand, the distillated CHCl3, CH2Cl2 (CF3SO3)Yb/CH2Cl2, and silica gel/MeOH were inactive for this selective isomerization. Treatment of (Z)-127 with pyridinium p-toluenesulfonate (PPTS)/MeOH/PhH, or (CF3SO3)Sc/CH2Cl2 or InCl3/CH2Cl2 gave a complex mixture including a mixture of (Z)-127 and (E)-127. The same type isomerization as conversion of (Z)-127 to (E)-127 in 99.8% CDCl3 was observed in our previous case.69 Meanwhile, a solution of (Z)-127 in CHCl3 containing EtOH as stabilizer was treated with a small amount of 4M HCl in dioxane to give a mixture of (E)-127 and a trace amount of (4R,5R)-6-tert-butyldimethylsiloxy-3-ethoxy-5-methoxy-4-methyl-2(E)-hexenoate (E)-131 as shown in Scheme 23. This experiment indicated proton (H+)-assisted isomerization of (Z)-127 to (E)-127 to seek for thermodynamically more stable (E)-127 from (Z)-127. In the case of β-methoxy acrylate, it was reported that a trace amount of acidic impurities often was sufficient to bring about rapid equilibration toward the (E)-form from the (Z)-form at ordinary temperatures.78 The thus obtained (+)-88 was subjected to Dess-Martin periodinane oxidation to provide the desired aldehyde (+)-81 in 89% yield. The overall yield (10 steps from (2R,3S)-18a; 23%) of (+)-81 via the present route was improved in comparison to that (10 steps from (2R,3S)-18a; 10%) of the previously case (see 6.2.).58a,b Then, the modified Julia’s coupling of the aldehyde (+)-81 with sulfone (113) in the presence of LHMDS in THF gave a mixture (E/Z = 14/1) of cystothiazole A (67) and (Z)-cystothiazole A 67 in 64% yield. A part of this mixture was again subjected to chromatography to provide (E)-67, whose spectral data (1H- and 13C-NMR) were identical with those of the reported data.58b By applying the modified Julia’s coupling method, selectivity (E/Z=14:1) of the (E)-form (cystothiazole A 67) against the (Z)-form was improved in comparison to the Wittig method (E/Z=4:158b~ 6.9:164).
9. SYNTHESES AND STRUCTURE ELUCIDATION OF CYSTOTHIAZOLE A METABOLITES79
External addition of cystothiazole A (67) to a culture of C. fuscus without adsorbent resin was reported to give a number of polar metabolic derivatives including cystothiazoles B (68), C (69), D (70), melithiazol B (73) and the novel compounds 14-hydroxycystothiazole C (132), (14,15)-dihydroxycystothiazole A (133) and (14,15)-dihydroxycystothiazole C (134)80 as shown in Scheme 24. The absolute structures of 132, 133 and 135 were deduced by a spectroscopic analysis.80 The structure elucidations of the novel compounds 132, 133 and 135 based on their chiral syntheses was carried out. Retrosynthetic strategy for these natural products is illustrated in Scheme 24. It can be seen that these compounds can be synthesized by modified Julia coupling75 between the left half aldehyde (2E)-135 and the right half sulfone A possessing functionality in the side chain (Scheme 24).
9.1. Synthesis of left-half aldehyde (2E)-135
The synthesis of the left-half aldehyde (2E)-135 is shown in Scheme 25. Bis-silylation of (-)-83 (see Scheme 17) gave bis-silyl ether (136) in quantitative yield, which was treated with n-BuLi and methyl chloroformate to afford acetylenecarboxylate 137 in 93% overall yield. Conjugated addition of MeOH to acetylenecarboxylate 137 in the presence of a catalytic amount of Bu3P afforded a single isomer, (2Z)-β-methoxy-α,β-unsaturated ester 138 in 90% yield. In spite of a lack of isomerization of the β-methoxyacrylate moiety in our previous synthesis, isomerization occurred accidentally in the present case and gave the isomerized product (2E)-138 quantitatively when the MeOH addition product (138) was dissolved in aged CDCl3 (available from Cambridge Isotope Laboratories Inc.) for several hours. The structure of the isomerized product (2E)-138 was confirmed to be (E)-olefin due to NOESY observation between the C(2)-hydrogen and C(3)-methoxyl group in (2E)-138, and thence the geometry
of the MeOH addition product (2Z)-138 could be the (Z)-geometry. Both (2Z)-138 and (2E)-138 thus obtained could be converted into the corresponding aldehydes (2Z)-135 and (2E)-135, respectively. Deprotection of the silyl group attached to the primary alcohol group of both (2Z)-135 and (2E)-135 followed by Dess-Martin oxidation afforded the corresponding aldehydes (2Z)-135 and (2E)-135 in 69% and 48% overall yields, respectively (Scheme 25). Isomerization of (2Z)-135 to (2E)-135 in CDCl3 might be explained by the energy difference between (2Z)-135 and (2E)-135 based on the Monte Carlo Multiple Minimum (MCMM) method.81 Low-energy conformations were obtained for both (2E)-135 and (2Z)-135 using the MCMM method with OPLS2005 force fields implemented in MacroModel v.9.5 software. Then, the global energy minima of (2E)-135 and (2Z)-135 were determined with geometry optimization at the HF/6-31G(d.p) level with NMChem v. 4.7 software starting from the low-energy conformers obtained with the MCMM method. The simulation indicated that (2E)-135 is 7.5 kcal/mol more stable than (2Z)-135, which is consistent with the observed result.
