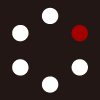
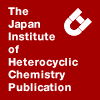
HETEROCYCLES
An International Journal for Reviews and Communications in Heterocyclic ChemistryWeb Edition ISSN: 1881-0942
Published online by The Japan Institute of Heterocyclic Chemistry
e-Journal
Full Text HTML
Received, 1st March, 2009, Accepted, 9th April, 2009, Published online, 10th April, 2009.
DOI: 10.3987/COM-09-11695
■ A Convenient Method for the Synthesis of 2-Oxazolidinones from Ethylene Carbonate and Primary Aryl Amines
Hang Gong and Nian-fa Yang*
Key Laboratory of Environment-Friendly Chemistry and Applications of Ministry of Education, College of Chemistry, Xiangtan University, Hunan, Xiangtan 411105, China
Abstract
One-step procedure for the synthesis of 2-oxazolidinones from ethylene carbonate and primary aryl amines in the presence of amine catalysts was described. Various reaction parameters including catalyst, temperature, reaction time, solvent and the amount of catalyst were investigated. Excellent results were obtained using DBU as catalyst at 100 °C within 2 h free of solvent.2-Oxazolidinones have been extensively used in organic synthesis. They are, in fact, employed as protecting groups in organic synthesis, chiral auxiliaries (Evans’ chiral auxiliaries) in asymmetric synthesis and as biologically active pharmaceutical agents and so on.1-7
Conventionally 2-oxazolidinones are synthesized by reactions of β-aminoalcohols or other β-amino compounds with toxic phosgene or its derivative,8-9 which may cause serious environmental pollution and equipment corrosion. Therefore, a number of non-toxic procedures have been suggested: (1) oxidative carbonylation using CO/O2 and β-aminoalcohols, (2) they are prepared by dialkyl carbonates and β-aminoalcohols, (3) direct synthesis of 2-oxazolidinones from carbon dioxide and β-aminoalcohols or aziridines.
The intramolecular oxidative cyclocarbonylation of β-aminoalcohols catalyzed by transition metals is a way to produce these heterocyclic compounds,10-11 but the oxidative carbonylation is expensive in using carbon monoxide and it has potential explosion hazards. As an alternative, dialkyl carbonates were used for the synthesis of 2-oxazolidinones.12-14 It should be noted that dimethyl carbonate is currently produced by phosgenation and oxidative carbonylation routes.15-16 There are several reports on the direct synthesis of 2-oxazolidinones by carbon dioxide and β-aminoalcohols or aziridines,17-19 however, toxic catalysts and high pressure and/or high temperature are required in most of those work. Development of a simple, highly efficient and environmentally benign method for synthesis of 2-oxazolidinone derivatives is still highly desired.
Recently, the synthesis of 2-oxazolidinones from ethylene carbonate (EC) and β-aminoalcohols in the presence of homogenous base catalyst20 or heterogeneous base catalyst21 was reported. The reaction could be considered as a carbon dioxide fixation reaction, since EC is synthesized via reaction of ethylene oxide with carbon dioxide.22-27
However, there are very few reports on the direct conversion of simple primary amine into 2-oxazolidinones. S. Tanimori and M. Kirihata reported that the reaction of dimethyl (Z)-2-butenylene dicarbonate with primary amines in the presence of [Pd(η3-C3H5)Cl]2 and 1,1’-bis(diphenylphosphino) ferrocene (dppf) produced vinyl-oxazolidone compounds in 70% yield28 (Scheme 1).
Herein, we report a novel method for synthesis of 2-oxazolidinones from five-membered cyclic carbonates EC and primary aryl amines under mild conditions (Scheme 2). This synthetic method has several advantages: (1) the reaction can be considered as a carbon dioxide fixation reaction, since five-membered cyclic carbonates are synthesized via reaction of ethylene oxide with carbon dioxide; (2) the 2-oxazolidinones were obtained under mild conditions that are neither dangerous nor noxious; (3) this reaction was wasteless and pollution-free, water was the sole co-product; (4) the readily available aryl amines was used as substrate. So, this reaction process was environmentally benign and high atom economic.
