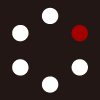
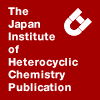
HETEROCYCLES
An International Journal for Reviews and Communications in Heterocyclic ChemistryWeb Edition ISSN: 1881-0942
Published online by The Japan Institute of Heterocyclic Chemistry
e-Journal
Full Text HTML
Received, 10th February, 2009, Accepted, 13th April, 2009, Published online, 13th April, 2009.
DOI: 10.3987/COM-09-11681
■ Reactivity of Arylnitrile Oxides and C-Aroyl-N-phenylnitrones with 3-Methylenedihydro-(3H)-furan-2-one and Itaconic Anhydride
Christophe Roussel, Kabula Ciamala,* Joël Vebrel, and Claude Riche
Institute of UTINAM UMR CNRS 6213, Faculty of Science and Technology, University of Franche-Comté, 16 Route de Gray, F-25030 Besançon, France
Abstract
1,3-dipolar cycloaddition is a powerful route for the synthesis of five-membered heterocycles. The [3+2] cycloadditions of some α-methylene-γ-buryrolactones, namely 3 methylenedihydro-(3H)-furan-2-one (1) and itaconic anhydride (2) were studied. Their reactions with arylnitrile oxides (3) and C-aroyl-N-phenylnitrones (7) proceed with complete regioselectivity. From a stereochemical point of view, the addition of arylnitrile oxides (3) leads to the unique spiroheterocycles (4-5). Actually, a single stereocenter is generated during the reaction. In the particular case of p-nitrophenylnitrile oxide (3d), only the diacidic form (10d) of the spiroheterocyle (5d) was isolated. In contrast, the addition of C-aroyl-N-phenylnitrones (7) produces a couple of diastereoisomers, since two stereocenters are generated simultaneously. Nevertheless, the reaction is regioselective and stereospecific leading also to the single spiroheterocycles (19-20). The proposed stereochemistry of spiranic compounds (19d) and (20d) has been corroborated by two single crystal X-ray crystallographic analysis.INTRODUCTION
Pharmaceutical industry is much interested in molecules presenting biological activities. Specific syntheses of α-methylene-γ-butyrolactones with cytotoxic, phytotoxic, antitumoral and bactericidal properties have been reviewed.1 In addition, simple chemical modifications performed at the methylene group have been carried out and the biological activity of the resulting compounds has been compared to that of the original molecules.2-5 For example, the transformation of dehydroleucodine into 11,13-dihydro-dehydroleucodine (=CH2→CH3) decreases cytotoxic effects without significant alteration of the original therapeutic properties.2 One of the possibilities to chemically modify the methylene group is the [3+2] cycloaddition, affording bicyclic spiranic heterocycles.6-12 As an example, cyclopropyl spiranic lactones presenting anticonvulsant properties have been prepared from the reaction of unsaturated lactones with diazomethane.11
Since several years we have focused on the reactivity of methylene-γ-butyrolactones towards several 1,3 dipoles such as nitrones,13,14 nitrile oxides15-17 and diazoalkanes.18,19 We particularly paid attention to the role played by the substituents of the dipolar entities on both chemical reactivity and stereochemistry. We have demonstrated that the reactions of linear C-N-O 1,3-dipoles such as arylnitrile oxides with achiral γ-methylene-γ-butyrolactones proceed with complete regioselectivity. The [3+2] cycloadditions lead to unique spiroheterocycles,15-17 only one stereocenter being generated from the prostereogenic carbon atom of the methylene group (Scheme 1). To the best of our knowledge, except our work,15 only two references relate the reactivity of arylnitrile oxides with simple α-methylene-γ-butyrolactones. The [3+2] cycloadditions of phenylnitrile oxide (3a) and p-tolylnitrile oxide (3b) with 3-methylenedihydro-(3H)-furan-2-one (1)20 and itaconic anhydride (2)21 have been reported (Scheme 1).
When going towards bended C-N-O 1,3-dipoles like nitrones, the reactions proceed differently as each reactant possesses a prostereogenic carbon atom. For example, the reactions of γ-methylene-γ-butyrolactones with C-aroyl-N-phenylnitrones (7) also present complete regioselectivities but lead to two diastereoisomers (8) and (9) as depicted in Scheme 2.13
In these examples, the diastereoselectivity of the reactions was explained by the different interactions occurring during the establishment of the transition states. Similar studies involving (7a) and several γ methylene-γ-butyrolactams (derived from (6)) showed the same behaviour.22 In contrast, the [3+2] cycloadditions of (7a) with various α-methylene-γ-butyrolactams (derived from (1)) were found to be stereospecific,23 pointing out the important role played by these secondary interactions.
Herein, we report a reinvestigation of the reactivity of substituted arylnitrile oxides (3a-d), bearing either electron donating or withdrawing groups, with 3-methylenedihydro-(3H)-furan-2-one (1) and itaconic anhydride (2) to complete and generalise the previously reported studies. In addition, we extended our study on the reactivity of C-aroyl-N-phenylnitrones (7) towards the dipolarophiles (1) and (2) with the following aims : (i) to figure out the factors governing the stereoselectivity of the reactions and (ii) to compare the stereochemistry of the [3+2] adducts with those resulting from the reactions of similar α methylene-γ-butyrolactams with the same 1,3-dipoles.23
RESULTS AND DISCUSSION
Cycloaddition of arylnitrile oxides
The [3+2] cycloadditions of 3-methylenedihydro-(3H)-furan-2-one (1) and itaconic anhydride (2) with arylnitrile oxides (3a-d) led to unique adducts corresponding to the expected five-membered spiroheterocyles (4-5), except for the reaction involving (2) and (3d). The reactions proceed with 100% regioselectivity and spiroheterocyles (4-5) were isolated as racemates (Scheme 3).
