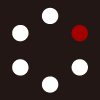
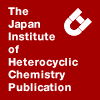
HETEROCYCLES
An International Journal for Reviews and Communications in Heterocyclic ChemistryWeb Edition ISSN: 1881-0942
Published online by The Japan Institute of Heterocyclic Chemistry
e-Journal
Full Text HTML
Received, 3rd February, 2009, Accepted, 11th March, 2009, Published online, 12th March, 2009.
DOI: 10.3987/COM-09-11674
■ Structure and Conformation of Photosynthetic Pigments and Related Compounds. 13. Identification of Localized Vibrational Modes in Chlorophyll A Derivatives
Mathias O. Senge*
School of Chemistry, SFI Tetrapyrrole Laboratory, Trinity College Dublin, Dublin 2, Ireland
Abstract
A Resonance Raman investigation of chlorophyll a and meso-chlorinated and ring V hydroxylated derivatives and pheophytins reveals localized vibrational modes in the chlorophyll series. Both chlorination and hydroxylation give rise to changes in both band intensity and frequency throughout the spectrum. Chlorination affects mainly the core sensitive bands with CaCm and CaN character. Hydroxylation leads to changes in the carbonyl and ring V associated bands. Small, but real spectral differences were found between the 10-epimers.INTRODUCTION
Chlorophylls are the main accessory and reaction center pigments in the photosynthetic apparatus of Higher Plants and algae. Due to their fundamental relevance these porphyrin-based heterocyclic pigments have been studied in detail.1 A fundamental question relates to the conformational flexibility of these pigments, i.e. whether and to what extend the tetrapyrrole macrocycle is flexible and can occur in different conformations.2 Next to static X-ray crystallography,3 vibrational spectroscopic techniques such as resonance Raman (RR) are crucial techniques for an investigation of their solution conformation and for the elucidation of their in vivo function.4 More specific studies have addressed the metal and axial ligand coordination in chlorophyll a (Chl a),5 normal mode characteristics in synthetic chlorins,6 liquid crystals,7 metallochlorins,8,9,10 amongst others.
Figure 1. Compounds studied.While most of these studies have used either unaltered Chl's or synthetic analogues, not much attention has been given to chemically, slightly altered Chl deriviatives. The only exception is the study of the chlorophyll allomerization reaction, which provides useful information on Chl alteration and structural parameters.11 Here we provide data on meso-chlorinated Chl's, some allomerization derivatives and the related free base pheophytins in order to elucidate any localized vibrational modes suitable for conformational analysis.
In particular, the 10-hydroxylated derivatives are very suitable for RR studies because the absence of the acidic proton at position 10 prevents epimerization which otherwise occurs rapidly in polar organic solvents. These compounds were studied with Soret excitation in dry diethylether (Et2O) and in tetrahydrofuran (THF) to provide two different solvent systems with distinct coordination at the central magnesium atom, the coordination number being five in ether and six in THF.12 The chlorophyll epimers (Chl a')13 and the corresponding pheophytins (Pheo) were prepared and studied for comparison. These derivatives are structurally different variants of the chlorophylls, which can be employed in the elucidation of the vibrational spectra. They are known to play an important role in photosynthesis; two bacteriopheophytins are an integral part of the reaction center of photosynthetic bacteria and they have also been shown to occur in the photosystems of Higher Plants.14 Both chlorination and hydroxylation give rise to changes in both band intensity and frequency throughout the spectrum. Chlorination effects mainly the core sensitive bands with CaCm and CaN character. Hydroxylation leads to changes in the carbonyl and ring V associated bands. Small, but real spectral differences were found between the 10-epimers. This investigation continues our earlier studies on the conformation and structure of photosynthetic pigments15 and complements related studies on the mass spectrometric analysis of ring V chlorophyll derivatives.16
RESULTS
The structures of Chl a and its derivatives are displayed in Figure 1. The meso substituted chloro-derivatives (δ-Cl-Chl a) is modified (relative to Chl a) at the δ-position and the ring V modifications are hydroxylation (10-OH-Ch1 a) and epimerization (Ch1 a’) at position 10. The absorption spectra (see experimental section) are very similar to that of Ch1 a; however there is a 6 nm bathochromic shift of the Qy-band of δ-Cl-Ch1 a and a slight shift in the Soret region. The similarity of the absorption spectra indicates that the π-system is not greatly perturbed by the Cl-substituent and that the red shifts may be caused by the inductive effect of the halogen substitution. As expected, the 10-OH-Ch1 a substitution has very little effect on the electronic structure of the macro cyclic ring because this position is out of the π-configuration pathway. Similarly, only small effects are seen for the epimers.