9.2. Synthesis of right-half sulfone congeners (R)-144 and (S)-144
Asymmetric dihydroxylation of bis-thiazole (103) with AD-mix α afforded an 83% ee of chiral diol (+)-140 in 90% yield, which was recrystallized from i-PrOH to give (+)-140 (>98% ee}. Likewise, asymmetric dihydroxylation of 103 with AD-mix β afforded a chiral diol (-)-140 in 90% yield, which was
recrystallized from i-PrOH to give optically pure (-)-140. The absolute configuration of (-)-140 was determined to be S by X-ray analysis. This assumption was confirmed by X-ray single crystal structure analysis of (S)-140 as shown in Fig. 3. A crystal of (S)-140, obtained by recrystallization from i-PrOH by slow evaporation of the solvent, with an approximate size 0.2x0.3x0.1 mm3, was employed for X-ray analysis. The compound crystallizes in the orthorhombic space group P212121 with a=5.497(2) Å, b=14.807(5) Å, c=17.658(6) Å, V=1437.2(8) Å3, Z=4, Dc=1.453 g cm-3, µ=3.84cm-1. The intensities were measured on a Rigaku AFC7R/MSC Mercury CCD diffractometer. A total of 7338 reflections utilizing MoKα radiation (λ=0.71070 Å) and 1599 unique reflections were used for structure determination. The structure was solved by the direct method SIR92 and refined by full matrix least squares with anisotropic displacement parameters for the non-hydrogen atoms. The positions of the hydrogen atoms were obtained from difference Fourier maps and refined isotropically. The final R1-value was 0.075 for 5150 reflections with I>2.00σ (I). The absolute configuration was established using the Flack parameter (x=0.2(5)). Figure 3 shows an ORTEP of the refined molecular structure. Treatment of the thus-obtained chiral diols (R)-140 and (S)-140 with 1,2-dimethoxy- cyclopentane in the presence of p-toluenesulfonic acid gave the corresponding ketals (R)-141 and (S)-141 in 82% and 93% yields, respectively. LiBH4 reduction of (R)-141 and (S)-141 afforded the corresponding primary alcohols (R)-142 and (S)-142, which were treated with 2-mercaptobenzothiazole (BTSH) in the presence of Ph3P and diethylazodicarboxylate (DEAD) to provide the corresponding sulfides (R)-143 and (S)-143. The sulfides (R)-143 and (S)-143 were then subjected to oxidation with 35% H2O2 in the presence of Mo7O24(NH4)6·4H2O to give the corresponding sulfones (R)-144 (79% yield from (R)-141) and (S)-144 (73% yield from (S)-141), respectively.
9.3. Synthesis and structure elucidation of 14,15-dihydroxycystothiazole A (133) and 14,15-dihydroxycystothiazole C (134)
The coupling reaction of (2E)-135 and (R)-144 in the presence of LHMDS in THF gave (2E,6E,14R)-145 in 76% yield. Deprotection of the ketal and silyl groups in (2E,6E,14R)-145 using aqueous HF in MeCN gave (14R)-14,15-dihydroxycystothiazole C (134) in 85% yield. Deprotection of the silyl group of (2E,6E,14R)-145 followed by methylation of the secondary alcohol group using Meerwein’s reagent
(Me3O+BF4-) in the presence of a proton sponge provided (2E,6E,14R)-146 (64% overall yield), which was treated with aqueous HF in MeCN to afford (14R)-14,15-dihydroxy-cystothiazole A (133) in 71% yield. The spectral data (1H- and 13C-NMR) of the synthetic (14R)-14,15-dihydroxycystothiazole C (134) were identical to those of the natural product (14R)-134 corresponding to the minor isomer,80 including the sign of specific rotation. On the other hand, the coupling reaction of (2Z)-135 and (R)-144 in the presence of LHMDS in THF gave (2E,6E,14R)-145 (76%) along with (2Z,6E,14R)-145 (4%) in 76% yield. This experiment indicates the occurrence of isomerization of (2Z) to (2E) in the reaction process or work-up step, although the isomerization mechanism has not been clarified. The encouraging result of this experiment prompted to carry out the reaction of (2Z)-135 and (S)-144 in the presence of LHMDS to give (2E,6E,14S)-147 (67%) and (2Z,6E,14S)-147 (8%). Deprotection of the silyl group of (2E,6E,14S)-147 followed by methylation of the secondary alcohol group using Meerwein’s reagent in the presence of a proton sponge provided (2E,6E,14S)-148 (54% overall yield), which was treated with aqueous HF in MeCN to afford (14S)-14,15-dihydroxycystothiazole A (133) in 36% yield. Deprotection of ketal and silyl groups in (2E,6E,14S)-147 using aqueous HF in MeCN gave (14S)-14,15-dihydroxycystothiazole C (134) in 65% yield. The spectral data (1H- and 13C-NMR) of the synthetic (14S)-14,15-dihydroxycystothiazole A (133) and (14S)-14,15-dihydroxycystothiazole C (134) were identical to those of the natural products (14S,15)-13380 and (14S,15)-13480 corresponding to the major isomer, respectively, including the sign of specific rotation (Scheme 27).