The regiochemistry depicted in Scheme 3 was ascertained by 13C NMR spectroscopy. The chemical shift of the spiranic carbon atom C3,5’ (~84 ppm/TMS for (4) and ~87 ppm/TMS for (5)) is in agreement with vicinity of the isoxazolinic oxygen atom, as already reported.20
The reactions were carried out at room temperature in the presence of hydroquinone as reported previously.13,14,16-19 The reaction of (1) with arylnitrile oxides (3) proceeded with good yields (except for (4d)) compared to that in refluxing benzene (71% instead of 41% for (4a)).20
The classic procedure for generating arylnitrile oxides (3) in situ from arylhydroximoyl chlorides (by addition of triethylamine)24 used for the reaction involving (1) was not suitable with itaconic anhydride (2). After addition of the base, a degradation occurred, even with DABCO or substituted pyridines (pyridine catalyzes arylnitrile oxide dimerization).25 To overcome any degradation, arylnitrile oxides (3a-d) were first generated with triethylamine in a separate flask and then added to itaconic anhydride (2). This procedure, different from the thermal generation of arylnitrile oxides from arylhydroximoyl chlorides,21 afforded also the adducts (5) in good yields (similar yield for (5a), Schemes 1 and 3), except for (5d).
Another important point concerns the reaction of (2) with p-nitrophenylnitrile oxide (3d) and especially the structure of the resulting product. Compared to the isolated adducts (5a-c), the IR analyses of the obtained product revealed the disappearance of the vibrations at ~1870 and 1790 cm-1 (C=O anhydride) and the appearance of vibrations in the range between 3305 - 2720 cm-1 (associated OH) and at 1710 cm-1 (carboxylic C=O). The 1H NMR spectrum displayed two exchangeable protons with D2O and MS analyses (EI at 70 eV) gave a molecular weight of 294 g/mol, corresponding to the diacidic form of the expected adduct (5d) (276 g/mol). Further comparisons of these spectroscopic data with those of the hydrolysis products of (5a-c), confirmed that (10d) arose from (5d) by an in situ opening of the anhydride moiety.
Cycloaddtions of C-aroyl-N-phenylnitrones
Goti and co-workers have reported the reaction of the C,N-diphenylnitrone with α-methylene-γ-butyrolactone (1) in refluxing benzene or toluene.12 The cycloaddition afforded two diastereoisomers
5 spirosubstituted isoxazolidines with high selectivity (90:10) or (80:20) respectively (Scheme 4).
The 1,3-dipolar cycloaddition of C-benzoyl-N-phenylnitrone with α-methylenelactams exclusively gave a single cycloaddition in toluene via an-endo transition state23 (Scheme 4).
For comparison, the reactions of C-aroyl-N-phenylnitrones (7a-d) with 3-methylenedihydro-(3H)-furan-2-one (1) and itaconic anhydride (2) were also investigated. Independently of the nature of the cycloaddition partners, the reactions proceeded with complete regioselectivity and stereospecificity.26,27 The spiranic adducts (19) and (20) were isolated as racemates and the stereochemical features were established by NMR and X-ray crystallographic analysis (Scheme 5).
Again, the reactions were carried out at room temperature in the presence of hydroquinone. Whereas the [3+2] cycloaddition of itaconic anhydride (2) with C-aroyl-N-phenylnitrones (7) proceeded in good yields, 3-methylenedihydro-(3H)-furan-2-one (1) remained less reactive, as already observed with ethyl diazoacetate.18,19
The regioselectivity of these [3+2] cycloadditions were accessed by 1H NMR spectroscopy. The 1H NMR spectra of (19) and (20) display an ABX system corresponding to the protons attached on the isoxazolidinic ring (H4’a, H4’b and H3’). This pattern is in favour of formation of regioisomers (19) and (20). For the hypothetical regioisomers (19’) and (20’), the proton H3’ should appear as a singlet. Further confirmation of the regiochemistry came from 13C-1H HSQC NMR. As shown in Figure 1, the spiranic carbon atom C3,5’ was easily identified at 80 ppm/TMS as it is the sole sp3 quaternary carbon atom which therefore, do not present any correlation with protons. Extended 13C NMR studies gave a chemical shift for the spiranic carbon atoms between ~82 and ~84 ppm for (19) and (20), in agreement with the proximity of the isoxazolidinic oxygen atom9,12,13 (Figure 2). In addition, 13C-1H HSQC NMR performed on spiroheterocycles (19) allows the correct attribution of the chemical shifts of C4 and C4’. Whereas these chemical shifts are rather similar, the main difference between C4 and C4’ stems from the spin multiplicity of their respective born protons. Actually, C4 bears the AB part of an ABXX’ system (H4a, H4b and H5a, H5b) and C4’, the AB part of an ABX system (H4’a, H4’b and H3’). Based on figure 1, the respective chemical shifts of C4 and C4’ were found to be ~34 and ~37 ppm/TMS respectively. In contrast, for spiroadducts (20), C4 and C4’ could be easily distinguished by 13C NMR. Since C4 is directly linked to the anhydride carbonyl, it is therefore more deshielded (C4 and C4’ at ~41.0 and ~37 ppm/TMS respectively).