Resonance Raman Spectra of Hydroxy- and Chloro-Substituted Ch1 a
The high frequency Soret excitation spectra of Ch1 a and the 10-OH and δ-Cl-derivatives are displayed in Figure 2 and tabulated in Table 1. Excitation at 413.2 nm affords good quality spectra throughout the high frequency region (1000-1800 cm-1) and reasonable quality spectra in low frequency region (200-1000 cm-1). The high frequency region is characterized by in-plane stretching and bending vibrations and the low frequency portion of the spectrum is interpreted as arising predominantly from in- and out of plane bending vibrations. The high frequency RR spectra of 10-OH and δ-Cl-derivatives look fairly similar to unmodified Ch1 a, which suggests that there are not major perturbation of the chlorine ring structure and that the Ch1 a normal coordinate calculations may also pertain to the chemically modified chlorophyll ring structure.
The bands that shift upon 10-OH substitution (Figure 2a compared to b) are: 1698 → 1707 cm-1, 1433 → 1430 cm-1, 1221 → 1223 cm-1 and 1881 → 1175 cm-1 (Table 3). The core-size sensitive vibrations at 1607, 1554 and 1491 cm-1 remain constant. The effects of δ-Cl-substitution (Figure 2a vs. c) are: 1607 → 1600 cm-1, 1491 → 1501 cm-1, changes in the 1388 and 1375 cm-1 bands to two bands at 1409 and 1388 cm-1, 1346 →1342 cm-1, 1287 →1282 cm-1, 1221 → 1224 cm-1, loss of intensity of the 1199 cm-1 band and shift of the 1181 cm-1 band to 1175 cm-1. The 1181 cm-1 band is similarly affected by 10-OH-substitution. The C9=O ketone stretching frequency remains fairly constant at 1698-1700 cm-1 as does the most intense band in the spectrum at 1555 cm-1.
The high frequency RR spectrum of di-substituted 10-OH-δ-Cl-Ch1 a derivative in Figure 2d is essentially the summation of the individual substitution effects seen in the Figure 3b and c. The 10-OH band shift relative to Ch1 a are observed at 1698 → 1708cm-1, 1433 → 1427 cm-1, 1221 → 1224 cm-1 and downshift of the 1881 cm-1 band. The δ-Cl individual substituent band shifts are observed at 1607 → 1598 cm-1, 1491 → 1501 cm-1, changes in the 1388-1375 cm-1 region, 1346 →1343 cm-1, 1287 → 1282 cm-1, loss of the 1199 cm-1 band and the downshift of the 1182 cm-1 band to 1166 cm-1. These frequency downshifts also seem to be additive for the 10-OH-δ-Cl- derivative which displays a 1182 to 1166 (delta = 16 cm-1) shift (relative to Ch1 a) compared to a 7 cm-1 shift for the individually substituted compounds. The same band shifts are observed with 406.7 nm laser excitation (data not shown).
Figure 4 presents the high frequency RR spectra of Chl a and its derivatives in THF (see also Table 1). In THF the central Mg2+ is six coordinate whereas in ether it displays typical five coordinate marker vibration.12 The differences in the RR spectrum of Chl a between five-coordinate Mg2+ in the ether and six coordination are a 5-10 cm-1 downshift of the vibrations >1400 cm-1. The ring vibration frequency shifts to 1597, 1547, 1530, 1485 and 1431 cm-1 are caused by expansion of hydrophorbin in ring as the central metal moves into the plane of the ring. The C9=O stretching frequency is sensitive to coordination number as seen by the 7 cm-1 downshift. Krawczyk has discussed the sensitivity of this band to both coordination number and dielectric constant of the environment.17 The vibrations <1400 cm-1 are essentially constant with changes in coordination number with the exception of the shift of the 1286 cm-1 band to 1289 cm-1.
Hydroxylation of Chl a at the C-10 position results in the following band shifts in THF (Figure 4b): 1696 → 1701 cm-1, 1597 → 1595 cm-1, the appearance of the distinct shoulder at 1576 cm-1, 1431 → 1428 cm-1, 1220 →1222 cm-1 and 1180 → 1175 cm-1. The band shifts and magnitudes are similar to those observed in the ether with the minor exceptions of a) a larger shift of the γ (C9=O) in THF, b) the small (2 cm-1)
shift of the 1597 cm-1 band in THF and c) the appearance of the band at 1576 cm-1. The additional shifts of two high frequency bands could be caused by an enhanced sensitivity of the macrocyclic ring owing the “strain” induced by six coordination.