The reaction of aniline and ethylene carbonate was investigated as a model. And the results are summarized in Tabel 1. The reaction could not occurred if the catalyst was absent (Table 1, entry 1). In this reaction, the yield of 2-oxazolidinone was greatly affected by basic catalyst (Table 1, entries 2–7). When DBU was used as catalyst, the excellent yield (91%) of 2-oxazolidinone was obtained (Table 1, entry 7). It is due to the stability of quaternary ammonium salt through conjugation, which increases its equilibrium concentration (Scheme 3). In order to establish the optimized reaction conditions, we examined the effect of temperature, reaction time, the amount of the catalyst and different catalysts. Slightly high temperature was necessary for this reaction. If the temperature was too low, the reaction does not proceed normally. The preferred temperature was 100 oC in our experiments (Table 1, entries 7-8). The yield was affected by the reaction time, and increasing reaction time was propitious to improve the yield of 2-oxazolidinone, yet the reaction almost completed within 2 h (Table 1, entries 7, 12–13). A higher yield was obtained by increasing the amount of the catalyst (Table 1, entries 7, 9-11). The yield of 2-oxazolidinone was affected by the scale of the substrates, the excessive of ethylene carbonate was required, perhaps it is caused by the decomposability of ethylene carbonate in the presence of the catalyst (Table 1, entries 7, 14-16). Another problem need to point out is that the reaction should proceed under the inert atmosphere, such as argon, to avoid the oxidation of aniline.
Under the optimized reaction conditions (the molar ratio of substrates and catalyst, aniline:EC:DBU = 1:5:1; temperature, 100 oC, reaction time, 2 h), it was found that this method was applicable to the synthesis of corresponding 2-oxazolidinones from different aryl amines and ethylene carbonate. The results are summarized in Table 2. It was found that the aryl amines bearing electron-withdrawing groups or none-substituent at the para- or meta-position on the benzene rings gave the corresponding 2-oxazolidinones with high yields (Table 2, entries 1-2, 4-5). Other aryl amines with electron-donating substituents at the para- or meta-position on the benzene rings provide middle to good yields (Table 2, entries 8-10). Especially, if there is a substituent at the ortho-position of the benzene ring of the aryl amine, the reaction yields will be much lower (Table 2, entries 3, 6-7, 11). Perhaps it is caused by the steric effect of the orth-substituent. Yet the 2,4,6-trichloroaniline gives a middle yield. Maybe it is caused by the steric effect and electronic effect (Table 2, entry 12).
Proposed mechanism for the reaction of ethylene carbonate with aniline catalyzed by amine catalyst displays in Scheme 3, which is similar to the the indole benzylation catalyzed by the amine catalyst.29 On the Cycle I, the amine catalyst acts as nucleophilic catalyst, which acts as a base on the Cycle II.
In summary, we have found that the synthesis of 2-oxazolidinones from ethylene carbonate and corresponding primary aryl amines could be performed with excellent yields by using DBU as catalyst under mild conditions. This atom economical methodology represents a valuable and environmentally benign non-phosgene alternative to the use of toxic phosgene or expensive dimethyl carbonate. Since ethylene carbonate was prepared from epoxide and carbon dioxide, the title reactions will be chemical fixation of carbon dioxide to important chemicals indirectly.
EXPERIMENTAL
General remarks
All glassware was dried in oven and cooled in a desiccator (silica gel desiccant) prior to use. Liquid amine were distilled and stored under a nitrogen atmosphere before being used. Other commercially supplied reagents were used without further purification. All the products were characterized by 1H NMR, 13C NMR and elemental analysis.
General procedure for the synthesis of 2-oxazolidinones (Table 2)
For each reaction, aryl amine (1 mmol), EC (5 mmol) and DBU (1 mmol) were charged into a 5 mL round-bottomed flask equipped with a magnetic stirrer in argon atmosphere at 100 oC for 2 h. At the end of the reaction, after the mixture was cooled back to rt, the mixture was diluted by 10 mL water. Then solid or oil was found in the mixture. The solid was filtered in vacuo and washed with water (5 mL×2), then dried in vacuo to obtain the purified product. In the circumstance of oil, the reaction mixture was extracted with CH2Cl2 (5 mL×3) and the crude product was obtained after evaporation of solvent. The crude product was purified by preparative TLC (silica gel, CH2Cl2) to give the fine product. All the products were characterized by 1H NMR, 13C NMR and elemental analyses.