From a stereochemical point of view, the reactions generate two stereocenters C3,5’ and C3’ from the two prostereogenic carbon atoms of (1-2) and (7), respectively. Indeed, two possible diastereoisomers as racemates (the two dipolarophilic faces are equivalent) could be envisaged if the stereochemistry of the nitrones (7) is conserved. As confirmed by the X-ray studies performed on both (19d) and (20d), the retention of the original relative (Z) configuration of the nitrones (7)28,29 is in favour of a concerted mechanism30 (Figure 2). Thus, according to the Woodward-Hoffmann rules,31 the stereospecificity of the reaction could be figured out from endo-C=O and exo-C=O transition states as depicted in scheme 6.12,13
Displacement ellipsoids are shown at the 20% probability level. Comparison of torsion angle values shows that the isoxazolidinic ring adopts the same half-chair conformation, in which atoms N2 and C3 are respectively deviated, by –0.347(3) and 0.293(3) Å from the mean plane of the other three atoms in (19d), by -0.288(2) and 0.329(2) Å, in (20d).
As shown in Figure 2, the anti-periplanar relationship between the dipolar and lactonic carbonyl groups is in favour of the endo-C=O approach, scheme 6. Whereas the endo-C=O transition state provides some secondary orbital interactions between the N-phenyl group of the nitrone and the carbonyl group of the lactone, the exo-C=O transition state does not afford such stabilising effects.12 Nevertheless, the difference between these two transition states could not explain the stereospecificity of the reaction. For example, the [3+2] cycloaddition of C,N-diphenylnitrone with (1), is stereoselective, the endo-C=O product being the major one.12 Indeed, as the two dipolarophiles under study lead to the same diastereoisomers, the stereospecificity of our reactions should stem from the presence of the supplementary C=O born by the C aroyl-N-phenylnitrone compared to C,N-diphenylnitrone. As shown in scheme 6, the exo-C=O transition state presents also the electrostatic repulsion of the two carbon-oxygen dipoles born by the reactants. This electrostatic repulsion could then be considered as the main factor that orientates the reaction through the generation of the endo-C=O spiroadducts (19) and (20) only.
CONCLUSION
We revisited the reactivity of arylnitrile oxides with simple α-methylene-γ-butyrolactones, using substituted 1,3-dipoles to complete and generalise the previous reported studies. The reactions involving both 3-methylene-(3H)-furan-2-one and itaconic anhydride lead to the expected spiroheterocycles, except for the reaction of itaconic anhydride with p-nitrophenylnitrile oxide which affords directly the diacidic form of the spiranic compound. Whatever the dipolarophile, the 1,3-dipolar cycloadditions are entirely regioselective, the observed regioselectivity being in total agreement with other studies reported previously. The [3+2] cycloadditions of the same dipolarophiles (1) and (2) with C-aroyl-N-phenylnitrones are again fully regioselective and stereospecific. Based on X-ray diffraction studies, the stereospecificity of the reaction was explained by the repulsion of the lactonic and dipolar oxygen atoms during the establishment of the transition state.
EXPERIMENTAL
The cycloadditions were carried out under nitrogen atmosphere using standard Schlenk techniques. TLC plates, DC-Alufolien Kieselgel 60 F254, were from Merck. Melting points were measured on an Electro¬thermal IA 9200 and are not corrected. 1H and 13C NMR spectra were recorded on Bruker AC 200 [200 MHz (1H) and 50 MHz (13C)]. HSQC NMR spectra were recorded on a Bruker Avance 300 spectrometer [300 MHz (1H) and 75 MHz (13C)]. Chemical shifts were measured relative to TMS. IR spectra were recorded on a Bio-Rad FTS-7 spectrometer. Mass spectrometry experiments were run on a NERMAG R 1010 H apparatus under electronic impact at 70 eV. Elemental analyses (C, H, N) were conducted on a Leco Elemental CHN 900; values were in satisfactory agreement with the calculated ones (0.30%).
Starting materials.
3-Methylenedihydro-(3H)-furan-2-one (1) was obtained from dihydro-(3H)-furan-2-one following the literature procedure33 (xylene was replaced by anhydrous toluene in the second step). (1) was further purified by column chromatography34 or by distillation under reduced pressure.35 Itaconic anhydride (2) was purshased from Aldrich. Arylnitrile oxides (3a-d), were obtained in situ by addition of triethylamine to arylhydroximoyl chlorides,24 synthetised according to the literature.36,37 C-Aroyl-N-phenylnitrones (7a-d) were obtained from p-substituted-2-bromoacetophenone pyridinium salts.38 p-Substituted-2-bromoacetophenone pyridinium salts were synthetised as follows: To 1 equiv. of p-substituted-2-bromo¬acetophenones dissolved in 100 mL of dry acetone was slowly added 1 equiv. of pyridine. The resulting mixture was stirred vigorously for 10 min. The formed precipitate was filtered, washed with 50 mL of dry acetone, dried and used without further purification (yields: 77%, 70%, 55%, 89% for (a-d) respectively).
Cycloaddition of 3-methylenedihydro-(3H)-furan-2-one (1) with arylnitrile oxides (3a-d)
In a Schlenk tube containing 3-methylenedihydro-(3H)-furan-2-one (1) (0.49 g, 5 mmol) and 0.05 g (0.45 mmol) of hydroquinone in anhydrous Et2O (20 mL), was added under stirring, arylhydroximoyl chlorides (7 mmol). After cooling the mixture at 0 °C, 1 mL (7 mmol) of Et3N was added dropwise and after purging with dry N2, the reaction was carried out at room temperature for 24 h. After reaction, the solution was first filtered on a glass frit to get rid of triethylammonium chloride (resulting from the dechlorination of arylhydroximoyl chlorides). The filtrate was evaporated under reduced pressure leading to a solid. The two collected solids were dissolved in 20 mL of CH2Cl2 and washed with distilled water (3×20 mL). The organic phase was dried on Na2SO4 and evaporated under reduced pressure; the crude products were purified by recrystallisation from EtOH.