Chlorination at the δ-methine position produces the following shifts relative to Chl a in THF (Figure 4c): 1696 → 1701 cm-1, 1597 → 1590 cm-1, the appearance of shoulder at 1575 cm-1, 1547 → 1544 cm-1, a shift of the 1533 cm-1, shoulder to 1527 cm-1, 1485 → 1495 cm-1, changes in the 1400-1375 cm-1 region, 1289 →1284, 1220 → 1226 cm-1, disappearance of the 1203 cm-1 band and 1180 → 1174 cm-1 shift. Again, these shifts, directions and magnitudes are very similar to those observed in the ether with the exceptions of the additional shifts present in the highest frequency bands, the presence of a new shoulder at 1626 cm-1 and the absence of the band shift at 1342 cm-1. The shoulder at 1626 cm-1 could be γ (C=C) and it is observed in these samples because of the downshift of the intense 1597 cm-1 band. The disubstituted derivative (Figure 4d) displays the additive effects of both 10-HO and δ-Cl substitutions. Table 2 compiles the frequency shifts observed upon hydroxylation and chlorination.
Chemical changes in chlorine periphery have been known to alter the equilibrium constant of ligand binding but the high frequency vibrational shifts observed here for the series of derivatives in one solvent are not consistent with changes in ligation. In particular, the opposing shifts of -8 cm-1 and +10 cm-1 of the 1597 and 1485 cm-1 sore-size sensitive bands, respectively, that typically display parallel shifts, argue against simple changes in ligation state. The additivity of the vibrational shifts implies that the chemical changes in one part oh the chlorophyll molecule do not effect all of the molecular vibrations and two different sets of vibrations are being altered with the ring V hydroxy- and δ-methine chloro-substitutions.
Epimers
Soret excitation RR spectra of the epimers of Chl a and its hydroxy- and chloro-substituted derivatives are displayed in Figure 5. Chl a’ and δ-Cl-Chl a’ were prepared according the method of Hynninen and Lötjonen.18 Epimerization proceeds via the C-10 acidic proton which deprotonates under basic conditions allowing scrambling of the stereochemistry at the 10-carbon position. Because of this, the epimers of the 10-hydroxy derivatives of Chl a were made by the hydroxylation of previously prepared samples of Chl a’. Therefore, hydroxy derivatives are especially stable for racemization.
The spectra of the series of compounds in Figure 5 display the same overall pattern of shifts seen in Figures 2 and 3. Therefore, epimerization is a minor perturbation on the Ch1 a vibrational structure. However, there are small, but consistent, changes between the corresponding compounds in Figures 3 and 5. The band shifts are tabulated in Table 3. The bands that shifts (most consistently) throughout the series are: a slight downshift of γ C9=O in the chlorinated derivatives, b) a 1-2 cm-1 downshift of the 1600-1610 cm-1 band, c) an upshift in the band at 1430 cm-1, d) a shift of the 1220 cm-1 band, and e) a 2-3 cm-1 upshift of the 1165-1180 cm-1 band. These vibrations are the same bands that are affected by hydroxylation, which indicates that the Chl vibrations that are affected by epimerization are those located at ring V.
In this matrix of band shifts there are several of the above vibrations that remain constant (Table 2). In some cases these bands are at the extreme of the observed range, for example, there is no noticeable downshift of the 1598 cm-1 band of 10-hydroxy-δ-chloro-Chl a. Also, the spectrum of the 10-OH derivative displays two bands in this group which do not shift at 1430 and 1175 cm-1. The acidic proton of this derivative has already been replaced by a bulky OH-group so a change in stereochemistry at the C-10 position may not induce an additional increase in the ring strain. This may explain why this derivative is least sensitive to epimerization.
Pheophytins
The high and low frequency RR spectra of hydroxy and chloro-derivatives of Pheo a are presented in Figure 6 and 7. The RR spectrum of Pheo a differs from Chl a by 5-30 cm-1 upshift of most of the vibrations in the core-size sensitive region (>1400 cm-1). This is the result of the smaller core size of Pheo a relative to Chl a.3a The major bands that shift between Pheo a and 10-OH-Pheo a are; γ C9=O shifts from 1710-1716 cm-1, the 1307 cm-1 band appears to split into two bands at 1310 and 1296 cm-1 and the 1130 band shifts down to 1126 cm-1. The difference between Pheo a and δ-Cl- Pheo a are: 1710 → 1715 cm-1, 1498 → 1512 cm-1, 1350 → 1354cm-1, 1307 → 1309 cm-1, 1224 → 1227 cm-1 and 1130 → 1133 cm-1.
