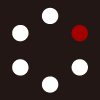
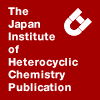
HETEROCYCLES
An International Journal for Reviews and Communications in Heterocyclic ChemistryWeb Edition ISSN: 1881-0942
Published online by The Japan Institute of Heterocyclic Chemistry
e-Journal
Full Text HTML
Received, 31st July, 2009, Accepted, 1st October, 2009, Published online, 1st October, 2009.
DOI: 10.3987/COM-09-S(S)94
■ Unusual Reactions of the Highly Strained Macrocycles with Lithium Salts: Anion Control for the Reaction Rates and Elucidation of the Properties of Their Lithium Complexes
Junko Morita, Shinji Tsuchiya, Nao Yoshida, Nirei Nakayama, and Shojiro Ogawa*
Center of Environmental Science for Human Life, Ochanomizu University, 2-1-1 Otsuka, Bunkyo-ku, Tokyo 112-8610, Japan
Abstract
The complexation reactions of dicyano monoalkyl tetraaza macrocycle 3b with lithium salts (Li+X-) and the properties of resulting macrocycle 3b-Li+ X- complexes were examined in detail by means of 1H-NMR spectroscopy, because unsymmetrical macrocycle 3b has exhibited intriguing features, such as high selectivity for binding to the Li+ ion. The following noticeable facts were found; the reaction rates of complexation are heavily controlled by the properties of anion (X-) and the spectroscopic properties of macrocycle 3b-Li+ X- complexes are also changed by the anion. The major origin for the unusual Li+ complexation reactions and the specific behavior of Li+ complexes would come from the unique properties of metal free macrocycle 3b, because macrocycle 3b has a strong intramolecular hydrogen bond in the macrocyclic ring and the highly strained macrocyclic ring by unsymmetrical structure consisting of nonplanar and planar moieties.INTRODUCTION
The macrocycles are a popular and useful class of compounds. For example, some macrocycles are able to catch a specific metal ion in the presence of various metal ions. Other macrocycles hold promise as a potential cancer therapeutic.1 Though typical macrocycles exhibiting these features are a series of oxygen atom-containing macrocycles such as crown ethers used most often, the mainstream of the highly functionalized macrocycles is no longer restricted to these compounds. The researchers are therefore required to have a knowledge base, which is much broader than those of oxygen atom-containing macrocyclic compounds.
To develop a new class of macrocycles exhibiting highly functionalized properties such as chemical sensors for medical uses, molecular devices, and displays for electrical devices, we had tried to synthesize novel macrocycles including many nitrogen heterocycles other than crown ethers.2-9, 15, 20 One of the examples prepared by us is a series of pyridine-containing tetraaza macrocycle 1 as shown in Scheme 1.5,6
Macrocycle 1 with nonplanar macrocyclic rings exhibits high selectivity for binding to the Li+ ion among alkali and alkaline earth metal ions.5,6 This macrocycle 1 can switch back and forth between nonfluorescent molecule 1 and fluorescent molecule 2; specifically, macrocycle 1 can switch to a fluorescent state 2 with more than 1000 times of fluorescence intensity by Li+ complexation.7-9 The versatility of this tetraaza macrocycle system has provided some of impetus for further exploration of Li+ selective aza macrocycles.
The idea of creating a more functionalized aza macrocycle for the Li+ ion is to introduce a unique geometry into the macrocyclic ring system, because the ability to catch the Li+ ion is believed to be closely related to a suitable cavity produced by macrocyclic ring and the specific electronic state by unique stereochemical structures.10-14 Based on this synthetic principle, macrocycle 3 with hybrid properties of nonplanar A moiety (alkyl-di(2-pyridyl)acetonitrile group) and planar B moiety (2-pyridyl-2-(1H)-pyridylidene acetonitrile group) were synthesized.15 As shown in the following Scheme 2, the striking features of this macrocycle 3 are the highly strained macrocyclic structure and a hydrogen atom in the core of macrocyclic ring. This hydrogen atom (H17) shows a strong intramolecular hydrogen bond and is labile due to the distorted cyclic structure.
This unsymmetrical macrocycle 3 has been proved to have the unusual spectroscopic properties, which may make it an important player in the color switching system; macrocycle 3 exhibits the solvent-responsive property with positional change of the hydrogen atom (H17) as an antenna.15
This fascinating property of macrocycle 3 that is produced by the strained ring structure and a labile hydrogen atom asked us to focus on exploring the ability as a Li+ ion ionophore, because the interaction between the Li+ ion and the macrocycle is expected to be heavily affected by these features of macrocycle 3.
On the other hand, the property of lithium has made it the object of a great deal of chemical interest and study, because there is presently great demand of lithium, that is caused by lithium ion battery for a cell phone or a car.16 However, the traditional lithium separation from ore is not environmentally benign and requires the high energy, and this method does not fit the pursuit of sustainability. Despite extensive studies of membrane technology which is a better method for the separation, relatively little attention has been paid to the Li+ ion separation by a liquid membrane. In particular, the previously reported Li+ selective carriers are unsuitable for practical applications and more efficient carrier molecules for use in the liquid membrane are required. 17-19
For these reasons, the ability of macrocycle 3 as a Li+ ion carrier in the liquid membrane had been explored. Examination for the property of macrocycle 3 indicated this molecule to exhibit a desirable property as a carrier of LiCl.20 A noticeable result was that pure LiCl separation from the seawater including 2.88 x 10-5 mol/L of LiCl ( 0.005 mol% of total amount of NaCl, KCl, LiCl, MgCl2, and CaCl2 ) can be realized by using the liquid membrane system including macrocycle 3.20 This performance is the key if one wants to use an environmentally friendly, low energy membrane system for practical purposes of lithium separation.