9.4. Synthesis of 14-hydroxycystothiazole C (3) and cystothiazole B (2)82
The first chiral synthesis of 14-hydroxycystothiazole C (132) was achieved by the following procedure as shown in Scheme 28. LiBH4 reduction of the hydroxy ester (100) (see Scheme 18) afforded the corresponding primary alcohol (101), which was treated with 2-mercaptobenzothiazole (BTSH) in the presence of Ph3P and diethylazodicarboxylate (DEAD) to provide the corresponding sulfide 149 in 97% yield from 100. Oxidation 100 with 35% H2O2 in the presence of Mo7O24(NH4)6·4H2O gave the corresponding sulfone 150 in 89% yield. The reaction of (2Z)-135 and 150 in the presence of LHMDS gave (2E,6E)-82 in 55% yield. Deprotection of the silyl group of (2E,6E)-151 with aqueous HF in MeCN gave 14-hydroxycystothiazole C (132) in 72% yield. The spectral data (1H- and 13C-NMR) of synthetic 132 were identical to those of the natural product,80 including the sign of specific rotation. Then, methylation of the secondary alcohol group of 132 using Meerwein’s reagent in the presence of a proton sponge provided cystothiazole B (68) in 34% yield along with the starting material 132 (25% yield). The spectral data (1H- and 13C-NMR) of synthetic 68 were identical to those of the natural cystothiazole B (68),55 including the sign of specific rotation (Scheme 28).
9.5. Syntheses of cystothiazoles C (69) and D (70)82
Concise syntheses of cystothiazoles A (67), C (69), D (70),55 and melithiazol B (73)57 was shown in Scheme 29. The reaction of (2Z)-135 and 113 (see Scheme 20) in the presence of LHMDS in THF gave
a mixture (E/Z = 20/1) of coupled products, which were separated to give (2E)-152 (80%) and (2Z)-152 (4%). Deprotection of (2E)-152 with tetrabutylammonium fluoride gave cystothiazole C (69) in 60% yield. The spectral data (1H- and 13C-NMR) of synthetic 69 were identical to those of the natural cystothiazole C (69),55 including the sign of specific rotation. Methylation of synthetic 69 using Meerwein’s reagent (Me3O+BF4-) in the presence of proton sponge provided cystothiazole A (67) in 35% yield along with the starting material 69 (37% recovery). The spectral data (1H- and 13C-NMR) of synthetic 67 were identical to those of the natural cystothiazole A (67),55 including the sign of specific rotation. Next the syntheses of cystothiazole D (3) and melithiazol B (4) were carried out. Treatment of the primary alcohol (104) (see Scheme 18) with 2-mercaptobenzothiazole (BTSH) in the presence of Ph3P and diethylazodicarboxylate (DEAD) followed by oxidation with 30% H2O2 in the presence of Mo7O24(NH4)6·4H2O gave the corresponding sulfone 153 in 69% overall yield. The reaction of (2Z)-135 and 153 in the presence of LHMDS in THF gave a mixture (E/Z = 26/1) of coupled products, which were separated to give (2E)-154 (51%) and (2Z)-154 (2%). Deprotection of (2E)-154 with tetrabutylammonium fluoride gave cystothiazole D (70) in 53% yield. The spectral data (1H- and 13C-NMR) of synthetic 70 were identical to those of the natural cystothiazole D (70),55 including the sign of specific rotation. Thus, the absolute structure of natural cystothiazole D (70) was confirmed by its first synthesis of 70. Methylation of synthetic 70 using Meerwein’s reagent (Me3O+BF4-) in the presence of proton sponge provided melithiazol B (73) in 62% yield. The spectral data (1H- and 13C-NMR) of synthetic 73 were identical to those previously reported melithiazol B (73),71 including the sign of specific rotation (Scheme 29).
10. SYNTHESIS OF CYSTOTHIAZOLE F83
The structure of cystothiazole F (71) was deduced to be 15-hydroxycystothiazole A based on the spectral analysis. The relative stereochemistry (4R*,5S*) could be the same as that of cystothiazole A (1) because of the similar 1H-NMR data (H-4 and H-5).55 The absolute configuration of C(14)-carbon of 71 was not determined. The determination of the absolute structure of cystothiazole F (71) was carried out based on the total synthesis of a chiral form of 71 as shown in Scheme 30.