3’-Phenylspiroisoxazolino[5’:3]dihydro-(3H)-furan-2-one (4a)
White solid; yield 0.76 g (71%, lit., 41%20); mp 132-134 °C (lit., 132-133 °C20); IR (KBr) 1775, 1580 cm-1; 1H NMR (CDCl3) δ/TMS 2.30-2.80 (m, 2H), 3.30-4.00 (AB, 2H J=16.9 Hz), 4.35-4.60 (m, 2H), 7.30-7.75 (m, 5H); 13C NMR (CDCl3) δ/TMS 35.1, 41.9, 65.8, 84.2, 126.7-130.5, 155.9, 173.9; Anal. Calcd for C12H11NO3: C, 66.35; H, 5.10; N, 6.45. Found: C, 66.58; H, 4.91; N, 6.57.
3’-(4-Methylphenyl)spiroisoxazolino[5’:3]dihydro-(3H)-furan-2-one (4b)
White solid; yield 0.74 g (64%, lit., 56%15); mp 128 °C (lit., 128 °C15); IR (KBr) 1775, 1580 cm-1; 1H NMR (CDCl3) δ/TMS 2.40 (s, 3H), 2.30-2.80 (m, 2H), 3.30-4.00 (AB, 2H, J=16.8 Hz), 4.30-4.60 (m, 2H), 7.20 (d, 2H, J=8.0 Hz), 7.55 (d, 2H, J=8.0 Hz); 13C NMR (CDCl3) δ/TMS 21.3, 35.0, 42.0, 65.8, 84.0, 125.3-140.8, 155.8, 174.0; Anal. Calcd for C13H13NO3: C, 67.52; H, 5.67; N, 6.06. Found: C, 67.69; H, 5.61; N, 6.12.
3’-(4-Methoxyphenyl)spiroisoxazolino[5’:3]dihydro-(3H)-furan-2-one (4c)
Colorless solid; yield 0.62 g (50%); mp 137-139 °C; IR (KBr) 1775, 1600 cm-1; 1H NMR (CDCl3) δ/TMS
2.30-2.80 (m, 2H), 3.85 (s, 3H), 3.25-4.00 (AB, 2H, J=16.8 Hz), 4.35-4.60 (m, 2H), 6.95 (d, 2H, J=8.8 Hz), 7.60 (d, 2H, J=8.8 Hz); 13C NMR (CDCl3) δ/TMS 35.0, 42.1, 55.2, 65.8, 83.9, 114.0-161.3, 155.5, 174.1; Anal. Calcd for C13H13NO4: C, 63.15; H, 5.30; N, 5.67. Found: C, 62.89; H, 5.41; N, 5.79.
3’-(4-Nitrophenyl)spiroisoxazolino[5’:3]dihydro-(3H)-furan-2-one (4d)
Yellow solid; yield 0.34 g (26%); mp 208-209 °C, IR (KBr) 1785, 1610 cm-1, 1H NMR (acetone-d6) δ/TMS 2.60-2.90 (m, 2H), 3.70-4.10 (AB, 2H, J=17.5 Hz), 4.40-4.70 (m, 2H), 8.00 (d, 2H, J=8.8 Hz), 8.35 (d, 2H, J=8.8 Hz); 13C NMR (acetone-d6) δ/TMS 35.2, 42.0, 66.8, 86.8, 124.8-135.9, 156.3, 174.6; Anal. Calcd for C12H10N2O5: C, 54.97; H, 3.84; N, 10.68. Found: C, 55.23; H, 3.89; N, 10.53.
Cycloaddition of itaconic anhydride (2) with arylnitrile oxides (3a-d)
To a magnetically stirred solution of 3.5 mmol of arylhydroximoyl chlorides in 15 mL of EtOAc at 0 °C,
was added 0.4 mL of Et3N (2.9 mmol). After 5 min, the solution was added dropwise through a glass fritted disk (to get ride of the formed Et3NHCl) to a solid mixture of 0.28 g (2.5 mmol) of itaconic anhydride (2) and 0.05 g (0.45 mmol) of hydroquinone contained in a Schlenk tube cooled at –15 °C. The glass fritted disk was rinsed with 5 mL of EtOAc. After purging with nitrogen, the reaction was stirred at room temperature for 24 h. After reaction, the solvent was evaporated under reduced pressure; the crude products were purified by recrystallisation from CH2Cl2-Et2O (1:1).
3’-Phenylspiroisoxazolino[5’:3]succinic anhydride (5a)
Colorless solid; yield 0.42 g (73%, lit., 75%21); mp 146-148 °C (lit., 147-149 °C21); IR (KBr) 1865, 1800, 1605 cm-1; 1H NMR (acetone-d6) δ/TMS 3.45-3.90 (AB, 2H, J=19.2 Hz), 3.90-4.30 (AB, 2H, J=17.9 Hz), 7.45-7.85 (m, 5H); 13C NMR (acetone-d6) δ/TMS 42.5, 45.0, 87.5, 128.5-132.3, 158.3, 169.4, 172.9; Anal. Calcd for C12H9NO4: C, 62.34; H, 3.92; N, 6.06. Found: C, 62.48; H, 4.19; N, 5.81.
3’-(4-Methylphenyl)spiroisoxazolino[5’:3]succinic anhydride (5b)
Colorless solid; yield 0.39 g (64%); mp 170-172 °C; IR (KBr) 1870, 1785, 1600 cm-1; 1H NMR (acetone-d6) δ/TMS 2.40 (s, 3H), 3.45-3.85 (AB, 2H, J=19.1 Hz), 3.85-4.25 (AB, 2H, J=17.8 Hz), 7.35 (d, 2H, J= 7.9 Hz), 7.65 (d, 2H, J=7.9 Hz); 13C NMR (acetone-d6) δ/TMS 22.1, 42.5, 45.1, 87.3, 127.1-142.6, 158.2, 169.4, 172.9; Anal. Calcd for C13H11NO4: C, 63.67; H, 4.52; N, 5.71. Found: C, 63.60; H, 4.61; N, 5.69.