This is a very important and interesting discovery, which may lead to an understanding of how the macrocycle accomplishes the discrimination between the Li+ ion and other metal ions. The best way to clear up uncertainties about the ability of discrimination would elucidate a mechanism about the complexation reactions of the macrocycles with lithium salts and the detailed properties of macrocycle-Li+ complexes. We already published a paper about the complexation reactions of macrocycle 3 with the Li+ ion; when macrocycle 3 forms Li+ complex 4, the hydrogen atom in the core of macrocycle is excluded to the outside of the ring, and the color of solution changes from orange to colorless as shown in above Scheme 2.15 In spite of this intriguing fact, we do not know how this complexation reaction occurs. To explore this mechanism, we need to follow the exact reaction processes under various conditions. Attempts were therefore made, using LiCl, LiBr, LiI, LiClO4, and LiOH as the lithium salts, to elucidate how this complexation reaction proceeds under the given conditions. A key method for exploring this reaction is the 1H-NMR spectroscopy, because the previously reported 1H-NMR data of macrocycle 3 have afforded an important information about the transformation of macrocyclic skeleton.15
The results of this experiment afforded unusual facts that the reaction rate of complexation is closely related to the property of anion as a counterion and the proton shift of hydrogen atom on the macrocyclic ring after the transformation by Li+ complexation is exquisitely susceptible to the anion. This is extremely noticeable not only because it is the first example of anion effect on the complexation reaction of macrocycle with the Li+ ion, but also because of the effect of the anion (X-) on the properties of macrocycle-Li+ X- complexes. Our general focus of this research is to understand how the anion (X-) influences the reaction rates of unsymmetrical macrocycle 3b with lithium salts and the properties of their Li+ complexes.
In this paper, we wish to report the detailed data about the complexation reactions and the reason why this unusual phenomenon occurs.
RESULTS AND DISCUSSION
To explore the reaction mechanism of unsymmetrical macrocycle 3b with the Li+ ion, the 1H-NMR spectra of probing the reaction processes of metal free macrocycle 3b with various lithium salts (Li+X-) were measured in CD2Cl2. The reaction model that we are using to study this complexation reaction is as follows; doing this study is acceptable when the interaction between macrocycle 3b and the Li+ ion can be disturbed readily by some factors. Thus, lithium salts (LiCl, LiBr, LiI, LiClO4, and LiOH), which are suitable for this type of investigation, were selected for this experiment. This selection has certain advantages over other methods, because the anion, which is included in lithium salt, is more effective to try a systematic survey of the interaction between the macrocycle and the Li+ ion.
The 1H-NMR spectra obtained in these experiments are presented in Figures 1-6 and their data are summarized in Table 1. These data including the figures and a table showed definite evidence that the significant spectral changes on complexation with lithium salts occur, and the reaction rates and the properties of their Li+ complexes depend on the anion of lithium salts.
As described in our earlier publication,15 we were able to obtain the important information about the structures and electronic states of metal free macrocycle 3b and its zinc complexes by means of 1H-NMR spectroscopy. We therefore decided to measure the transient 1H-NMR spectra during the reactions of macrocycle 3b with lithium salts. The use of dichloromethane-d2 as a solvent for 1H-NMR measurements could result in unequivocal transient spectra, because the reaction rates of these complexation reactions are slower in dichloromethane than other solvents.
The Complexation Reactions of Macrocycle 3b with Lithium Salts in Dichloromethane. As shown in Figure 1, the 1H-NMR spectrum in the complexation reaction of macrocycle 3b with LiCl did not change immediately after mixing these compounds in CD2Cl2, but the new small peaks appeared at the area of around δ8 ppm after 2 hours (Figure 1 (3)). Though the new peaks of reaction product and the original peaks of starting metal free macrocycle 3b coexisted during 2 days (Figures 1 (4) and (5)), most of original signals assigned to metal free macrocycle 3b had disappeared after 9 days (Figure 1 (6)); about 90% of macrocycle 3b reacted with LiCl to form its lithium complex, which was estimated from the integral values of the peaks in its 1H-NMR spectrum. The new peaks observed atδ7.96 – 8.14 ppm were assigned to macrocycle 3b-Li+Cl- complex, and in particular, two doublet peaks (δ8.10 andδ8.14 ppm) in these peaks were specified to H4,12 and H5,11 protons. The proton signals of macrocycle 3b-Li+Cl- complex appeared at lower field than those of A part (pyridine moieties: H2,14, H3,13, H4,12) of starting metal free macrocycle 3b (Scheme 2). This means that the lithium ion in this complex withdraws the electrons of macrocycle as a ligand and as a result, the electron density of macrocycle 3b-Li+Cl- complex protons becomes much lower than that of starting metal free macrocycle 3b. The signal corresponding to the H17 proton in the core of metal free macrocycle 3b appeared atδ15.64 ppm, indicating a strong intramolecular hydrogen bond. The peak intensity of this proton (H17) decreased with time. In stead of decreasing peak intensity of H17 proton, a new small peak appeared atδ7.66 ppm. As described in earlier our publication,15 this fact means that the hydrogen atom (H17) in the core of macrocyclic ring is excluded to the outside of the ring and this hydrogen atom transforms from H17 to H8 on the macrocyclic ring. Since this peak (H8) did not appear clearly in its spectrum as shown in the Figure 1, the spectrum area where the peak of H8 appeared was examined in detail. By the expanded spectra in the region betweenδ7.5 and 7.7 ppm, the peak of H8 proton was observed to appear among the triplet peaks of H6 and H10 protons.
There is a possibility that this complextion reaction is affected by the size and electronegativity of anion (X-, counterion). The complexation reaction of macrocycle 3b with LiBr was therefore carried out to examine this anion effect. As shown in Figures 1 and 2, a definite difference in reaction rates between LiCl and LiBr was observed. That is, macrocycle 3b reacted rapidly with LiBr to give its Li+ complex in comparison with LiCl. The new peaks with formation of complex appeared at lower field than the signals of metal free macrocycle 3b after 1 hour of mixing of macrocycle 3b and LiBr in CD2Cl2 (Figure 2 (3)). About 20% of macrocycle 3b was complexed after 5 hours (Figure 2 (5)). After 1 day, all of proton signals assigned to metal free macrocycle 3b disappeared and the only signals assigned to the macrocycle 3b-Li+Br- complex were observed. Thus, the rate of LiBr is extremely faster than that of LiCl. This spectrum pattern was also quite the same during 8 days (Figure 2 (7)), indicating the formation of stable lithium complex. The spectrum pattern of macrocycle 3b-Li+Br- complex is similar to that of macrocycle 3b-Li+Cl- complex, except that the signal position of H8 hydrogen atom, which is pushed out from the core to the ring; the H8 peak of macrocycle 3b-Li+Br- complex appeared atδ7.79 ppm, which was lower field than that (δ7.66 ppm) of macrocycle 3b-Li+Cl- complex ( Table 1 ).