Retrosynthetically, the synthesis of 71 can be achieved by Wittig condensation of the left-half aldehyde A (= (+)-81) and the right-half chiral phosphonium iodide B. For the synthesis of a chiral form of B ((S)-155 or (R)-155), lipase-catalyzed optical resolution of an alcohol [(±)-156)] or an acetate [(±)-157)] is thought to be the most effective method. The synthesis of substrate [(±)-156 or (±)-157] for enzymatic reaction is shown in Scheme 31.
Hydroboration of the exo-olefin (103) (see Scheme 18) gave a primary alcohol (±)-156 (82% yield), which was subjected to acetylation to afford the corresponding acetate (±)-157 (93% yield). Initially, (±)-155 was subjected to screening experiments using several kinds of commercially available lipases. Among them, lipase “Godo E-1” from Pseudomonas sp. was found to be effective. When (±)-155 was subjected to enantioselective acetylation using “Godo E-1” in the presence of isopropenyl acetate as acylating reagent for 1 day, an acetate (S)-157 (47%, [α]D25 -4.6 (c 1.28, CHCl3); corresponds to 83% ee) and unchanged alcohol (R)-156 (50%, [α]D25 -3.7 (c 1.03, CHCl3); corresponds to 74% ee) were obtained
(entry 1, Table 3). The enantiomeric excess (ee) was calculated by means of HPLC analysis. The E-value of this enzymatic reaction was estimated to be 23.8. The absolute structures of enzymatic reaction products (S)-157 and (R)-157 were determined by the direct comparison of the reported samples84 (R)-157 ([α]D21 +5.0 (c 2.0, CHCl3); corresponds to 81% ee) and (S)-156 ([α]D21 +4.36 (c 1.1, CHCl3); corresponds to 91% ee). When this reaction was carried out for a prolonged period (2 d), (S)-157 (70%, 37% ee) and (R)-156 (28%, 99% ee) were obtained (entry 2, Table 3). On the other hand, asymmetric hydrolysis of (±)-157 for 1 d gave (S)-156 (37%, 99% ee) and (R)-157 (62%, 59% ee) (entry 3, Table 3). The acetate (S)-157 with 37% ee was again subjected to enantioselective hydrolysis to afford (S)-156 (50%, 98% ee) and (R)-157 (44%, 16% ee) (entry 4, Table 3). The synthesis of (4R,5S,6E,14S)- and (4R,5S,6E,14R)-cystothiazole F (71) from the enzymatic productions, (S)- and (R)-156, respectively, is shown in Scheme 32. Protection of the hydroxyl group of (S)-156 as a silyl ether group followed by reduction with LiBH4 gave an (S)-alcohol (159) in 90% overall yield. Treatment of (S)-159 with I2/triphenylphosphine/imidazole provided iodide (S)-160 (89% yield), which was treated with Ph3P to give phosphonium salt (S)-155 in 85% yield. Condensation of (S)-155 with (+)-81 (see Scheme 21) in the presence of LHMDS in THF followed by deprotection of silyl group afforded (4R,5S,6Z,14S)-71 (22%, [α]D26 +206.0 (c 1.36, CHCl3)) and (4R,5S,6E,14S)-71 (66%, [α]D26 +86.2 (c 1.05, CHCl3)). The synthesis of (4R,5S,6Z,14R)-71 (10%, [α]D27 +208.8 (c 0.3, CHCl3)) and (4R,5S,6E,14R)-71 (30%, [α]D26 +94.4 (c 0.78, CHCl3)) from (R)-155 was carried out in the same way as for the synthesis of (4R,5S,6Z,14S)-71 and (4R,5S,6E,14S)-71. The NMR data (1H- and 13C-NMR) of the synthetic (4R,5S,6E,14S)- and (4R,5S,6E,14R)-cystothiazoles F (71) were found to be quite similar to those of natural product cystothiazole F (71),55 respectively. Meanwhile, the sign of specific rotation of the natural 71 [[α]D23 +77.0 (c 0.074, CHCl3)]55 was the same as that of the synthetic (4R,5S,6E,14S)-71
and (4R,5S,6E,14R)-71, respectively. At this stage, identification of natural cystothiazole F (71) and synthetic 71 seemed to be difficult and direct comparison by means of a chiral HPLC analysis was carried out. The result is shown in the Fig. 4, and natural cystothiazole F (71) was found to be a 33:67 diastereomeric mixture [(4R,5S,6E,14S)-71 : (4R,5S,6E,14R)-71 =33:67] (Fugure 4).