Characterization of the oxazolidinone derivatives
Compound 1a 1H NMR (CDCl3, 400 MHz, TMS): δ 4.08 (2H, t, J = 8.0 Hz, -CH2-), 4.50 (2H, t, J = 8.0 Hz, -CH2-), 7.15 (1H, t, J = 7.4 Hz, Ar), 7.39 (2H, dd, J = 7.8, 8.1 Hz, Ar), 7.55 (2H, d, J = 8.0 Hz, Ar); 13C NMR (CDCl3, 60 MHz, TMS): δ 45.23, 61.31, 118.30, 124.10, 129.07, 138.32, 155.30; Anal. Calcd for C9H9NO2: C, 66.25; H, 5.56; N, 8.58. Found: C, 66.16; H, 5.56; N, 8.75.
Compound 2a 1H NMR (CDCl3, 400 MHz, TMS): δ 4.15 (2H, t, J = 8.0 Hz, -CH2-), 4.57 (2H, t, J = 8.0 Hz, -CH2-), 7.74 (2H, d, J = 9.3 Hz, Ar), 8.26 (2H, d, J = 9.3 Hz, Ar); 13C NMR (CDCl3, 60 MHz, TMS): δ: 44.95, 61.37, 117.48, 125.00, 143.45, 143.80, 154.54; Anal. Calcd for C9H8N2O4: C, 51.93; H, 3.87; N, 13.46. Found: C, 52.03; H, 3.81; N, 13.40.
Compound 3a 1H NMR (CDCl3, 400 MHz, TMS): δ 4.10 (2H, t, J = 7.8 Hz, -CH2-), 4.59 (2H, t, J = 7.8 Hz, -CH2-), 7.44-7.49 (2H, m, Ar), 7.67 (1H, dd, J = 7.8, 7.8 Hz, Ar), 7.67 (1H, d, J = 8.2 Hz, Ar); 13C NMR (CDCl3, 60 MHz, TMS): δ: 47.43, 62.83, 125.96, 128.00, 128.12, 131.53, 133.93, 145.64, 156.21; Anal. Calcd for C9H8N2O4: C, 51.93; H, 3.87; N, 13.46. Found: C, 51.80; H, 3.75; N, 13.66.
Compound 4a 1H NMR (CDCl3, 400 MHz, TMS): δ 4.15 (2H, t, J = 8.0 Hz, -CH2-), 4.57 (2H, t, J = 8.0 Hz, -CH2-), 7.57 (1H, dd, J = 8.2, 8.2 Hz, Ar), 8.00 (1H, d, J = 8.0 Hz, Ar), 8.16 (1H, d, J = 8.5 Hz, Ar), 8.22 (1H, s, Ar); 13C NMR (CDCl3, 60 MHz, TMS): δ 45.01, 61.40, 112.32, 118.59, 123.90, 130.05, 139.53, 148.74, 154.84; Anal. Calcd for C9H8N2O4: C, 51.93; H, 3.87; N, 13.46. Found: C, 52.62; H, 4.10; N, 13.16.
Compound 5a 1H NMR (CDCl3, 400 MHz, TMS): δ 4.04 (2H, t, J = 8.0 Hz, -CH2-), 4.50 (2H, t, J = 8.0 Hz, -CH2-), 7.44 (2H, d, J = 9.1 Hz, Ar), 7.51 (2H, d, J = 9.6 Hz, Ar); 13C NMR (CDCl3, 60 MHz, TMS): δ 29.69, 61.28, 116.88, 119.70, 132.00, 137.43, 155.04; Anal. Calcd for C9H8BrNO2: C, 44.66; H, 3.33; N, 5.79. Found: C, 44.87; H, 3.28; N, 5.80.
Compound 6a 1H NMR (CDCl3, 400 MHz, TMS): δ 4.02 (2H, t, J = 7.9 Hz, -CH2-), 4.55 (2H, t, J = 8.0 Hz, -CH2-), 7.22-7.25 (1H, m, Ar), 7.38-7.40 (2H, m, Ar), 7.65 (1H, d, J = 8.5 Hz, Ar); 13C NMR (CDCl3, 60 MHz, TMS): δ 47.27, 62.60, 122.51, 128.73, 129.73, 129.85, 133.77, 136.48, 156.75; Anal. Calcd for C9H8BrNO2: C, 44.66; H, 3.33; N, 5.79. Found: C, 44.68; H, 3.28; N, 5.78.