The disubstituted 10-OH-δ-Cl- Pheo a derivatives displays the following band shifts; the frequency upshift of γ C9=O from 1710 → 1719 cm-1, a decrease in intensity and possible frequency downshift of the 1630 cm-1 band, 1498 → 1512 cm-1, 1350 → 1353 cm-1, 1307 → 1315 cm-1, 1224 → 1226 cm-1, and a frequency shift of 1130 → 1126 cm-1. Although the band shifts are not as distinct as those observed for Chl a, a similar pattern emerges. These is a frequency upshift of γ C9=O and the core-size marker band at 1498 cm-1. The larger downshift of the highest frequency core-size marker band is less obvious than for the Chl a derivatives. In the low frequency RR spectrum of the pheophytin derivatives (Figure 7) all bands are shifted.
DISCUSSION
Chlorophyll a ― Band Shifts and Assignments
Chemical substitution(s) of complex molecules can aid in the interpretation of their vibrational properties; However, the perturbations must be small in order to correlate the vibrations from one molecule to another. Here we consider the following possibilities to interpret the hydroxy- and chloro-substituted Chl a derivatives: 1) ring puckering or out-of-plane distortions, 2) changes in vibrational mode composition and 3) a localization of vibrational modes in the specific quadrants of Chl a macrocycle. Ring strain induced by chemical substitutions can be alleviated by flexibility in the chlorophyll macrocyclic ring.2,19,20 This S4-ruffing or ring puckering effects is manifest as a frequency downshift of the core-size sensitive vibrations, e.g., D2d form of NiOEP.21 For the hydroxy-substituted derivative two core-size sensitive bands at 1600 and 1430 cm-1 shift down slightly in the frequency relative to Chl a.22 This may be an indication of ring ruffling. However, for δ-Chloro-substituted Chl a both frequency downshifts (1609 cm-1) and upshifts (1490 cm-1) are observed for the methane bridge stretching vibrations. This is inconsistent with the shifts expected for the chlorin core ruffling.
The possibilities of changes in vibrational mode composition can be recognized as inconsistent band shifts. For example, deuteration produces frequency downshifts in C-H bending and stretching modes as the result of a pure isotope effect but the observation of frequency upshifts in these bands would be indicative of a remixing of the vibration modes.6a,23 To establish a consistent pattern of band shifts of the hydroxy- and chloro-substituted derivatives, we have obtained RR spectra of these compounds in two different coordination states: five-coordinate in ether and six-coordinate in THF. In both ligation states the corresponding bands shift, both up and down in frequency, with approximately the same magnitude. This is an indication that the character of the vibrational modes remains constant.
The third possibility for the Chl a derivatives vibrational band shifts is that of localized mode description of the vibrations. This has been previously suggested by Boldt et al.6a In this case we would expect to observe a possible inconsistent pattern of band shifts because vibrations in one part of molecule would be affected differently than those in another quadrant.
The observed high frequency band shifts for δ-chloro-Chl a are at 7 cm-1 downshift of the 1600 cm-1 band and a 10 cm-1 upshift of 1490 cm-1 band. Both of these vibrations are core-size sensitive and have been assigned as having predominantly CaCm character. Because of the opposite frequency shifts in these vibrations with similar mode character, the band shifts observed for δ-chloro- and also the 10-OH-Chl a derivatives are interpreted as arising, in part, from localized vibrational mode.
10-Hydroxy Derivative
The type of localized vibrations that one might expect to shift upon hydroxylation at the C-10 position can be determined by examination of Ch1 a structure. These projected vibrational shifts are: addition of γ (C10-OH) in the range 1000-1150 cm-1, and shifts in γ CmC10, γ C9=O, γ CaCm(δ), γ C2aaN (III, IV) and (possibly) δ CbH (IV). There are no new bands observed in the crowded 1000-1200 cm-1 region, so we are unable to detect the appearance of γ C10-OH band. As predicted the C9 ketone stretching band is affected by C10-hydroxylayion. This band shifts up by 2 cm-1 and 5 cm-1 in ether and THF, respectively, owing to the increase in the ring strain.
Two core-size sensitive vibrations at 1607 and 1433 cm-1 in ether and 1597 and 1431 in THF are slightly sensitive to hydroxylation and have been assigned as , γ CaCm(α, β) at 1600 cm-1 and [ð CH2 (V) and γ CaCm(ð,δ)] at 1430 cm-1 (Table 2). These shifts may be indicative of core ruffling. The mid-frequency range vibrations that are shifted by hydroxylation at 1220 and 1180 cm-1 are assigned to vibrations # 28 and 30, respectively, of Boldt et al.6a The band at 1220 cm-1 contains predominantly δ CbH (IV), γ CaN (IV) and δ CmH (δ) character. In addition to ring V, it is not surprising that the pyrroline ring IV is also affected by hydrixy-substitution at C10. Vibration #306a is assigned as γ CmC10 (V) and ð CbH (IV); its 5-7 cm-1 downshift upon hydroxylation is consistent with the electron-withdrawing nature of the C10-OH group and the possible ring distortions.