11. TOTAL SYNTHESES OF MYXOTHIAZOLS A AND Z85
Myxothiazols A (10) and Z (11) possessing a bithiazole skeleton as well as a β-methoxyacrylate moiety were isolated from the myxobacterium Myxococcus fulvus strain Mxf1656 and Myxococcus fulvus, respectively. Feeding experiments with labeled precursors established biosynthesis of 11 from 10.86 Myxothiazol A (10) is active against many filamentous fungi and completely inhibits growth of Mucor hiemalis at a concentration of 2 µg/ml.56 The fungicidal activity of the β-methoxyacrylate (MOA) inhibitors has been shown to be due to their ability to inhibit mitochondrial respiration by blocking electron transfer between cytochrome b and cytochrome c.87 Myxothiazol Z (11) was reported to exhibit potent cytotoxicity against human tumor cell.88 The structure of myxothiazol A (10) was established by a combination of chemical degradation and NMR study, and its absolute configuration at C(14)-carbon was determined by X-ray analysis of its degradation product.89 The synthesis of a diasteromeric mixture of 10 was achieved based on a Wittig coupling between racemic aldehyde (±)-161 corresponding to the left-half and chiral phosphonium salt (S)-162 corresponding to the right half.59,90 Meanwhile, the synthesis of (+)-11 was reported by a Wittig coupling between chiral aldehyde (4R,5R)-81 and (S)-16290 as shown in Scheme 33. The chiral synthesis of 10 has not been achieved to date and the first synthesis of (+)-10 was obtained based on modified Julia olefination between the chiral aldehyde (4R,5R)-161 and the chiral benzothiazole sulfone (S)-163. Furthermore, the synthesis of (+)-11 was obtained based on modified Julia olefination between a (4R,5R)-81 and (S)-163 (Scheme 33).
11.1. Synthesis of left-half (4R,5R)-81 and (4R,5R)-161
The synthesis of (±)-161 was achieved in overall 1% yield (9 steps) based on a condensation reaction between cinnamaldehyde and the dianion derived from methyl 3-oxopentanoate followed by several synthetic steps.59,90 In this case, the preparation of chiral form of 161 could be possible due to the optical resolution of the intermediate. On the other hand, the synthesis of (4R,5R)-81 was reported based on Evans asymmetric aldol condensation procedure.90 The preparation of (4R,5R)-161 was achieved by the following synthetic route as shown in Scheme 34.
The silyl ether 125 (Scheme 21) was treated with n-BuLi and methyl chloroformate to give an acetylenecarboxylate 126 in 88% yield. Conjugate addition of MeOH to 126 followed by isomerization under the previously mentioned procedure (Scheme 21) gave (E)-127 in 82% overall yield. Desilylation of (E)-127 with Et3N·(HF)3 followed by oxidation with Dess-Martin reagent gave the desired aldehyde (4R,5R)-81 in 84% yield. Conversion of ester group of (E)-127 to amide was carried out by the following procedure. Alkaline hydrolysis of (E)-127 followed by acid treatment gave a carboxylic acid. Treatment of this acid with water-soluble carbodiimide hydrochloric acid salt (WSCD·HCl) in the presence of 1-hydroxy-7-aza-benzotriazole (HOAt) followed by addition of aqueous NH3 gave the desired amide (E)-164 in 39% overall yield from (E)-127. Desilylation of (E)-127 with HF·pyridine followed by oxidation with tetrapropylammonium perruthenate (TPAP) in the presence of 4-methylmorpholine N-oxide (NMO) and MS-4A afforded the desired aldehyde (4R,5R)-161 in 32% overall yield. 1H-NMR data of the synthetic (4R,5R)-161 were consistent with those of the reported (±)-161.90
11.2. Synthesis of right-half (S)-163, myxothiazols A (10) and Z (11)
Treatment of chiral alcohol (S)-156 (see Table 3) with 2-mercaptobenzothiazole (BTSH) in the presence of Ph3P and diethyl azodicarboxylate (DEAD) gave the corresponding sulfide (S)-165 in 93% yield. LiBH4 reduction of (S)-165 followed by oxidation with 35% H2O2 in the presence of Mo7O24(NH4)6·4H2O provided the corresponding sulfone-alcohol, which was again treated with 2-mercaptobenzothiazole (BTSH) in the presence of Ph3P and DEAD to afford the corresponding sulfide (S)-166 in 66% overall yield. The reaction of (S)-166 and (2E)-4-methylpentenal in the presence of LHMDS in THF gave a mixture (E/Z = 3.3/1) of coupled products, which were chromatographically separated to give (1S,2E)-167 (59%) and (1S,2Z)-168. Oxidation of (1S,2E)-167 under the same condition as preparation of (S)-166 provided the desired (S)-163 in 77% yield. The overall yield of (S)-163 from (S)-156 was 28% (4 steps). In contrast, the overall yield of (S)-162 from the commercially available (2R)-3-hydroxy-2-methylpropanoate was 1% (19 steps).59,90 Finally, modified Julia coupling between the chiral aldehyde (4R,5R)-161 and the chiral benzothiazole sulfone (S)-163 in the presence of LHMDS afforded (+)-myxothiazol A (10) in 61% yield. The spectral data of the synthetic 10 were identical with those of natural (+)-myxothiazol A (10)56 including the sign of a specific rotation. Julia coupling between a chiral aldehyde (4R,5R)-81 (see Scheme 21) and a chiral benzothiazole sulfone (S)-163 in the presence of LHMDS afforded (+)-myxothiazol Z (11) in 73% yield. The spectral data of the synthetic 11 were identical with those of natural (+)-myxothiazol Z (11)88 including the sign of a specific rotation.