Compound 7a 1H NMR (CDCl3, 400 MHz, TMS): δ 4.02 (2H, t, J = 7.9 Hz, -CH2-), 4.54 (2H, t, J = 8.0 Hz, -CH2-), 7.30-7.34 (2H, m, Ar), 7.40 (1H, d, J = 1.8 Hz, Ar), 7.46 (1H, d, J = 1.8 Hz, Ar); 13C NMR (CDCl3, 60 MHz, TMS): δ 47.13, 62.61, 127.99, 129.42(two carbon), 130.61, 132.34, 134.99, 156.85; Anal. Calcd for C9H8ClNO2: C, 54.70; H, 4.08; N, 7.09. Found: C, 54.26; H, 4.11; N, 6.98.
Compound 8a 1H NMR (CDCl3, 400 MHz, TMS): δ 3.80 (3H, s, Me), 4.03 (2H, t, J = 8.0 Hz, -CH2-), 4.47 (2H, t, J = 8.0 Hz, -CH2-), 6.91 (2H, d, J = 9.0 Hz, Ar), 7.43 (2H, d, J = 9.0 Hz, Ar); 13C NMR (CDCl3, 60 MHz, TMS): δ 45.79, 55.54, 61.27, 114.38, 120.38, 131.52, 155.61, 156.50; Anal. Calcd for C10H11NO3: C, 62.17; H, 5.74; N, 7.25. Found: C, 62.10; H, 5.80; N, 7.20.
Compound 9a 1H NMR (CDCl3, 400 MHz, TMS): δ 3.83 (3H, s, Me), 4.04 (2H, t, J = 8.0 Hz, -CH2-), 4.48 (2H, t, J = 8.0 Hz, -CH2-), 6.70 (1H, d, J = 8.3 Hz, Ar), 7.04 (1H, d, J = 8.6 Hz, Ar), 7.26-7.30 (2H, m, Ar); 13C NMR (CDCl3, 60 MHz, TMS): δ 45.36, 55.40, 61.27, 104.59, 109.65, 110.38, 129.78, 139.58, 155.17, 160.28; Anal. Calcd for C10H11NO3: C, 62.17; H, 5.74; N, 7.25. Found: C, 62.42; H, 5.77; N, 7.30.
Compound 10a 1H NMR (CDCl3, 400 MHz, TMS): δ 2.34 (3H, s, Me), 4.05 (2H, t, J = 8.0 Hz, -CH2-), 4.48 (2H, t, J = 8.0 Hz, -CH2-), 7.19 (2H, d, J = 8.3 Hz, Ar), 7.43 (2H, d, J = 8.5 Hz, Ar); 13C NMR (CDCl3, 60 MHz, TMS): δ 20.75, 45.40, 61.28, 118.43, 129.60, 133.80, 135.82, 155.38; Anal. Calcd for C10H11NO2: C, 67.78; H, 6.26; N, 7.90. Found: C, 67.91; H, 6.31; N, 7.87.
Compound 11a 1H NMR (CDCl3, 400 MHz, TMS): δ 2.31 (3H, s, Me), 3.94 (2H, t, J = 8.0 Hz, -CH2-), 4.48 (2H, t, J = 8.0 Hz, -CH2-), 7.23-7.28 (4H, m, Ar); 13C NMR (CDCl3, 60 MHz, TMS): δ 17.83, 47.88, 62.42, 126.51, 127.00, 128.17, 131.38, 135.92, 136.17, 156.80; Anal. Calcd for C10H11NO2: C, 67.78; H, 6.26; N, 7.90. Found: C, 67.71; H, 6.24; N, 7.78.
Compound 12a 1H NMR (CDCl3, 400 MHz, TMS): δ 3.93 (2H, t, J = 8.0 Hz, -CH2-), 4.60 (2H, t, J = 8.0 Hz, -CH2-), 7.44 (2H, s, Ar); 13C NMR (CDCl3, 60 MHz, TMS): δ 45.30, 63.07, 128.98, 131.31, 135.58, 136.36, 155.73; Anal. Calcd for C9H6Cl3NO2: C, 40.56; H, 2.27; N, 5.26. Found: C, 40.45; H, 2.20; N, 5.12.
ACKNOWLEDGEMENTS
We are grateful to the National Natural Science Foundation of China (No. 20572090) and the Higher Education Doctoral Science Foundation of China (No. 20060530002) for financial support.