In the case of complexation reaction with LiI, the trend of faster reaction rate and lower field shift of H8 proton is much enhanced. As shown in 1H-NMR spectral changes by the reaction of macrocycle 3b with LiI ( Figure 3 ), the signals of metal free macrocycle 3b disappeared immediately after mixing of two compounds, and the only signals assigned to macrocycle 3b-Li+I- complex appeared. Three spectra of macrocycle 3b-Li+I-, macrocycle 3b-Li+Br-, and macrocycle 3b-Li+Cl- complexes, which appear in the spectrum region fromδ7.95 to 8.20 ppm, are identical in the peak shapes. However, the signal of H8 proton on the ring of macrocycle 3b-Li+I- complex appeared atδ7.87 ppm (Figure 3 (2)), indicating the lower field shift with decreasing electronegativity of anion as shown in Table 1.
Eventually, an intriguing tendency for the complexation reaction of macrocycle 3b was observed, when the halogen atoms were used as the anions (counterions); the reaction rate becomes faster, and the proton signals of H8 and bipyridine moieties move to lower field with decreasing electronegativity of anion (Cl-, Br-, and I-). However, the clear understanding of how lithium halide reacts with macrocycle 3b could not be obtained by only experimental results described above. Of particular interest is the role of anion in changing the reaction rate and the peak position of H8 proton. Thus, it is necessary to further investigate the complexation reactions of macrocycle 3b with lithium salts having other kinds of anions for obtaining more evidence about the difference in the interaction between the complex and the anion from measurable methods. The good candidates as the anions are perchlorate (ClO4-) and hydroxide (OH-), because the properties of these species as the anions are very different from those of lithium halides, such as the solubility to organic solvents.
1H-NMR spectral changes after mixing macrocycle 3b and LiClO4 in CD2Cl2 were shown in Figure 4. The new signals appeared at aroundδ8.0-8.2 ppm after standing of reaction mixture for 1 hour (Figure 4 (2)). About 23% of macrocycle 3b was complexed after 2 hours (Figure 4 (3)) and subsequently, about 35% of macrocycle 3b was complexed after 5 hours (Figure 4 (4)). After 1 day, all of the signals assigned to metal free macrocycle 3b disappeared and the only peaks of macrocycle 3b-Li+ClO4- complex was observed in its 1H-NMR spectrum (Figure 4 (5)). This spectral pattern has been kept up for 8 days as shown in Figure 4 (6). Though the whole spectral pattern of this macrocycle 3b-Li+ClO4- complex resembles those of Li+ complexes having the halide ions, the shape of proton peaks which correspond to bipyridine moieties is much sharper. The feature which is plainly visible is the large difference in the peak positions of H8 proton between perchlorate and halides; that is, the H8 signal of macrocycle 3b-Li+ClO4- complex appeared atδ6.61 ppm and this is extremely higher than those of macrocycle 3b-Li+X-complexes with halide ions (X = Cl-, Br-, I-) as shown in Table 1.
As mentioned above, the reaction of macrocycle 3b with lithium salt LiX (X- = Cl-, Br-, I-, ClO4-) afforded complex 4 as shown in Scheme 2.15 Complex 4 is supposed to have the stereochemical structure 2 of following Scheme 3. We can figure out this structure by combining the knowledge of macrocycle 1 and its Li+ complex 2 in our earlier publication5 with the 1H NMR data described above.
In the case of hydroxide (OH-) being used as an anion, somewhat different complexation reactions seem to proceed. That is to say, after standing of the solution for 1 day, this complexation reaction gave new several multi-peaks at the side of lower field compared to metal free macrocycle 3b. These multi-peaks would be assigned to the signals of complex consisting of macrocycle 3b and the Li+ ion. The peak intensities of these signals with complexation increased with time and the yields of Li + complex after 1 day and 2 days were 16 % and 68 %, respectively (Figure 5 (3) and (4)). After 9 days, all of metal free macrocycle 3b was found to lead to its Li+complex (Figure 5 (5)). Additionally, a signal, which is considered to be assigned to H8 proton of this macrocycle 3b-Li+ complex, appeared at δ7.33 ppm. The noticeable feature (Figure 5 (5)) is that this spectral pattern is quite different from those of other macrocycle 3b-Li+X- complexes described above. That is, in comparison to the spectra of macrocycle 3b-Li+X- complexes including other anions (Cl-, Br-, I- and ClO4-), the shapes of these peaks are significantly sharp and complicated. For example, the 1H-NMR spectrum of macrocycle 3b-Li+ClO4- complex consists of one large peak and two doublet peaks, but three doublet and one triplet peaks are observed in the spectrum of this Li+ complex as shown in Figure 5 (5). This spectral pattern is more similar to metal free macrocycle 3b itself rather than typical spectral pattern of macrocycle 3b-Li+X- complex. This suggests that this complex includes not only alkyl-di(2-pyridyl)acetonitrile moiety in the macrocyclic skeleton but also the 2-pyridyl-2-(1H)-pyridylideneacetonitrile moiety, because the multi-peaks observed in this spectrum seem to be arisen from the 2-pyridyl-2-(1H)-pyridylideneacetonitrile moiety. One plausible explanation about these experimental results is that two kinds of macrocycle 3b-Li+ complexes 4 and 5 shown below exist in this solution.
The existence of complex 5 is due to the appearance of peaks which would be assigned to 2-pyridyl-2-(1H)-pyridylideneacetonitrile moiety. Since complex 5 is easy to form under basic conditions as described earlier in our publication,15 the hydroxide (OH-) of LiOH used in this reaction is considered to function partially as a base after initial formation of complex 4 and to form complex 5. A reason why complex 4 exists in these reaction products comes from the appearance of H8 proton signal in this 1H-NMR spectrum (Figure 5 (5)).
Eventually, as shown in above spectra, the reaction rates and the signal shift of H8 proton are heavily influenced by the character of anion (counterion), such as Cl-, Br-, I-, ClO4-, and OH-.