12. CONCLUSION
Diastereoselective synthesis of (±)-(2,3)-syn-2-methyl-3-hydroxy ester I or (±)-(2,3)-syn-3-methyl-2- hydroxy ester III was achieved based on diastereoselective reduction of (±)-2-methyl-3-keto ester IV or the reaction of (±)-trans-epoxybutanoate V and carbon-nucleophile, respectively. These racemic alcohols were subjected to enzymatic resolution to afford the corresponding enantiomers. Each enantiomerically pure compound was converted to biologically active natural products such as oudemansins, chuangxinmycin, asperlin, indolmycin, cystothiazoles, melithiazols and myxothiazols possessing antifungal and cytotoxic activities, inhibition of NADH oxidation, etc.
References
1. J. M. Clough, Natural Product Reports, 1993, 565. CrossRef
2. H. Sauter, W. Steglich, and T. Anke, Angew. Chem. Int. Ed., 1999, 1328. CrossRef
3. T. Anke, H. J. Hecht, G. Schramm, and W. Steglich, J. Antibiot., 1979, 32, 1112.
4. T. Anke, H. Besl, U. Mocek, and W. Steglich, J. Antibiot., 1983, 36, 661.
5. T. Anke, A. Werle, M. Bross, and W. Steglich, J. Antibiot., 1990, 43, 1010.
6. H. Akita, H. Koshiji, A. Furuich, K. Horikoshi, and T. Oishi, Tetrahedron Lett., 1983, 24, 2009. CrossRef
7. T. Nakata, T. Kuwabara, Y. Tani, and T. Oishi, Tetrahedron Lett., 1982, 23, 1015. CrossRef
8. H. Akita, A. Furuichi, H. Koshiji, K. Horikoshi, and T. Oishi, Tetrahedron Lett., 1982, 23, 4051. CrossRef
9. H. Akita, A. Furuichi, H. Koshiji, K. Horikoshi, and T. Oishi, Chem. Pharm. Bull., 1983, 31, 4384.
10. J. A. Dale and H. S. Mosher, J. Am. Chem. Soc., 1973, 95, 512. CrossRef
11. T. Nakata and T. Oish, Tetrahedron Lett., 1980, 21, 1641. CrossRef
12. H. Akita, H. Matsukura, and T. Oishi, Tetrahedron Lett., 1986, 27, 5241. CrossRef
13. H. Akita, H. Matsukura, and T. Oishi, Tetrahedron Lett., 1986, 27, 5397. CrossRef
14. J. Kallmerten, and M. D. Wittman,. Tetrahedron Lett., 1986, 27, 2443. CrossRef
15. W. R. Roush, T. A. Blizzard, and F. Z. Basha, Tetrahedron Lett., 1982, 23, 2331. CrossRef
16. H. Akita, R. Todoroki, H. Endo, Y. Ikari, and T. Oishi, Synthesis, 1993, 513. CrossRef
17. T. Mukaiyama, and M. Murakami, Chem. Lett., 1981, 1129. CrossRef
18. H. Akita, T. Kawaguchi, Y. Enoki, and T. Oishi, Chem. Pharm. Bull., 1990, 38, 323.
19. H. Akita, I. Umezawa, M. Nozawa, and S. Nagumo, Tetrahedron Asymm., 1993, 4, 757. CrossRef
20. I. Umezawa, M. Nozawa, S. Nagumo, and H. Akita, Chem. Pharm. Bull., 1995, 43, 1111.
21. H. Akita, C-Y. Chen, and S. Nagumo, Tetrahedron: Asymm., 1994, 5, 1207. CrossRef
22. H. Akita, C-Y. Chen, and S. Nagumo, J. Chem. Soc., Perkin Trans. I, 1995, 2159. CrossRef
23. K. Kato, M. Ono, and H. Akita, Tetrahedron Lett., 1997, 38, 1805. CrossRef
24. K. Kato, M. Ono, and H. Akita, Tetrahedron: Asymm., 1997, 8, 2295. CrossRef
25. K. Kato, M. Ono, and H. Akita, Tetrahedron, 2001, 57, 10055. CrossRef
26. For a review concerning biological activity and syntheses, see ref. 25 and references cited therein.
27. C.-S. Chen and C. J. Sih, Angew. Chem. Int. Ed. Engl., 1989, 28, 695. CrossRef
28. H. Akaike, H. Horie, K. Kato, and H. Akita, Tetrahedron: Asymm., 2008, 19, 1100. CrossRef
29. A. D. Argoudelis and J. Z. Zieserl, Tetrhedron Lett., 1966, 7, 1969. CrossRef