References
1. M. E. Dyen and D. Swern, Chem. Rev., 1967, 67, 197. CrossRef
2. B. J. Ueberbacher, H. Griengl, and H. Weber, Tetrahedron: Asymmetry, 2008, 19, 838. CrossRef
3. A. Choy, N. Colbry, C. Huber, M. Pamment, and J. V. Duine, Org. Process Res. Dev., 2008, 12, 884. CrossRef
4. T. Komine, A. Kojima, Y. Asahina, T. Saito, H. Takano, T. Shibue, and Y. Fukuda, J. Med. Chem., 2008, 51, 6558. CrossRef
5. S. J. Katz and S. C. Bergmeier, Tetrahedron Lett., 2002, 43, 557. CrossRef
6. S. Fonquerna, A. Moyano, M. A. Pericàs, and A. Riera, Tetrahedron: Asymmetry, 1997, 8, 1685. CrossRef
7. B. B. Lohray, S. Baskaran, B. S. Rao, B. Y. Reddy, and I. N. Rao, Tetrahedron Lett., 1999, 40, 4855. CrossRef
8. D. Ben-Ishai, J. Am. Chem. Soc., 1956, 78, 4962. CrossRef
9. H. L. Crowther and H. J. McCombie, J. Chem. Soc., 1913, 103, 27.
10. F. W. Li and C. G. Xia, J. Catal., 2004, 227, 542. CrossRef
11. B. Gabriele, R. Mancuso, G. Salerno, and M. Costa, J. Org. Chem., 2003, 68, 601. CrossRef
12. Y. Fu, T. Baba, and Y. Ono, J. Catal., 2001, 197, 91. CrossRef
13. T. Baba, A. Kobayashi, T. Yamauchi, H. Tanaka, S. Aso, M. Inomata, and Y. Kawanami, Catal. Lett., 2002, 82, 193. CrossRef
14. M. Tingoli, L. Testaferri, A. Temperini, and M. J. Tiecco, J. Org. Chem., 1996, 61, 7085. CrossRef
15. Y. Ono, Catal. Today, 1997, 35, 15. CrossRef
16. D. Delledonne, F. Rivetti, and U. Romano, Appl. Catal. A: Gen., 2001, 221, 241. CrossRef
17. B. M. Bhanage, S. I. Fujita, Y. Ikushima, and M. Arai, Green Chem., 2004, 6, 78. CrossRef
18. B. M. Bhanage, S. I. Fujita, Y. Ikushima, and M. Arai, Green Chem., 2003, 5, 340. CrossRef
19. M. L. Kantam, U. Pal, B. Sreedhar, and B. M. Choudary, Adv. Synth. Catal., 2007, 349, 1671. CrossRef
20. L. F. Xiao, L. W. Xu, and C. G. Xia, Green Chem., 2007, 9, 369. CrossRef
21. S. R. Jagtap, Y. P. Patil, S. I. Fujita, M. Arai, and B. M. Bhanage, Appl. Catal. A: Gen., 2008, 341, 133. CrossRef
22. F. Shi, Q. H. Zhang, Y. B. Ma, Y. D. He, and Y. Q. Deng, J. Am. Chem. Soc., 2005, 127, 4182. CrossRef
23. D. J. Darensbourg and M. W. Holtcamp, Coord. Chem. Rev., 1996, 153, 155. CrossRef
24. B. M. Bhanage, S. I. Fujita, Y. Ikushima, and M. Arai, Appl. Catal. A: Gen., 2001, 219, 259. CrossRef
25. S. R. Jagtap, M. J. Bhanushali, A. G. Panda, and B. M. Bhanage, Catal. Lett., 2006, 112, 51. CrossRef
26. S. R. Jagtap, V. P. Raje, S. D. Samant, and B. M. Bhanage, J. Mol. Catal. A: Chem., 2006, 266, 69. CrossRef
27. F. W. Li, L. F. Xiao, and C. G. Xia, Tetrahedron Lett., 2004, 45, 8307. CrossRef
28. S. Tanimori and M. Kirihata, Tetrahedron Lett., 2000, 41, 6785. CrossRef
29. W.-C. Shieh, M. Lozanov, M. Loo, O. Repič, and T. J. Blacklock, Tetrahedron Lett., 2003, 44, 4563 CrossRef