3’-(4-Methoxyphenyl)spiroisoxazolino[5’:3]succinic anhydride (5c)
White solid; yield 0.39 g (60%); mp 169-171 °C; IR (KBr) 1875, 1795, 1605 cm-1; 1H NMR (acetone-d6) δ/TMS 3.35-3.80 (AB, 2H, J=19.0 Hz), 3.85 (s, 3H), 3.80-4.20 (AB, 2H, J=18.0 Hz), 7.00 (d, 2H, J= 8.9 Hz), 7.70 (d, 2H, J= 8.9 Hz); 13C NMR (acetone-d6) δ/TMS 42.5, 45.2, 56.5, 87.2, 115.8-163.3, 157.8, 169.5, 173.0; Anal. Calcd for C13H11NO5: C, 59.77; H, 4.24; N, 5.36. Found: C, 59.94; H, 4.19; N, 5.42.
Alkaline treatment of 3’-arylspiroisoxazolino[5’:3]succinic anhydride (5a-c)
To a solution of spiroadducts 5a-c (0.25 mmol) in 4.1 mL of EtOH was added 0.9 mL of NaOH (2 M). The mixture was refluxed for 30 min, poured into an ice-water mixture (100 mL) and acidified with hydrochloric acid (or sulfuric acid). After extraction with CH2Cl2 (20 mL), the organic phase was washed with distilled water (2×20 mL), dried (Na2SO4) and evaporated in vacuo. The crude products were purified by recrystallization from distilled water.
Isoxazolino-3’-phenyl-5’-carboxy-5’-ethanoic acid (10a)
Beige solid; yield 0.057 g (92%); mp 206-207 °C (lit., 197 °C21); IR (KBr) 2720-3285, 1705, 1605 cm-1; 1H NMR (acetone-d6) δ/TMS 3.00-3.40 (AB, 2H, J=16.7 Hz), 3.50-4.20 (AB, 2H, J=17.2 Hz), 7.30-7.90 (m, 5H), 9.30 (s, 2H); 13C NMR (acetone-d6) δ/TMS 41.0, 44.0, 86.6, 127.5-131.0, 157.4, 171.2, 171.3; Anal. Calcd for C12H11NO5: C, 57.83; H, 4.45; N, 5.62. Found: C, 57.99; H, 4.33; N, 5.73.
Isoxazolino-3’-(4-methylphenyl)-5’-carboxy-5’-ethanoic acid (10b)
Beige solid; yield 0.062 g (94%); mp 192-194 °C; IR (KBr) 2810-3280, 1705, 1610 cm-1; 1H NMR (acetone-d6) δ/TMS 2.40 (s, 3H), 3.00-3.40 (AB, 2H, J=16.8 Hz), 3.50-4.20 (AB, 2H, J=17.5 Hz), 7.30 (d, 2H, J= 8.0 Hz), 7.65 (d, 2H, J= 8.0 Hz), 8.35 (s, 2H); 13C NMR (acetone-d6) δ/TMS 21.3, 41.0, 44.1, 86.4, 127.5-141.2, 157.3, 171.2, 171.3; HRMS (EI, 70 eV) m/z 263 (I= 0.50%) M+• , 245 (I= 4.24%) M+•-(H2O), 218 (I= 0.50) M+• -(COOH), 201 (I= 4.40%) M+•-(COOH) -(OH), 160 (I= 100%) M+•-(COOH) -(OH) -(CH=C=O), 131 (I= 26,03%) M+• -(COOH) -(OH) -(CH=C=O) -(CHO) a; Anal. Calcd for C13H13NO5: C, 59.31; H, 4.98; N, 5.32. Found: C, 59.16; H, 5.07; N, 5.39.
a) determined according to the literature 39
Isoxazolino-3’-(4-methoxyphenyl)-5’-carboxy-5’-ethanoic acid (10c)
Beige solid; yield 0.065 g (93%); mp 199-200 °C; IR (KBr) 2760-3280, 1705, 1605 cm-1; 1H NMR (acetone-d6) δ/TMS 3.00-3.40 (AB, 2H, J=16.9 Hz), 3.85 (s, 3H), 3.50-4.20 (AB, 2H, J=17.4 Hz), 7.00 (d, 2H, J= 8.8 Hz), 7.70 (d, 2H, J= 8.8 Hz), 9.10 (s, 2H); 13C NMR (acetone-d6) δ/TMS 41.0, 44.2, 55.7, 86.2, 114.9-162.1, 156.9, 171.2, 171.4; Anal. Calcd for C13H13NO6: C, 55.91; H, 4.69; N, 5.02. Found: C, 56.10; H, 4.71; N, 4.97.