30. K. Fukuyama, Y. Katsube, A. Noda,T. Hamasaki, and Y. Hatsuda, Bull. Chem. Soc. Jpn., 1978, 51, 3175. CrossRef
31. S. Valverde, B. Herradon,R. M. Rabanal, and M. Martin-Lomas,. Can. J. Chem., 1986, 65, 339. CrossRef
32. a) H. Hiraoka, K. Furuta, K, N. Ikeda, and H. Yamamoto, Bull. Chem. Soc. Jpn., 1984, 57, 2777; CrossRef b) T. Murayama, T. Sugiyama, and K. Yamashita, Agric. Biol. Chem., 1987, 51, 2055; c) R. H. Schlessinger and K. W. Gillman, Tetrahedron Lett., 1999, 40, 1257. CrossRef
33. S. Ramesh and R. W. Franck, Tetrahedron:Asymm., 1990, 1, 137. CrossRef
34. Y. Masaki, T. Imaeda, H. Oda, A. Itoh, and M. Shiro, Chem. Lett., 1992, 1209. CrossRef
35. a) T. K. M. Shing and M. Aloui, Can. J. Chem. 1990, 68, 1035; CrossRef b) A. M. Gomez, B. A. M, Lopez de Uralde, S. Valverde, and J. Lopez, Chem. Commum., 1997, 1647. CrossRef
36. a) T. Honda, N. Sano, and K. Kanai, Heterocycles, 1995, 41, 425; CrossRef b) Z-C. Yang, and W-S. Zhou, Tetrahedron Lett., 1995, 36, 5617; CrossRef c) T. Honda, H. Mizutani, and K. Kanai, J. Chem. Soc., Perkin Trans. 1, 1996, 1729; CrossRef d) Z-C. Yang, X-B.Jiang, Z-W. Wang, and W-S. Zhou, J. Chem. Soc., Perkin Trans. 1, 1997, 317; CrossRef e) K. Kanai, N. Sano, and T. Honda, Heterocycles, 1999, 50, 433. CrossRef
37. M. Lombardo, R. Girotti, S. Morganti, and C. Trombini, Org. Lett., 2001, 3, 2981. CrossRef
38. Handbook of Metathesis; WILEY-VCH 2003, ed. by R. H. Grubbs.
39. M. Lombardo, K. Gianotti, S. Licciulli, and C. Trombini, Tetrahedron, 2004, 60, 11725. CrossRef
40. M. Scholl, S. Ding, C. W. Lee, and R. H. Grubbs, Org. Lett., 1999, 1, 953. CrossRef
41. M. Lombardo, S. Morganti, and C. Trombini, J. Org. Chem., 2003, 68, 997. CrossRef
42. L. A. Paquette and T. M. Mitzel, J. Am. Chem. Soc., 1996, 118, 1931. CrossRef
43. N. Sutou, K. Kato, and H. Akita, Tetrahedron: Asymm., 2008, 19, 1833. CrossRef
44. a) K. V. Rao, Antibiot. Chemother., 1960, 10, 312; b) W. S. Marsh, A. L. Garretson, and E. M. Wesel, ibid., 1960, 10, 316.
45. R. F. Werner and A. Demain, J. Antibiot., 1981, 34, 551.
46. T. Kanamaru, Y. Nakano, Y. Toyoda, K. Miyagawa, M. Tada, T. Kaisho, and M. Nakao, Antimicrob. Agents Chemother., 2001, 45, 2455. CrossRef
47. a) J. P. Dirlam, D. A. Clark, and S. J. Hecker, J. Org. Chem., 1986, 51, 4920; CrossRef b) Y. –K. Shue, Tetrahedron Lett., 1996, 37, 6447. CrossRef
48. T. Takeda and T. Mukaiyama, Chem. Lett., 1980, 163. CrossRef
49. M. N. Preobrazhenskaya, E. G. Balashova, K. F. Turchin, E. N. Padeiskaya, N. V. Yvarova, G. N. Pershin, and M. N. Suvorov, Tetrahedron, 1968, 24, 6131. CrossRef
50. A. Hasuoka, Y. Nakayama, M. Adachi, H. Kamiguchi, and K. Kamiyama, Chem. Pharm. Bull., 2001, 49, 1604. CrossRef
51. R. C. Larock and E. K. Yum, J. Am. Chem. Soc., 1991, 113, 6689. CrossRef
52. H. Akita, N. Sutou, T. Sasaki, and K. Kato, Tetrahedron, 2006, 62, 11592. CrossRef
53. L. M. Deborah, D. Marcin, K.-C. Teresa, I. T. Nadya, and J. M. Christopher, Bioorg. Med. Chem. Lett., 2007, 17, 2380. CrossRef
54. T. Bando, and K. Shishido, Heterocycles, 1997, 46, 111. CrossRef
55. a) M. Ojika, Y. Suzuki, A. Tsukamoto, Y. Sakagami, R. Fudou, T. Yoshimura, and S. Yamanaka, J. Antibiot., 1998, 51, 275; b) Y. Suzuki, M. Ojika, Y. Sakagami, R. Fudou, and S. Yamanaka, Tetrahedron, 1998, 54, 11399. CrossRef