The Reaction Rates of Macrocycle 3b with Lithium Salts Observed by UV-Visible Spectroscopy. The results by the 1H-NMR measurements showed the anion effect on the reaction rate. To confirm this effect, we need further information about the reaction rates by other spectroscopic methods under different reaction conditions. Thus, the reaction rates of macrocycle 3b with lithium salts in homogeneous system were measured by UV-Visible method. The acetonitrile was used as a solvent, because lithium salts are moderately soluble in acetonitrile. An acetonitrile solution (0.2 M) of lithium salt was added to an acetonitrile solution of macrocycle 3b (5.45 x 10-5 M) and the decrease of the peak intensity at 462.5 nm was measured with time. By these procedures, the relative ratios of initial reaction rates were obtained; the ratios of ClO4- : I- and Br-: I- were 0.85 and 0.5, respectively. 21 The fastest reaction rate was found in the reaction of macrocycle 3b with LiI, and the order of increasing reaction rate is I- > ClO4- > Br-. The result of this relative initial reaction rate is in good agreement with that by 1H-NMR measurements and this observation corroborates the 1H-NMR measurements described in above section.
The Mechanism of Complexation Reaction. In our earlier publication, we described the results about the reactions of macrocycle 3b with CuCl2, CoCl2, FeCl2 and SnCl2 in dichloromethane.15 Typically, these reactions proceed as shown in the following Scheme 4 and form macrocycle-metal complexes 5 with alkyl- di(2-pyridyl)acetonitrile and 2-pyridyl-2-(1H)-pyridylideneacetonitrile moieties.
The structures of these complexes 5 are different from those of complexes 4 having a hydrogen atom on the macrocyclic ring as described in above sections. In this reaction, the hydrogen atom in the core of macrocyclic ring of metal free macrocycle 3b is replaced by the metal ion (M2+) with the elimination of hydrochloric acid (HCl). Thus, the hydrogen atom binds neither in the core of macrocyclic ring nor on the ring. More interestingly, when macrocycle 3b reacted with ZnCl2, both types of the complex 4 (type 4) and complex 5 (type 5) were formed. Whether the complex of type 4 is formed or the complex of type 5 is formed depends on the solvent used in the reaction.15
Most of macrocycle 3b-Mn+ complexes formed by the reaction of macrocycles 3b with metal chlorides had the structure of type 5, and the macrocycle 3b-Mn+ complex having the structure of type 4 was formed under special reaction conditions, such as LiCl and ZnCl2 in dichloromethane. Even if the zinc complex of type 4 is formed, the complex of type 4 is easily transformed to the complex of type 5 by the basic solvents or weak basic reagents such as amines.15
What is unclear is a reason why unusual complexation occurs in this reaction system. The observation described above has led to the consideration about this reason. That is to say, this unusual phenomenon would come from unique property of macrocycle 3b. The feature of macrocycle 3b is the unusual hybrid structure consisting of nonplanar part A ( alkyl-di(2-pyridyl)acetonitrile moiety ) and planar part B ( 2-pyridyl-2-(1H)- pyridylideneacetonitrile moiety) as shown in Scheme 2. The bridging carbon atom between two pyridine moieties of nonplanar part A is sp3 and then, these pyridine moieties are tilted each other. In contrast, the part B has a strong intramolecular hydrogen bond in the ring and the conjugated double bond on the ring. These strong intramolecular hydrogen bond and the conjugation would force macrocycle 3b into the planar structure of the B part. The whole ring of macrocycle 3b has therefore a big strain and distortion owing to this hybrid structure consisting of planar and nonplanar parts. This highly strained ring structure would afford the unusual complexation reaction of macrocycle 3b with metal salts.
Though a metal ion (Mn+) of macrocycle 3b-Mn+complex would be coordinated by the nitrogen atoms of four pyridine ring moieties, the interaction between the metal ion and the nitrogen atoms of two pyridine ring moieties of the planar B part could play an important role in forming the complex at initial step. The loss of the intramolecular hydrogen bond accompanied by the coordination of metal ion is considered to result in two unusual modes of reactions (1) and (2). (1) When the interaction between the metal ion and two nitrogen atoms of the part B is strong, the conjugated system consisting of the metal ion and the double bond on the macrocyclic ring is formed and the planar structure of the part B will be kept as shown in below structure 6. As a result, this system is stabilized and the hydrogen atom in the core is removed from the part B as hydrochloric acid. This is the case when CuCl2, CoCl2, FeCl2 and SnCl2 are used.
As shown in above structure, the interaction between the metal ion and two nitrogen atoms of the part B is quite different from that of the part A. This fact can be confirmed by 1H NMR spectrum of macrocycle 3b-Zn complex of type 5 formed in methanol as shown in earlier our publication; this spectrum exhibited the existence of two kinds of pyridine moieties.15 On the other hand, when the interaction between the metal ion and two nitrogen atoms of the part B is weak, another reaction (2) occurs. (2) The weak interaction between the metal ion and the nitrogen atoms of two pyridine rings of the part B is supposed to induce the breakup of energy balance between the bonds of the B part and the ring strain of the whole ring, because the intramolecular hydrogen bond, which is lost in this complexation process, could possibly play an important role in keeping the planarity of B part of macrocycle 3b. As a consequence, the tilting effect induced by the ring strain becomes much larger. This allows each pyridine moieties of part B of macrocycle 3b to rotate, thus making a transformation from sp2 hybrid orbital of the bridging carbon atom of the part B to sp3 hybrid orbital. This excludes the hydrogen atom from the core of macrocyclic ring (H17) to the bridging carbon atom of the outside of ring (H8) and results in the formation of macrocycle 3b-M complex of type 4 as shown in above Schemes 2 and 3. This is the case when LiCl and ZnCl2 are used in dichloromethane. It is generally accepted that the interaction between the Li+ ion and the ligand or the Zn2+ ion and the ligand is much weaker than that between the ligand and the transition metal ion, such as the Cu2+ ion, the Co2+ ion, and the Fe2+ ion. Additionally, it is supposed that the stability of the complex of type 4 formed in dichloromethane is considerably low and the hydrogen atom (H8) on the bridging carbon atom is labile. Thus, this hydrogen atom of zinc complex of type 4 is easy to be removed by methanol or the weak bases, and the zinc complex of type 5 is formed. The interaction between the metal ion and the nitrogen atoms of four pyridine rings in the complex of type 4 is similar each other compared with the case of complex of type 5. For example, the 1H-NMR measurements showed that the multi-peaks of metal free macrocycle 3b unite to form a peak by forming the Zn complex of type 4, indicating the existence of four pyridine moieties with similar environment as shown in the spectrum previously reported.15 This point is quite different from the 1H-NMR spectrum of complex of type 5 described above. Specifically, it is impressive observation that the distinctly separated and sharp multi-peaks of metal free macrocycle 3b due to two different electronic states of the part A and part B is transformed dramatically into one peak by the formation of complex of type 4, such as Zn2+ or Li+ complexation.