Previous work has investigated the effects of strain in ring V by a comparison of decarboxylated chlorophyll derivatives.8,20c In this case as the ring strain is relieved by pyrolysis, the bands of Cl-Fe3+-pheophorbide a at 1623 and 1494 cm-1 shift up in frequency by 2-6 cm-1 , and the 1227 cm-1 band shifts down by 8 cm-1 . The opposing shifts of the high frequency bands for the hydroxy-substitution (increasing ring strain) and pyrolysis (decreasing strain in ring V) suggest a common mechanism for these spectral effects, possibly changes in ring planarity and more importantly, because these vibrations seem to be ring V specific they are consistent with localized mode picture of Chl a.
δ-Chloro Derivative
In the localized mode model of chloro-substituted Chl a we would expect to observed a new band in the range of 650-750 cm-1, loss of δ CmH (δ), and a new band that correspond to δ CmCl (δ) in the low frequency region. Chloro-substitution at this position should also affect γ CaCm(δ) and possibly other methine bridge stretching mode, γ CaN on ring I and IV and γ CaCb(I and IV). No low frequency γ CmCl bending or stretching modes are observed (due to the quality of the spectra). The predicted Cl-sensitive vibrations in the high frequency region do compare fairly well with the assignments of the observed Ch1 a derivative band shifts. These assignments are given in Table 2. The observed core-size sensitive vibration band shift include a 7 cm-1 downshift of the 1607 cm-1 band [γ CaCm(α, β)] and a 10 cm-1 upshiftof the 1491 cm-1 band [γ CaCm(δ), γ CaCb(I)]. These large opposing frequency shifts underscore the difference in the mode character for these vibrations. Boldt et al. assign the corresponding band of the Chl a 1491 cm-1 in Ni2+ pyropheophorbide at 1552 cm-1 as γ CaCb(I), γ CaCb(III).6a Because of the strong core-size sensitivity of the 1491 cm-1 band [9-11] we suggest that this band should be more properly assigned as vibration # 13 (11) γ CaCm(δ), γ CaCb(I)]. This is also consistent with the large upshift of this band resulting from electron withdrawal from π-system by the δ-chloro substituent. Again, similar to hydroxy-substituted Chl a, the lack of an observed shift in all the high frequency core-size sensitive modes leads us to conclude that a localized vibrational mode picture is most consistent with the data.
The other vibrations affected by chloro-substitution are 1696 cm-1 γ (C9=O) in THF only 1398 cm-1 (γ CaN (IV), 1286 cm-1 [δ CmH (α, β), γ CaN (II, IV)], 1220 cm-1 [δ CbH (IV), δ CaN (IV), δ CmH (δ)] and 1182 cm-1 (γ CmC10 (V)). The atoms associated with these modes are all located in the immediate vicinity of the δ-Cl-position. Some of these vibrations , i.e., (e.g.), the ketone stretching vibration at 1700 cm-1 and the highest frequency core-size marker at 1597 cm-1, γ (CaCm), and the 1220 cm-1 band and cyclopentanone ring mode γ CmC10 at 1180 cm-1 also shift upon C-10 hydroxylation. There is obviously a change in electron density induced by Chloro-substituent that weakens the γ CaN bonds near it and γ CmC10. The upshift of the γ C9=O vibration could be caused by steric strain induced in ring V by redistribution of electron density of the halogen substituent or by a decrease in overlap of the ketone group with the aromatic π-system. There are two possible interpretations of the observed shifts; there are a) core-ruffling induced by δ-chloro-substituent and b) a redistribution of charge due to the inductive effect of the halogen atom. The disubstituted 10-OH and δ-Cl-Chl a derivatives are simply a summation of the effects and band shifts observed for the singly-substituted compounds. This provides more evidence against changes in vibrational mode composition. The opposing shifts of the high frequency core-size marker vibrations support a localized mode description of Chl a.