Isoxazolino-3’-(4-nitrophenyl)-5’-carboxy-5’-ethanoic acid (10d) b
Yellow solid; yield 0.15 g (20%); mp 110-112 °C; IR (KBr) 2720-3305, 1710, 1605 cm-1; 1H NMR (acetone-d6) δ/TMS 3.10-3.45 (AB, 2H, J=16.9 Hz), 3.70-4.30 (AB, 2H, J=17.5 Hz), 8.05 (d, 2H, J= 8.9 Hz), 8.40 (d, 2H, J= 8.9 Hz); 13C NMR (acetone-d6) δ/TMS 41.7, 44.2, 88.5, 123.6-136.7, 157.3, 171.9; Anal. Calcd for C12H10N2O7: C, 48.99; H, 3.43; N, 9.52. Found: C, 49.26; H, 3.49; N, 9.46.
b) by in situ ring opening during cycloaddition
Cycloadditions of 3-methylenedihydro-(3H)-furan-2-one (1) and itaconic anhydride (2) with C-aroyl-N-phenylnitrones (7a-d)
In a Schlenk tube were added 5 mmol of methylene-lactone (1-2), 5 mmol of C-aroyl-N-phenylnitrones (7a-d), 0.05 g (0.45 mmol) of hydroquinone and 20 mL of EtOAc. The mixture was stirred at room temperature for 24 h under nitrogen atmosphere and the solvent removed under reduced pressure leading to a crude oil. For spiroheterocyles (19), EtOH (20 mL) was added to the crude oil and the mixture subjected to ultrasonication. The resulting solids were recrystallised from EtOH. For spiroheterocycles (20), Et2O (20 mL) was added to the crude oil and the mixture was subjected to ultrasonication. The resulting solids were recrystallised from a mixture of CH2Cl2-Et2O (1:1).
3’-Benzoyl-2’-phenyl-2’,3’-dihydrospiroisoxazolino[5’:3]dihydro-(3H)-furan-2-one (19a)
Beige solid; yield 0.70 g (43%); mp 159-160 °C; IR (KBr) 1775, 1685 cm-1; 1H NMR (CDCl3) δ/TMS 2.40-2.75 (m, 2H), 2.85-3.10 (AB part of an ABX, 2H, JAX=4.2, JBX=7.5, JAB=12.4 Hz), 4.30-4.55 (m, 2H), 5.55 (X part of an ABX, 1H, JAX=4.2, JBX=7.5), 6.95-8.20 (m, 10H); 13C NMR (CDCl3) δ/TMS 34.4, 37.2, 65.9, 69.0, 82.5, 115.1-148.9, 174.6, 195.3; Anal. Calcd for C19H17NO4: C, 70.58; H, 5.30; N, 4.33. Found: C, 70.87; H, 5.41; N, 4.34.
3’-(4-Methylbenzoyl)-2’-phenyl-2’,3’-dihydrospiroisoxazolino[5’:3]dihydro-(3H)-furan-2-one (19b)
Beige solid; yield 0.52 g (31%); mp 142-143 °C; IR (KBr) 1775, 1680 cm-1; 1H NMR (CDCl3) δ/TMS 2.40-2.75 (m, 2H), 2.45 (s, 3H), 2.80-3.10 (AB part of an ABX, 2H, JAX=4.4, JBX=7.5, JAB=12.5 Hz), 4.30-4.55 (m, 2H), 5.50 (X part of an ABX, 1H, JAX=4.4, JBX=7.5), 6.95-8.00 (m, 9H); 13C NMR (CDCl3) δ/TMS 21.4, 34.0, 37.4, 65.7, 68.8, 82.3, 114.9-149.0, 174.6, 194.8; Anal. Calcd for C20H19NO4: C, 71.20; H, 5.68; N, 4.15. Found: C, 70.97; H, 5.76; N, 4.21.
3’-(4-Methoxybenzoyl)-2’-phenyl-2’,3’-dihydrospiroisoxazolino[5’:3]dihydro-(3H)-furan-2-one (19c)
Colorless solid; yield 0.58 g (33%); mp 136-138 °C; IR (KBr) 1775, 1680 cm-1; 1H NMR (CDCl3) δ/TMS 2.35-2.75 (m, 2H), 2.85-3.05 (AB part of an ABX, 2H, JAX=4.5, JBX=7.0, JAB=12.4 Hz), 3.95 (s, 3H), 4.30-4.55 (m, 2H), 5.45 (X part of an ABX, 1H, JAX=4.5, JBX=7.0), 6.90-8.15 (m, 9H); 13C NMR (CDCl3) δ/TMS 34.3, 37.2, 56.4, 65.9, 69.0, 82.6, 110.9-160.0, 174.7, 193.0; Anal. Calcd for C20H19NO5: C, 67.98; H, 5.42; N, 3.96. Found: C, 68.13; H, 5.51; N, 3.89.
3’-(4-Nitrobenzoyl)-2’-phenyl-2’,3’-dihydrospiroisoxazolino[5’:3]dihydro-(3H)-furan-2-one (19d)
Yellow solid; yield 0.77 g (42%); mp 180-181 °C, IR (KBr) 1775, 1700 cm-1, 1H NMR (acetone-d6) δ/TMS 2.40-2.80 (m, 2H), 2.80-3.20 (AB part of an ABX, 2H, JAX=7.1, JBX=2.9, JAB=12.7 Hz), 4.30-4.55 (m, 2H), 5.95 (X part of an ABX, 1H, JAX=7.1, JBX=2.9), 6.90-8.50 (m, 9H); 13C NMR (acetone-d6) δ/TMS 35.1, 37.0, 66.6, 70.3, 84.2, 115.7-151.2, 175.4, 196.6; Anal. Calcd for C19H16N2O6: C, 61.95; H, 4.38; N, 7.61. Found: C, 62.14; H, 4.33; N, 7.67.