The Effect of the Anion (X-) on the Reaction Rates. The above discussion would lead to one conclusion that the interaction between the metal ion and the nitrogen atoms of two pyridine moieties of the part B could possibly play an important role in forming the macrocycle 3b-metal complex. Especially, the formation of complex of type 4 would be heavily influenced by this effect, because the stability of complex of type 4 is supposed to be considerably lower. In reverse words, if the interaction between the metal ion and two nitrogen atoms of part B is changed by some factors, there is the possibility that the formation reaction of complex is also controlled by these factors. One of the factors that could make it possible is considered to be the anion (X-) in the complexation reaction of macrocycle 3b with lithium salts (Li +X-), that is described in above section.
As mentioned above, the complexation reactions of macrocycle 3b with lithium salts in dichloromethane afforded the complex of type 4, and their reaction rates and the signal shift of H8 proton on the macrocyclic ring depended on the anion, such as Cl-, Br-, I- and ClO4-. These experimental results could be explained by electrostatic Li+ ion-anion (X-) interaction, which depends on the properties of anion; its electronegativity, size, and charge. By considering the properties of anion, the observed difference in the reaction rates between Cl- and Br- would be explained as follows. Originally, the interaction between the Li+ ion and two nitrogen atoms of the B part is smaller compared with those of transition metals because of monovalent Li+ ion. The stabilization effect by formation of metal ion-double bond conjugation system observed in the case of the transition metal ion is more difficult as described above, and this reaction has therefore the trend that forms the Li+ complex of type 4. The interaction between the Li+ ion and the Cl- ion is much larger than that between the Li+ ion and the Br- ion because of higher electronegativity and smaller size of the chloride anion. The stronger electrostatic interaction between the Li+ ion and the Cl- ion would make the interaction between the Li+ ion and the nitrogen atoms of pyridine moieties weaker. Thus, the reaction rate of macrocycle 3b with LiCl would become slower compared with that of macrocycle 3b with LiBr. The interaction between these two species in solvents of low polarity such as CH2Cl2 may be affected by the solubility properties of anion. It is considered that the difference in the solubility between LiBr and LiCl may help partially the enhancement of reaction rate.22, 23
The difference in the H8 peak position between macrocycle 3b-Li+Cl- complex and macrocycle 3b-Li+Br- complex could be also explained by the difference in the interaction between the Li+ ion and the anion (counterion). The anion (X-) in macrocycle 3b-Li+X- complex is considered to interact with the Li+ ion in the core of macrocyclic ring. This interaction between the anion and the Li+ ion could result in some variation of the interaction between the Li+ ion and the nitrogen atoms of four pyridine moieties of macrocycle in complex. That is, the larger interaction between the Li+ ion coordinated by macrocycle and the Cl- ion induces smaller interaction between the Li+ ion and the nitrogen atoms of four pyridine moieties in macrocycle 3b-Li+ X- complex. As a result, the electron density of macrocyclic ring in macrocycle 3b-Li+Cl- complex is much higher than that of macrocycle 3b-Li+ Br- complex and then, the peak of H8 proton of macrocycle 3b-Li+Cl- complex appears at higher field. The higher electron density of macrocyclic ring for macrocycle 3b-Li+Cl- complex can be confirmed from the evidence that the proton peaks of bipyridine moieties of macrocycle 3b-Li+Cl- complex appear at higher field in comparison with those of macrocycle 3b-Li+Br- complex as shown in Table 1.
The difference in the rate between the reaction of macrocycle 3b with LiBr and that of macrocycle 3b with LiI and the difference in the peak shift of H8 proton between their macrocycle-Li+ X- complexes can be also explained by the same reason. As shown in Figure 4, the reaction rate is strongly accelerated by the I- anion.22
By changing the anion to ClO4-, we can achieve another intriguing fact. The whole spectral pattern of macrocycle 3b-Li+ ClO4- complex was extremely similar to those of Li+ complexes having halide anions and its H8 proton peak appeared atδ6.61 ppm that was the highest field shift among the anions used in this study. If the mechanism mentioned above is operative, the higher field shift must lead slower reaction rate. The reaction rate of macrocycle 3b with LiClO4 is therefore expected to be slower than that of macrocycle 3b with LiCl. However, the reaction rate of macrocycle 3b with LiClO4 was faster than that of macrocycle 3b with LiCl. Thus, the rule we present is not sufficient to explain this result. This discrepancy would probably come from the difference in the solubility between LiClO4 and LiCl. With use of methanol, the effect of solubility on the reaction rate will be able to be examined, because LiClO4 is more soluble in methanol rather than dichloromethane. This experiment was therefore made and indicated that the reaction rate of macrocycle 3b with LiClO4 is enhanced greatly by the addition of methanol to the reaction solution of dichloromethane.22 It would be possible to change mutual interaction between the metal ion, the anion (counterion), and the macrocycle by providing workable solubility. Another possible reason for the highest field shift of H8 proton is that the coordination type of macrocycle 3b-Li+ ClO4- complex differs from that of macrocycle 3b-Li+ halide anion complexes, suggesting the variation of the number of coordinated anions and the difference in the interaction between the hydrogen atom ( H8 ) and the anion.
The 1H-NMR spectral pattern observed in the reaction of macrocycle 3b with LiOH is quite different from that of other complexation reactions (Cl-, Br-, I-, ClO4-) in that in addition to being sharp peaks, this 1H-NMR spectrum shows several and complicated multi-peaks. Our explanation and rule established for Cl-, Br-, I-, ClO4- therefore faces some difficulty. As described in above section, the answer for this spectrum pattern is that two kinds of macrocycle 3b-Li+ complexes (type 4 and 5) would coexist in the solution. This indicates that macrocycle 3b reacts with LiOH in dichloromethane to form the mixed complexes (type 4 and 5). As this reaction differs from those by using other anions, a direct comparison with other findings about the reaction rate and the H8 peak position of Li+ complex could not profitably be made. However, this spectrum shows that the anion (OH-) could interact with not only the Li+ ion coordinated by macrocycle but also the hydrogen atom (H8) on the macrocyclic ring. Though our above discussion includes the electrostatic effect of the anion as playing a possibly important role in the interaction between the anion and macrocycle Li+ complex, the result of this reaction indicates that the OH- anion functions as a base to remove the hydrogen atom from the macrocyclic ring. We do not have now a few clues about what governs whether or not OH- acts as the base. There is also the possibility that other kinds of mechanisms are at work in this reaction, but whether such mechanisms necessarily work is not yet clear. Even if only two Li+ complexes are formed, it is impossible to separate directly this multi-peaks in the spectrum into each components of two complexes. Detailed discussion about the complex of type 4 formed in this reaction will be carried out in the future.