Epimers
The most consistent band shifts observed for the epimers of Chl a and its derivatives (Figure 5) are a 1-2 cm-1 downshifts of the 1600 cm-1 band and a 2-3 cm-1 uoshift of the 1800 cm-1 band. The less consistent, but real band shifts of this series of compounds are slight downshifts of 1707 cm-1 (γ C9=O) and 1430 cm-1 bands for the hydroxy- and δ-Cl-substituted derivatives, respectively, and a 2 cm-1 upshift of the 1220 cm-1 band. This set of vibrations has already been assigned in Table 2 with reference to 10-hydroxy substitution of Chl a. It is reasonable to assume that the addition of steric strain in ring V by epimerization should affect the same vibrations that were modified by the introduction of a OH-group at position 10. These two substitutions clearly identify the ring V sensitive vibrations of Chl a. The magnitude of the shifts is smallest for 10-OH- Chl a. The largest perturbation of ring V is most likely the OH-substitution, while a reversion in stereochemistry in this carbon atom only induces minor additional band shifts. These small band shifts also make identification of epimeric forms of Chl a by resonance Raman spectroscopy very difficult. The main identifications are 1-3 cm-1 shifts of the 1600 and 1180 cm-1 bands.
Pheophytin a
Only limited spectral information is available on pheophytins. Because some parallel vibrational shifts of hydroxy- and chloro- substituted Chl's a and pheo's a are observed, it was possible to assign some of the high frequency bands of Pheo a (Table 4). The band shifts noted upon hydroxylation that can be assigned by analogy with Chl a shifts are a 6 cm-1 upshift of the 1710 cm-1 γ C9=O band, and a 4 cm-1 downshift of ring V-specific γ CmC10(V), vibrations at 1130 cm-1. For the δ-Cl-derivative, the core-size marker bands that shift are at 1626 and 1498 cm-1. These bands shift by 19 and 14 cm-1, respectively. The exact position of the 1626 cm-1 band is less certain because of its low intensity and the presence of several shoulders in this region; however, these bands do correlate with shifts in the core sensitive vibrations of chlorinated Chl a at 1600 and 1490 cm-1. These bands are assigned as γ CaCm(α, β) and γ CaCm(δ), respectively. The band that remains constant at 1583 cm-1 may be analogous to the 1555 cm-1, band of Chl a. The 10-30 cm-1 upshift of these core-size marker bands in pheo a relative to Chl a is expected for the smaller core-size of the metal-free derivatives. The other pheophytin vibrations that correlate with the Chl a derivatives are observed at 1220 and 1130 cm-1. These bands are assigned to [δ CbH (IV), γ CaN (IV), δ CmH (δ)] and γ CmC10 (V), respectively.
CONCLUSIONS
The hydroxy- and chloro substitutions of Chl a are small enough perturbations on the chlorophyll molecule so that the observed band shifts can be interpreted within the framework of the Chl a vibrations. The vibrational shifts are indicative of a localized mode description of Chl a , as previously suggested by Boldt et al.6a The shifts caused by 10-hydroxy substitution are localized mainly within or near ring V while δ-chloro substitution results in shifts of methine bridge stretching vibrations and also bands in ring IV and V region of the molecule. The shift induced by epimerization of the Chl a derivatives at the C-10 position are the same as those observed for 10-hydroxy substitution, but smaller in magnitude. These shifted vibrations are thus at ring V sensitive vibrations. Because the band shifts are small, it is difficult to identify epimers from their RR spectra alone. This localized mode description of Chl a may be an aid in the interpretation of specific protein effects in intact protein systems.
EXPERIMENTAL
Chl a was isolated from Scenedesmus obliquus mutant C-6E according to published procedure.24 Hydroxylated chl a derivatives were prepared using the method of Pennington et al.25 or by subjecting Chl a to a separation on silica gel thin layer plates, which results in the oxidation of the chlorophylls.26 20-Cl-Chl a was prepared from Chl a by chlorination with chloroperoxidase.27
The 10-epimers of Ch1 a and δ-Cl-Ch1 a were prepared by epimerization of the parent chlorophylls in triethylamine18 and subsequent separation of the equilibrium mixture was carried out with HPLC. The hydroxylated 10S-epimers were always formed as side products in the formation of 10R-configurated epimers and were separated from the reaction mixture by TLC.26 The corresponding pheophytins were derived from the parent chlorophylls by acidification with trifluoroacetic acid (H2O/light petroleum mixture.28 All pigments were extensively purified by reverse phase HPLC, using HPLC grade solvents.
Samples for resonance Raman spectroscopy were placed in 5 mm Pyrex tube, vacuum degassed by using the freeze-pump-thaw technique, and sealed under vacuum. The setup for Raman measurements has been described elsewhere.29 For laser excitation sources a Coherent Innova 100 Kr+ and Coherent Innova 90-5 Ar+ laser were used. Typical laser powers employed were 10-15 mW and the Raman scattered light was collected in the backscattering geometry. An 1800 groove.mm-1 grating was used in the spectrograph (Spex Trilemate) stage and indene was used for frequency calibration. The integration time for each spectrum was 200 seconds. All spectra were collected for the diode array response and for background fluorescence. The reproducibility of the data in one set-up was ±1 cm-1. Between different set-up’s, i.e. involving a new calibration the reproducibility was ±5 cm-1 Only samples of one set-up were compared with each other.