3’-Benzoyl-2’-phenyl-2’,3’-dihydrospiroisoxazolino[5’:3]succinic anhydride (20a)
White solid; yield 1.55 g (92%); mp 135-136 °C; IR (KBr) 1875, 1795, 1680 cm-1; 1H NMR (CDCl3) δ/TMS 2.95-3.20 (AB part of an ABX, 2H, JAX=6.7, JBX=2.6, JAB=12.7 Hz), 3.20-3.55 (AB, 2H, JAB=19.6 Hz), 5.65 (X part of an ABX, 1H, JAX=6.7, JBX=2.6), 7.00-8.10 (m, 10H); 13C NMR (CDCl3) δ/TMS 37.6, 41.0, 68.1, 84.4, 115.4-147.7, 166.7, 170.7, 194.4; Anal. Calcd for C19H15NO5: C, 67.65; H, 4.48; N, 4.15. Found: C, 67.93; H, 4.52; N, 4.13.
3’-(4-Methylbenzoyl)-2’-phenyl-2’,3’-dihydrospiroisoxazolino[5’:3]succinic anhydride (20b)
White solid; yield 1.36 g (77%); mp 133-134 °C; IR (KBr) 1875, 1790, 1680 cm-1; 1H NMR (CDCl3) δ/TMS 2.45 (s, 3H), 2.95-3.15 (AB part of an ABX, 2H, JAX=6.5, JBX=2.7, JAB=12.8 Hz), 3.15-3.55 (AB, 2H, JAB=19.5 Hz), 5.65 (X part of an ABX, 1H, JAX=6.5, JBX=2.7), 7.00-8.00 (m, 9H); 13C NMR (CDCl3) δ/TMS 21.6, 37.8, 40.9, 68.1, 84.3, 115.4-147.8, 166.9, 170.9, 194.1; Anal. Calcd for C20H17NO5: C, 68.37; H, 4.88; N, 3.99. Found: C, 68.59; H, 4.97; N, 4.03.
3’-(4-Methoxybenzoyl)-2’-phenyl-2’,3’-dihydrospiroisoxazolino[5’:3]succinic anhydride (20c)
White solid; yield 1.45 g (79%); mp 132-133 °C; IR (KBr) 1880, 1805, 1680 cm-1; 1H NMR (CDCl3) δ/TMS 2.95-3.20 (AB part of an ABX, 2H, JAX=6.8, JBX=2.5, JAB=12.5 Hz), 3.20-3.55 (AB, 2H, JAB=19.5 Hz), 3.90 (s, 3H), 5.60 (X part of an ABX, 1H, JAX=6.8, JBX=2.5), 6.80-8.10 (m, 9H); 13C NMR (CDCl3) δ/TMS 37.8, 40.9, 55.4, 67.9, 84.3, 113.9-164.1, 166.9, 170.9, 192.8; Anal. Calcd for C20H17NO6: C, 65.39; H, 4.66; N, 3.81. Found: C, 65.15; H, 4.81; N, 4.01.
3’-(4-Nitrobenzoyl)-2’-phenyl-2’,3’-dihydrospiroisoxazolino[5’:3]succinic anhydride (20d)
Yellow solid; yield 1.71 g (90%); mp 148-150 °C; IR (KBr) 1875, 1790, 1680 cm-1; 1H NMR (CDCl3) δ/TMS 2.95-3.20 (AB part of an ABX, 2H, JAX=6.7, JBX=1.6, JAB=12.8 Hz), 3.15-3.55 (AB, 2H, JAB=19.5 Hz), 5.70 (X part of an ABX, 1H, JAX=6.7, JBX=1.6), 7.00-8.40 (m, 9H); 13C NMR (CDCl3) δ/TMS 37.2, 41.0, 68.7, 84.7, 115.7-147.5, 166.2, 170.4, 193.6; Anal. Calcd for C19H14N2O7: C, 59.69; H, 3.69; N, 7.33. Found: C, 59.42; H, 3.81; N, 7.16.
X-Ray crystal structure analyses of compounds (19d) and (20d)
For both structures, data were measured from very small prismatic crystals with a Nonius Kappa-CCD area-detector diffractometer, using graphite monochromated Mo-Kα radiation, according to the phi and omega scan method, up to θ = 19° for (19d) and 22° for (20d).40 The structures were solved with program SHELXS8641 and refined by full-matrix least-squares, based upon all unique F2 with program SHELXL97.42 The hydrogen atoms were fitted at theoretical positions and treated as riding, assigned of an isotropic displacement parameter equivalent to 1.10 the one of the bonded atom. CCDC 664441 & 664442 contain the supplementary crystallographic data for (19d) and (20d) respectively. These data can be obtained free of charge from the Cambridge Crystallographic Data Center via www.ccdc.cam.ac.uk/data_request/cif.
ACKNOWLEGDEMENTS
We thank Gregorio Crini (CERAC, University of Franche-Comté) for NMR measurements, E. Pousson (University of Bourgogne) for performing the elemental analyses, and Angèle Chiaroni (Laboratory of Crystallochemistry, Institute of Chemistry of Natural Products) for fruitful collaboration on X-ray studies. Sandrine Gerber (Laboratory of Glycochemistry and Asymetric Synthesis, Institute of Chemical Science and Engineering) is also acknowledged for fruitful discussion. This work was supported by the French Ministry of Research.
References
1. H. M. R. Hoffmann and J. Rabe, Angew. Chem., Int. Ed. Engl., 1985, 24, 94. CrossRef
2. L. M. Polo, C. M. Castro, M. C. Cruzado, C. J. G. Collino, F. D. Cuello-Carrion, D. R. Ciocca, O. S. Giordano, M. Ferrari, and L.A. Lopez, Eur. J. Pharmacology, 2007, 556, 19. CrossRef
3. W. Adam and V. O. Navas-algado, J. Org. Chem., 1995, 60, 578. CrossRef
4. S. M. Kupchan, D. C. Fessler, M. A. Eakin, and T. J. Giacobbe, Science, 1970, 168, 376. CrossRef
5. D. Hwang, N. H. Fischer, B. C. Jang, H. Y. Tak, J. K. Kim, and W. Lee, Biochem. Biophys. Res. Commun., 1996, 226, 810. CrossRef
6. Synthetic Applications of 1,3-Dipolar Cycloaddition Chemistry Toward Heterocycles and Natural Products, ed. by A. Padwa and W. H. Pearson, John Wiley and Sons, 2002.