The Ability of Macrocycle 3b as a Carrier in Liquid Membrane Systems. The experimental findings indicate that the interaction between the Li+ ion and the nitrogen atoms of four pyridine ring moieties in the complex of type 4 is considerably weak. This means that the stability of the Li+complex of type 4 is not so high in dichloromethane. But it seems possible that this complex has the stability of being enough to pass through an organic membrane. This property would provide a desirable ability of being a carrier of LiCl as described in our earlier publication,20 because the stability is not too high to release the Li+ ion into the receiving phase. The effect of the molecular structure and substituents on the stability of macrocycle-Li+ complex seems to be extremely sensitive and delicate. For example, the stability of structurally similar Li+ complex 2, which is shown in Scheme 1, was so high that macrocycle 1 was unable to use as the carriers of the Li+ ion in the liquid membrane. Thus, the Li+ complex of type 4 would be a tailoring compound to separate the Li+ ion by liquid membrane systems. In reverse words, the Li + ion separation system using liquid membranes is one of the most promising targets for application of metal free macrocycle 3b. This result suggests that the molecular design by us leads to a new Li+ ion ionophore with highly functionalized ability, and in reverse words, a key obstacle to using nitrogen atom-containing macrocycles as a metal ion carrier for the liquid membrane systems can be overcome by using our synthetic strategies.
The facts that macrocycle 3b has not only the enough ability to discriminate the specific metal ion but also unique spectroscopic properties such as the color switching, the change of fluorescent intensity, and the temperature-dependent 1H-NMR spectrum indicate that this type of macrocycles will give rise to a considerable amount of chemistry with a diversity.
CONCLUSION
The highly distorted macrocycles 3b, which consists of nonplanar alkyl- di(2-pyridyl) acetonitrile group (part A) and planar 2-pyridyl-2-(1H)-pyridylideneacetonitrile group (part B) including a strong intramolecular hydrogen bond, exhibits a new type of complexation reaction. That is, the reactions of macrocycle 3b with metal salts afforded two types of complexes (I) and (II); (I) the complex of type 5 forming by replacement of hydrogen atom in the core by the metal ion (Mn+) and removal of HX, and (II) the complex of type 4 forming by transfer of hydrogen atom from the core to the macrocyclic ring.15 Whether the complex of type 5 is formed or whether the complex of type 4 is formed is dependent upon the metal ion and the solvent used in the complexation reactions. Another unique property of macrocycle 3b was to exhibit high selectivity for binding to the Li+ ion among alkali and alkaline earth metal ions.15 We were therefore interested in seeing if we can explore this unusual phenomenon by studying the complexation reaction of macrocycle 3b with lithium salts (Li+X-). Using LiCl, LiBr, LiI, LiClO4 and LiOH, the complexation reactions of macrocycle 3b with lithium salts were carried out in dichloromethane. This experiment provided the fascinating facts that the complexation of macrocycle 3b with lithium salt gives the complex of type 4, and the anion (X-) of lithium salt (Li+X-) has a big effect on the reaction rate and the signal shift of the H8 proton on the macrocyclic ring of macrocycle 3b-Li+ X- complex formed. That is, the order of decreasing reaction rate was as given below; I- > ClO4- > Br- > Cl-. The peak of H8 proton of Li+ complex shifted to higher field side with variation of the anion in order of I-, Br-, Cl-, and ClO4- (Figure 6).
These results can be explained by the high strain of the macrocyclic ring, the strong intramolecular hydrogen bond (H17), the conjugated double bond of the planar group (part B), and the interaction between the metal ion (Mn+), four pyridine moieties, and the anion (X-).
Most of the reactions of macrocycle 3b with the metal ion provide the formation of the complex of type 5 due to the stabilization of metal ion-conjugated double bond system, which is caused by the strong interaction between the metal ion and two pyridines of the planar B part. In contrast, the formation of the complex of type 4 would probably arise from the weak interaction between the metal ion and two pyridines of the part B. This weak interaction is unable to compensate the loss of intramolecular hydrogen bond, though the intramolecular hydrogen bond and the conjugated double bond of macrocyclic ring force macrocycle 3b into the planar structure of part B. As a result, two pyridine moieties of the B part are tilted due to the highly strained ring, and the bridging carbon atom between these pyridine moieties does not keep sp2 hybrid orbital. This leads the transfer of hydrogen atom from the nitrogen atom of pyridine moiety to the bridging carbon atom and then, the complex of type 4 is formed. Specifically, the reaction rate for the formation of the complex of type 4 would be very sensitive to mutual interaction between the metal ion and pyridine moieties, and then be changed by some factors affecting this interaction. One of such factors would be the anion, which was examined by this investigation. The interaction between the metal ion and the nitrogen atoms of pyridine moieties is heavily influenced by the interaction between the metal ion and the anion, and as a consequence, the reaction rate is dependent upon the anion (counterion, X-). As described above, this is the case that has been certified in the present study.23
The signal shift of H8 proton on the macrocyclic ring of the Li+ complex of type 4 would be arisen from the difference in the interaction between the anion, the metal ion, and the nitrogen atoms of four pyridine moieties, providing the change of electron density on the macrocyclic ring.
Eventually, the experimental findings indicated that the major origin of unusual complexation reaction by macrocycle 3b would come from the unique property of macrocycle 3b, that is a strong intramolecular hydrogen bond in the macrocyclic ring and the highly strained macrocyclic ring by hybrid structure of nonplanar A and planar B moieties. These high functionalities that were found for the nitrogen heterocycle- containing macrocycles developed by us would fortify their macrocycle chemistry capabilities and specifically, quintessential unsymmetry of the macrocyclic ring will mark future functionalized macrocycles for diverse uses.