Absorption Spectra: Chl a (Et2O): 661, 615, 578, 428 nm, (THF): 664, 627, 436 nm; 10-HO-Chl a (Et2O): 661, 614, 427 nm, (THF): 664, 627, 436 nm; δ-Cl-Chl a (Et2O): 666, 626, 590, 432 nm, (THF): 668, 635, 438 nm; 10-HO-δ-Cl-Chl a (Et2O): 666, 623, 585, 431 nm, (THF): 668, 631, 437 nm; Pheo a (Et2O): 667, 613, 530, 503, 410 nm; 10-HO-Pheo a (Et2O): 667, 613, 530, 503, 409 nm; δ-Cl-Pheo a (Et2O): 675, 617, 542, 512, 413 nm; 10-HO-δ-Cl-Pheo a (Et2O): 675, 616, 542, 512, 413 nm. The 10-epimers of the different Chls show the same absorption specta at the 10R-configurated parent Chls.
ACKNOWLEDGEMENTS
This work was generously supported by a Science Foundation Ireland Research Professorship award (SFI/04/RP1/B482). I am indebted to P. M. Callahan for many helpful discussions and Y. Sergeeva for assistance with the manuscript.
References
1. H. Scheer, 'Chlorophylls', CRC Press, Boca Raton, 1991.
2. M. O. Senge, J. Photochem. Photobiol. B: Biol., 1992, 16, 3. CrossRef
3. a) K. M. Barkigia, J. Fajer, and K. M. Smith, J. Am. Chem. Soc., 1981, 103, 5890; CrossRef b) M. O. Senge and K. M. Smith, Z. Kristallogr., 1992, 199, 239.
4. M. Lutz, J. Kleo, and F. Reisshusson, Biochem. Biophys. Res. Commun., 1976, 69, 711; CrossRef E. Höxtermann, W. Werneke, I. N. Standniuck, A. Lau, and P. Hoffmann, Studia Biophys., 1982, 92, 147; M. Lutz, 'Advices in Infrared and Raman Spectroscopy,' Vol. II, ed. by R. H. H. Clark and R. E. Hester, Wiley, New York, 1984, pp. 211-300; B. Robert and M. Lutz, Biochemistry, 1986, 25, 2303; CrossRef Q. Zhou, B. Robert, and M. Lutz, Biochim. Biophys. Acta, 1987, 890, 368; CrossRef D. F. Bocian, N. J. Boldt, B. W. Chadwick, and H. A. Frank, FEBS Lett., 1987, 214, 92; CrossRef M. Fujiwara, H. Hayashi, M. Tasumi, M. Kanaji, Y. Koyama, and K. Satoh, Chem. Lett., 1987, 16, 2005; CrossRef R. L. Heald, P. M. Callahan, and T. M. Cotton, J. Phys. Chem., 1988, 92, 4820; CrossRef R. Picorel, T. H. Lu, R. E. Holt, T. M. Cotton, and M. Seibert, Biochemistry, 1990, 29, 707; CrossRef T. A. Mattioli, A. Hoffmann, B. Robert, B. Schrader, and M. Lutz, Biochemistry, 1991, 30, 4648; CrossRef M. Chen, H. Zeng, A. W. D. Larkuma, and Z.-L. Cai, Spectrochim. Acta A, 2004, 60, 527. CrossRef
5. M. Fujiwara and M. J. Tasumi, J. Phys. Chem., 1986, 90, 250; CrossRef M. Fujiwara and M. J. Tasumi, J. Phys. Chem., 1986, 90, 5646. CrossRef
6. a) N. J. Boldt, R. J. Donohoe, R. R. Birge, and D. F. Bocian, J. Am. Chem. Soc., 1987, 109, 2284; CrossRef T. Sashima, M. Abe, N. Kurano, S. Miyachi, and Y. Koyama, J. Phys. Chem. B, 1998, 102, 6903; CrossRef b) H. N. Fonda, W. A. Oertling, A. Salehi, C. K. Chang, and G. T. Babcock, J. Am. Chem. Soc., 1990, 112, 9497. CrossRef
7. D. Wrobel, Biophys. Chem., 1987, 26, 91. CrossRef
8. L. A. Andersson, T. M. Loehr, T. M. Cotton, D. M. Simpson, and K. M. Smith, Biochim. Biophys. Acta, 1989, 974, 163. CrossRef
9. L. A. Andersson, T. M. Loehr, C. K. Chang, and A. G. Mauk, J. Am. Chem. Soc., 1985, 107, 182. CrossRef
10. a) Y. Ozaki, K. Iriyama, H. Ogoshi, T. Ochiai, and T. Kitagawa, J. Phys. Chem., 1986, 90, 6105; CrossRef b) Y. Ozaki, K. Iriyama, H. Ogoshi, T. Ochiai, and T. Kitagawa, J. Phys. Chem., 1986, 90, 6113; CrossRef c) O.-K. Song, J.-S. Ha, and M. Yoon, J. Raman Spectrosc., 1990, 21, 645. CrossRef
11. P. S. Woolley, B. J. Keely, and R. E. Hester, J. Chem. Soc., Perkin Trans. 2, 1997, 1731; CrossRef P. S. Woolley, A. J. Moir, and R. E. Hester, J. Chem. Soc., Perkin Trans. 2, 1998, 1833. CrossRef
12. J. J Katz, L. L. Shipman, T. M. Cotton, and T. R. Janson, 'The Porphyrins', Vol. V, ed. by D. Dolphin, Academic Press, New York, 1978, pp. 401-458.