7. P. de March, M. el Arrad, M. Figueredo, and J. Font, Tetrahedron, 1998, 54, 11613. CrossRef
8. R. Grigg, V. Savic, and M. Thornton-Pett, Tetrahedron, 1997, 53, 10633. CrossRef
9. D. Alonso-Perarnau, P. de March, M. el Arrad, M. Figueredo, J. Font, and T. Parella, Tetrahedron, 1997, 53, 14763. CrossRef
10. P. Micuch, L. Fisera, V. Ondrus, and P. Ertl, Molecules, 1997, 2, 57. CrossRef
11. E. M. Peterson, K. Xu, K. D. Holland, A. C. McKeon, S. M. Rothman, J. A. Ferrendelli, and D. F. Covey, J. Med. Chem., 1994, 37, 275. CrossRef
12. M. Cacciarini, F. M. Cordero, C. Faggi, and A. Goti, Molecules, 2000, 5, 637. CrossRef
13. C. Roussel, R. Fihi, K. Ciamala, J. Vebrel, T. Zair, and C. Riche, Org. Biomol. Chem., 2003, 1, 2689. CrossRef
14. J. C. Daran, R. Fihi, C. Roussel, N. Laghrib, M. Azrour, K. Ciamala, and J. Vebrel, Acta Cryst., Sect. E, 2006, 62, O329. CrossRef
15. R. Fihi, K. Ciamala, J. Vebrel, and N. Rodier, Bull. Soc. Chim. Belg., 1995, 104, 55. CrossRef
16. C. Roussel, K. Ciamala, P. Audebert, and J. Vebrel, New J. Chem.,1999, 23, 989. CrossRef
17. C. Roussel, R. Fihi, K. Ciamala, P. Audebert, and J. Vebrel, New J. Chem., 2000, 24, 471. CrossRef
18. C. Roussel, K. Ciamala, J. M. Melot, J. Vebrel, and C. Riche, J. Chem. Res. (S), 2002, 449. CrossRef
19. C. Roussel, K. Ciamala, J. Vebrel, M. Knorr, and M. M. Kubicki, Heterocycles, 2007, 71, 1517. CrossRef
20. S. Stverkova, Z. Zak, and J. Jonas, Liebigs Ann., 1995, 477. CrossRef
21. A. Quilico and P. Grünanger, Gazz. Chim. Ital., 1952, 82, 140.
22. S. Rigolet, P. Goncalo, J. M. Melot, and J. Vebrel, J. Chem. Res.(S), 1998, 686. CrossRef
23. S. Rigolet, J. M. Melot, J. Vebrel, A. Chiaroni, and C. Riche, J. Chem. Soc., Perkin Trans. 1, 2000, 1095. CrossRef
24. R. Huisgen and W. Mack, Tetrahedron Lett., 1961, 583. CrossRef
25. F. Desarlo, J. Chem. Soc., Perkin Trans. 1, 1974, 1951. CrossRef
26. S. W. Baldwin and A. Long, Org. Lett., 2004, 6, 1653. CrossRef
27. O. Tamura, T. Shiro, M. Ogasawara, A. Toyao, and H. Ishibashi, J. Org. Chem., 2005, 70, 4569. CrossRef
28. W. B. Jennings, D. R. Boyd, and L. C. Waring, J. Chem. Soc., Perkin Trans. 2, 1976, 610. CrossRef
29. M. Joucla, D. Gree, and J. Hamelin, Tetrahedron, 1973, 29, 2315. CrossRef
30. S. Chandrasekhar, M. Ravindranath, B. S. Neela, S. Ramakumar, and M. A. Viswamitra, J. Chem. Res. (S), 1989, 252.
31. R. B. Woodward and R. Hoffmann, The Conservation of Orbital Symmetry, Verlag Chemie Gmbh, 1971.
32. A. L. Spek, J. Appl. Cryst., 2003, 36, 7. CrossRef
33. M. Ueda, M. Takahashi, T. Suzuki, Y. Imai, and C. U. Pittman, J. Polym. Science Part a-Polymer Chemistry, 1983, 21, 1139. CrossRef
34. C. R. Hutchinson, J. Org. Chem., 1974, 39, 1854. CrossRef
35. P. A. Grieco and C. S. Pogonowski, J. Org. Chem., 1974, 39, 1958. CrossRef
36. K. C. Liu, B. R. Shelton, and R. K. Howe, J. Org. Chem.,1980, 45, 3916. CrossRef
37. C. Grundmann and R. Richter, J. Org. Chem., 1967, 32, 2308. CrossRef
38. F. Krönhke and E. Börner, Chem. Ber., 1936, 69, 2006. CrossRef
39. A. R. Katritzky and C. W. Rees, Comprehensive Heterocyclic Chemistry : the structure, reaction, synthesis and uses of heterocyclic compounds; Oxford a.o. : Pergamon Press, 1984 ed., 1984; Vol. 4. CrossRef
40. Collect (Brucker AXS BV) 1997-2000; HKL Denzo and Scalepack (Otinowski & Minor, 1997).
41. G. M. Sheldrick, Acta Cryst., Sect. A, 1990, 46, 467. CrossRef
42. G. M. Sheldrick, SHELXL97. Program for the refinement of Crystal structures, Univ. of Göttingen, Germany, 1997