EXPERIMENTAL
Chemicals. The reagents such as LiCl, LiBr, LiI, LiClO4, and LiOH were purchased from Merck Ltd., Wako Pure Chemical Industries Ltd., and Kanto Chemical Co. Other reagents for syntheses of macrocycles were also obtained from these companies and used as received. Dry and pure deuterio-dichloromethane was required for the 1H-NMR measurements of the complexation reactions of macrocycle 3b with lithium salts, and then deuterio-dichloromethane (CH2Cl2-d2) sealed in a glass tube (1mL) was purchased from Aldrich Co. Since dry diethyl ether and toluene were required to carry out the syntheses of macrocycles, these solvents were distilled from sodium under an argon atmosphere. These solvents were stored over 4 A molecular sieves under an argon atmosphere. N,N-dimethylformamide (DMF) used for monoalkylation of macrocycle was dried over 4 A molecular sieves and distilled under vacuum prior to use. Other solvents (methanol, hexane, and chloroform) were obtained commercially and purified according to standard methods. For column chromatography to purify macrocycle 3, Wakogel (silica-gel) C-300 (particle size 45-75 µm) was used and this silica gel was packed carefully into 1m glass tube. Chloroform and hexane for chromatography were of reagent grade and were used without further purification.
1H-NMR Measurements. To explore the processes of the complexation reactions of macrocycle 3b with lithium salts, the 1H-NMR spectral changes of the CH2Cl2-d2 solutions including macrocycle 3b and lithium salt (Li+X-) were measured with time. A JEOL JNM GX-270 spectrometer (270 MHz) was employed for these measurements and the spectra were recorded at 298 K with SiMe4 as an internal reference. The samplings for this measurement were carried out under a dry argon atmosphere, because lithium salts are very sensitive to the moisture and the complexation reactions are easy to be changed by the existence of water in the solution. When a known amount of lithium salt (5.78 x 10-1- 13.5 x 10-1 M) was added to the macrocycle 3b solution
(4.81 x 10-3 M) and this reaction mixture was kept at room temperature (298 K), the complex formed gave the 1H-NMR spectra that revealed several signals with relative area consistent with that expected for Li+complex of type 4; the new multi-peaks assigned to pyridine moieties appeared in the region between δ7.95 and 8.20 ppm except the case of LiOH. A single peak assigned to H8 proton on the macrocyclic ring appeared in the region betweenδ6.60 and 7.90 ppm. As the term of peak appearance and the positions depended on the anion (X-) of lithium salt (Li+X-), the 1H-NMR spectra of the dichloromethane-d2 solution containing macrocycle 3b and lithium salt were measured at regular intervals to monitor the spectral change with progress of complexation. As described above, excess amount of lithium salts (LiCl, LiBr, LiI, LiClO4, and LiOH) were used for this experiment. Each concentration of lithium salt used is described in captions of Figures 1 - 5. The transient signals obtained by the reaction of macrocycle 3b with LiBr were somewhat obscured by the exchange reaction between metal free macrocycle and its lithium salt. The properties of macrocycle 3b-Li+X- complexes were also examined by using 1H-NMR spectrum measurements.
Measurements of Reaction Rates by UV-Visible Spectroscopy. The reaction rates of macrocycle 3b with lithium salts were measured in homogeneous system. The data of these reaction rates were obtained on a Shimadzu UV 2200 spectrophotometer at room temperature in 1 cm quartz cell. An acetonitrile solution containing excess amount of lithium salts (0.2 M) was added to an acetonitrile solution of macrocycle 3b
(5.45 x 10-5 M), and the change of the peak intensity (462.5 nm) of macrocycle 3b was measured with time by UV-Visible spectrometer.22
Preparation and purification of macrocycle 3b. Macrocycle 3b was prepared by using the detailed procedure we have described previously.15 Pure macrocycle 3b was made by careful purification using column chromatography on silica-gel with chloroform-methanol system as an eluent. This procedure for each spectral measurement is very important to avoid complications from possible contamination of impurity.
Preparations of macrocycle 3b-Li+ X- complexes of type 4. To obtain pure Li+ complexes for the characterization, excess amount of lithium salt (5.78 x 10-1- 13.5 x 10-1 M) was added to the orange solution of macrocycle 3b in dry dichloromethane (4.81 x 10-3 M) under an argon atmosphere. Lithium salt which is present in excess reacts with macrocycle 3b more strongly to give its lithium complex. The dichloromethane solution including metal free macrocycle 3b shows orange color, but the dichloromethane solution of macrocycle 3b-Li+X- complex of type 4 is colorless. The orange color of the solution of reaction mixture disappears with the formation of macrocycle 3b-Li+X- complex. Thus, the formation process of Li+ complex was able to be monitored by the UV-Visible spectrum measurements. After confirming the completion of reaction by UV-Visible spectrum measurements, the solvent was reduced partially by rotary evaporator. After standing for overnight, the precipitates were obtained and were filtered. After drying under vacuum, the colorless solid was obtained as a pure lithium complex. The 1H-NMR spectra of macrocycle 3b-Li+complexes are the same as the previous results which had been assigned to the Li+complex of type 4.15
Macrocycle 3b-Li+ Cl- complex. 1H-NMR (CD2Cl2, 298K) δ 7.96-8.08 (m),
8.10(d), 8.14(d), 7.66(s) ; UV-Vis (CH2Cl2) λ max (ε) 295 nm (8796), 244.5 (10469).
Macrocycle 3b-Li+ Br- complex. 1H-NMR (CD2Cl2, 298K) δ 7.97-8.11 (m),
8.12 (d), 8.15(d), 7.79(s) ; UV-Vis (CH2Cl2) λ max (ε) 299 nm (10906), 243 (11781).
Macrocycle 3b-Li+ I- complex. 1H-NMR (CD2Cl2, 298K) δ 7.99-8.12 (m),
8.15 (d), 8.17(d), 7.87(s); UV-Vis (CH2Cl2) λ max (ε) 294 nm (24095), 237 (19653).
Macrocycle 3b-Li+ ClO4- complex. 1H-NMR (CD2Cl2, 298K) δ 8.03-8.14 (m),
8.15 (d), 8.17(d), 6.61(s); UV-Vis (CH2Cl2) λ max (ε) 295 (5436), 245 (5626).