56. K. Gerth, H. Irschik, H. Reichenbach, and W. Trowitzsch, J. Antibiot. 1980, 33, 1474.
57. B. Böhlendrof, M. Herrmann, H.-J. Hecht, F. Sasse, E. Forche, B. Kunze, H. Reichenbach, and G. Höfle, Eur. J. Org. Chem., 1999, 2601. CrossRef
58. a) K. Kato, A. Nishimura, Y. Yamamoto, and H. Akita, Tetrahedron Lett., 2002, 43, 643; CrossRef b) K. Kato, T. Sasaki, H. Takayama, and H. Akita, Tetrahedron, 2003, 59, 2679. CrossRef
59. B. J. Martin, J. M. Clough, G. Pattenden, and I. R. Waldron, Tetrahedron Lett., 1993, 34, 5151. CrossRef
60. D. Backhaus, Tetrahedron Lett., 2000, 41, 2087. CrossRef
61. D. R. Williams, S. Patnaik, and M. P. Clark, J. Org. Chem., 2001, 66, 8463. CrossRef
62. U. Söker, F. Sasse, E. Forche, B. Kunze, and G. Höfle, Eur. J. Org. Chem., 2000, 1497. CrossRef
63. T. Bach and S. Heuser, Chem Eur. J., 2002, 8, 5585. CrossRef
64. P. L. DeRoy and A. B. Charette, Org. Lett., 2003, 5, 4163. CrossRef
65. M. Ojika, T. Watanabe, J. Qi J, T. Tanino, and Y. Sakagami, Tetrahedron, 2004, 60, 187. CrossRef
66. J. S. Panek and J. Shao, Org. Lett., 2004, 6, 3083. CrossRef
67. J. Gebauser, S. Arseniyadis, and J. Cossy, Eur. J. Org. Chem., 2008, 2701. CrossRef
68. T. Bach and S. Heuser, Angew. Chem. Int. Ed. 2001, 40, 3184. CrossRef
69. K. Kato, A. Nishimura, Y. Yamamoto, and H. Akita, Tetrahedron Lett., 2001, 42, 4203. CrossRef
70. T. Sasaki, K. Kato, and H. Akita, Chem. Pharm. Bull., 2004, 52, 770. CrossRef
71. H. Akita, T. Sasaki, H. Takayama, and K. Kato, Heterocycles, 2005, 66, 219. CrossRef
72. H. Akita, T. Sasaki, K. Kato, Y. Suzuki, K. Kondo, Y. Sakagami, M. Ojika, R. Fudou, and S. Yamanaka, Tetrahedron, 2004, 60, 4735. CrossRef
73. H. Takayama, K. Kato, and H. Akita, Eur. J. Org. Chem., 2006, 644. CrossRef
74. H. Takayama, K. Kato, M. Kimura, and H. Akita, Heterocycles, 2007, 71, 75. CrossRef
75. P. R. Blakemore, J. Chem. Soc., Perkin Trans. 1, 2002, 2563. CrossRef
76. J. Tsuji, M. Takahashi, and T. Takahashi, Tetrahedron Lett., 1980, 21, 849. CrossRef
77. J. Inanaga, Y. Baba, and T. Hanamoto, Chem. Lett., 1993, 241. CrossRef
78. S. J. Rhoads, J. K. Chattopadhyay, and E. E. Waali, J. Org. Chem., 1970, 35, 3352. CrossRef
79. Y. Iwaki, S. Yamamura, and H. Akita, Tetrahedron: Asymm., 2008, 19, 2192. CrossRef
80. Y. Suzuki, M. Ojika, and Y. Sakagami, Biosci. Biotecnol. Biochem., 2004, 68, 390. CrossRef
81. a) G. Chang, W. C. Guida, and W. C. Still, J. Am. Chem. Soc., 1989, 111, 4379; CrossRef b) M. Saunders, K. N. Houk, and Y. D. Wu, J. Am. Chem. Soc., 1990, 112, 1419. CrossRef
82. Y. Iwaki and H. Akita, Chem. Pharm. Bull., 2007, 55, 1610. CrossRef
83. H. Akita, Y. Iwaki, K. Kato, J. Qi, and M. Ojika, Tetrahedron: Asymm., 2007, 18, 513. CrossRef
84. H. Akita, M. Nozawa, and S. Nagumo, Chem. Pharm. Bull., 1994, 42, 1208.
85. a) Y. Iwaki, M. Kaneko, and H. Akita, Tetrahedron Lett., 2008, 49, 7024; CrossRef b) Y. Iwaki, M. Kaneko, and H. Akita, Tetrahedron: Asymm., 2009, 20, 298. CrossRef
86. H. Steinmetz, E. Forche, H. Reichenbach, and G. Höfle, Tetrahedron, 2000, 56, 1681. CrossRef
87. G. Thierbach and H. Reichenbach, Biochim. Biophys. Acta, 1981, 638, 282. CrossRef
88. J-W. Ahn, S-H. Woo, C-O. Lee, K-Y. Cho, and B-S. Kim, J. Nat. Prod., 1999, 62, 495. CrossRef
89. a) W. Trowitzsch, G. Reifenstahl, V. Wray, and K. Gerth, J. Antibiot., 1980, 33, 1480; b) W. Trowitzsch, G. Höfle, and W. S. Sheldrick, Tetrahedron Lett., 1981, 22, 3829. CrossRef
90. J. M. Clough, H. Dube, B. J. Martin, K. S. G. Pattenden, Reddy, and I. R. Waldron, Org. Biomol. Chem., 2006, 4, 2906. CrossRef