13. T. Watanabe, M. Kobayashi, A. Hongu, M. Nakazato, T. Hiyama, and N. Murata, FEBS Lett., 1985, 191, 252. CrossRef
14. W. W. Parson, 'Photosynthesis', ed. by J. Amesz, Elsevier, New York, 1987, pp. 43-61; CrossRef T. Watanabe, M. Nakazato, H. Mazaki, A. Hongu, M. Konno, S. Saitoh, and K. Honda, Biochim. Biophys. Acta, 1985, 807, 110. CrossRef
15. M. O. Senge, M. Speck, A.Wiehe, H. Dieks, S. Aguirre, and H. Kurreck, Photochem. Photobiol., 1999, 70, 206. CrossRef
16. R. P. Grese, R. C. Cerny, M. L. Gross, and M. Senge, J. Am. Soc. Mass Spectrom., 1990, 1, 72. CrossRef
17. S. Krawczyk, Biochim. Biophys. Acta, 1989, 976, 140. CrossRef
18. P. H. Hynninen and S. Lötjonen, Synthesis, 1983, 705. CrossRef
19. J. Fajer, K. M. Barkigia, E. Fujita, D. A. Goff, L. K. Hanson, J. D. Head, T. Horning, K. M. Smith, and M. C. Zerner, 'Antennas and Reaction Centers of Photosynthetic Bacteria', ed. by M. E. Michel-Beyerle, Springer, Berlin, 1985, pp. 324-338.
20. a) M. O. Senge and K. M. Smith, Photochem. Photobiol., 1991, 54, 841; CrossRef b) M. O. Senge, W. W. Kalisch, and S. Runge, Tetrahedron, 1998, 54, 3781; CrossRef c) M. O. Senge, K. Ruhlandt-Senge, and K. M. Smith, Z. Naturforsch., 1995, 50b, 139.
21. L. D. Spaulding, C. C. Chang, N.-T. Yu, and R. H. Felton, J. Am. Chem. Soc., 1975, 97, 2517. CrossRef
22. L. L. Thomas, J.-H. Kim, and T. M. Cotton, J. Am. Chem. Soc., 1990, 112, 9378. CrossRef
23. K. N. Solovyov, L. L. Gladkov, A. T. Gradyushko, N. M. Ksenofontova, A. M. Shulga, and A. S. Starukhin, J. Mol. Struct., 1978, 45, 267. CrossRef
24. M. Senge, D. Dörnemann, and H. Senger, FEBS Lett., 1988, 234, 215. CrossRef
25. F. C. Pennington, H. H. Strain, W. A. Svec, and J. J. Katz, J. Am. Chem. Soc., 1967, 89, 3875. CrossRef
26. M. Senge, A. Struck, D. Dörnemann, H. Scheer, and H. Senger, Z. Naturforsch., 1988, 43c, 515.
27. M. Senge and H. Senger, Photochem. Photobiol., 1988, 48, 711; CrossRef M. Senge and H. Senger, Biochim. Biophys. Acta, 1989, 977, 177. CrossRef
28. S. Lötjonen and P. H. Hynninen, Synthesis, 1983, 708. CrossRef
29. P. M. Callahan and T. M. Cotton, J. Am. Chem. Soc., 1987, 109, 7001. CrossRef