References
1. (a) R. M. Izatt and J. J. Christensen, ‘Synthetic Multidentate Macrocyclic Compounds;
Academic press: New York, 197; (b) F. Vogtle, Ed. Host Gust Chemistry II; Springer-Verlag: Berlin-Heidelberg, 1982; (c) Chemical & Engineering News, 2003, 81, 45.
2. (a) C. O. Paul-Roth, J. M. Lehn, J. Gulhem, and C. Pascard, Helv. Chim. Acta, 1995, 78, 1895; CrossRef (b) N. Sabatini, M. Guardigli, and J. M. Lehn, Coord. Chem. Rev., 1993, 123, 201; CrossRef (c) J. M. Lehn and C. O. Paul-Roth, Helv. Chim. Acta, 1991, 74, 572; CrossRef (d) C. Kaes, A. Katz, and M. W. Hosseini, Chem. Rev., 2000, 100, 3553; CrossRef (e) C. Kaes, M. W. Hosseini, E. F. Rickard, B. W. Skelton, and A. H. White, Angew. Chem. Int. Ed., 1998, 37, 920; CrossRef (f) C. Kaes, M. W. Hosseini, A. De Cian, and J. Fischer, J. Chem. Soc., Chem. Commun., 1997, 2229; (g) C. Glaup, M. C. Carrie, J. Azema, and C. Picard, Tetrahedron Lett., 1998, 39, 1573; CrossRef (h) V. Alexander, Chem. Rev., 1995, 95, 273; CrossRef (i) D. S. Kumar and V. Alexander, Inorg. Chim. Acta, 1995, 238, 63; CrossRef (j) J. Beech, P. J. Cragg, and M. G. B. Drew, J. Chem. Soc. Dalton Trans., 1994, 719; CrossRef (k) C. Wendelstorf and R. Kramer, Angew. Chem., Int. Ed., 1997, 36, 2791. CrossRef
3. (a) A. P. De Silva, H. Q. N. Gunaratne, T. Gunnlaugsson, A. J. M. Huxley, C. P. McCoy, J. T. Rademacher, and T. E. Rice, Chem. Rev., 1997, 97, 1515; CrossRef (b) J. Canales, J. Ramirez, G. Estiu, and J. Costamagna, Polyhedron, 2000, 19, 2373; CrossRef (c) R. B. Hopkin, J. S. Albert, D. V. Engen, and A. D. Hamilton, Bioorg. Med. Chem., 1996, 4, 1121. CrossRef
4. (a) F. R. Fronczek, P. J. Schilling, S. F. Walkins, V. K. Majestic, and G. R. Newcome, Inorg. Chim. Acta, 1996, 246, 119; CrossRef (b) G. R. Newcome, S. Pappalardo, and V. K. Gupta, J. Org. Chem., 1983, 48, 4848; CrossRef (c) G. R. Newcome and D. K. Kohli, J. Chem. Soc.,Chem. Commun., 1980, 9; CrossRef (d) G. R. Newcome, J. D. Sayer, J. M. Roper, and D. C. Hager, Chem. Rev., 1977, 77, 513. CrossRef
5. S. Ogawa, T. Uchida, T. Uchiya, T. Hirano, M. Saburi, and Y. Uchida, J. Chem. Soc., Perkin Trans.1, 1990, 16486.
6. S. Ogawa, R. Narushima, and Y. Arai, J. Am. Chem. Soc., 1984, 106, 5750. CrossRef
7. S. Ogawa and S. Tsuchiya, Chem. Lett., 1996, 709. CrossRef
8. K. Takano, A. Furuhama, S. Ogawa, and S. Tsuchiya, J. Chem. Soc., Perkin Trans. 2, 1999, 106.
9. A. Furuhama, A. Takano, S. Ogawa, and S. Tsuchiya, Bull. Chem. Soc. Jpn., 2001, 74, 1241. CrossRef
10. M. Seno, S. Tsuchiya, and S. Ogawa, J. Am. Chem. Soc., 1977, 99, 3014. CrossRef
11. U. Olsher, J. Am. Chem. Soc., 1982, 104, 4006. CrossRef
12. S. Sawada, H. Torii, T. Osakai, and T. Kimoto, Anal. Chem., 1998, 70, 4286. CrossRef
13. Y. Habata, H. Ugajin, and S. Akabori, J. Org. Chem., 1994, 59, 6766. CrossRef
14. Y. Inoue and G. W. Gokel, Cation Binding by Macrocycle; Marcel Dekker: New York, 1990.
15. R. Ibrahim, S. Tsuchiya, and S. Ogawa, J. Am. Chem. Soc., 2000, 122, 12174. CrossRef
16. Chem. & Eng. News, 2002, 80, 25.
17. S. Tsuchiya and M. Seno, J. Phys. Chem., 1994, 98, 13680. CrossRef
18. H. Dugas, Bioorganic Chemistry Frontiers; Springer-Verlag: New York, 1990.
19. J. J. Christensen, D. J. Eatough and R. M. Izatt, Chem. Rev., 1974, 74, 351. CrossRef
20. S. Tsuchiya, Y. Nakatani, R. Ibrahim and S. Ogawa, J. Am. Chem. Soc., 2002, 124, 4936. CrossRef
21. The reactions of macrocycle 3b with lithium salts in acetonitrile were carried out at the same concentration (LiI, LiBr, and LiClO4), and the UV-Visible spectra of these products indicated the formation of the complex of type 4. The reaction rate of LiCl was not obtained because of low solubility of LiCl in acetonitrile. Since the complexation reactions in acetonitrile-d3 are fast, the measurements of spectral changes by 1H-NMR using acetonitrile-d3 as a solvent are unsuitable for this study.
22. The solubility of lithium salt would be one of the factors by which the reaction rate is influenced. However, the UV-Visible experiments using homogeneous acetonitrile system described in above section provided an unequivocal fact that the interaction between macrocycle, lithium cation, and anion plays a major role in determining the reaction rate.
23. Another factor that possibly contributes to the faster reaction rate may be found in the solubility effect.22 In addition, other candidates would be the polarity effect of solvent, affording the stabilization effect by the dipole-dipole interaction. .
24. Since the reactions of macrocycle 3b with lithium salts in methanol afford the lithium complex of type 5 shown in Scheme 4, a large amount of methanol can not be added to the reaction solution of dichloromethane.
25. Another reason for the use of dichloromethane is that the stable Li+ complex of type 4 is formed readily.