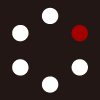
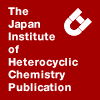
HETEROCYCLES
An International Journal for Reviews and Communications in Heterocyclic ChemistryWeb Edition ISSN: 1881-0942
Published online by The Japan Institute of Heterocyclic Chemistry
e-Journal
Full Text HTML
Received, 3rd June, 2009, Accepted, 1st July, 2009, Published online, 2nd July, 2009.
DOI: 10.3987/REV-09-SR(S)3
■ Pharmaceutical Studies on Vitamin D Derivatives and Practical Syntheses of Six Commercially Available Vitamin D Derivatives That Contribute to Current Clinical Practice
Noboru Kubodera*
Chugai Pharmaceutical Company, Ltd., 2-1-1, Nihonbashi-Muromachi, Chuo-ku, Tokyo 103-8324, Japan
Abstract
Three milestones exist in vitamin D research from the perspective of pharmaceutical development. The article first discusses the various milestones outlining vitamin D and derivatives in terms of pharmaceutical applications. Several active vitamin D derivatives based on the findings during the third milestone are now commercially available and contributing to current clinical practice. The paper also reviews the practical syntheses of six derivatives, maxacalcitol, calcipotriol, tacalcitol, paricalcitol, doxercalciferol, and falecalcitriol, as well as summarizes future prospects for further drug development.CONTENTS
1. Introduction
2. Pharmaceutical studies
2-1. Discovery of the physiological significance of vitamin D in the first milestone
2-2. Discovery and clinical application of active vitamin D in the second milestone
2-3. Drug discovery research on active vitamin D derivatives in the third milestone
3. Practical syntheses of six commercially available derivatives
3-1. Maxacalcitol
3-2. Calcipotriol
3-3. Tacalcitol
3-4. Paricalcitol
3-5. Doxercalciferol
3-6. Falecalcitriol
4. Future prospects for further drug development
5. Acknowledgments
6. References and notes
1. INTRODUCTION
In vitamin D research, there are three milestones from the perspective of pharmaceutical development. The first represents the early stages of vitamin D research and involves the discovery of vitamin D and its physiological significance as an anti-rachtic factor. The second is the elucidation of the vitamin D activation pathway in the body and the discovery of the biologically active metabolites. This led to a remarkable growth of knowledge in the pathophysiology of vitamin D, paving the way for clinical applications of active vitamin D and prodrugs. The third is characterized by the discovery of the differentiation-inducing properties of active vitamin D. This showed the diversity of the physiological actions of active vitamin D, which initially had been thought only to contribute to bone and calcium metabolism. Because these physiological actions were clinically useful, the third discovery revealed opportunities for the development of drugs appropriate to specific purposes by synthesizing derivatives to separate biological effects. Several active vitamin D derivatives based on the findings during the third milestone are now commercially available and contributing to current clinical practice. This article discusses the various milestones outlining vitamin D and derivatives in terms of pharmaceutical applications. The paper also deals with practical syntheses of six derivatives developed during the third milestone, maxacalcitol, calcipotriol, tacalcitol, paricalcitol, doxercalciferol, and falecalcitriol, as well as summarizes future prospects for further drug development.
2. PHARMACEUTICAL STUDIES
In this chapter, the characteristic aspects in each milestone are introduced especially in respect of pharmaceutical applications of vitamin D derivatives.
2-1. DISCOVERY OF THE PHYSIOLOGICAL SIGNIFICANCE OF VITMIN D IN THE FIRST MILESTONE
Rachitis, a bone disease, has been common since ancient times, particularly in Europe. In 1925, McCollum and colleagues found that rachitis was caused by a nutritional disorder, specifically involving vitamin D. Around the same time, Steenbock and colleagues discovered that anti-rachitis substances are generated when food is exposed to ultraviolet rays. In addition, Hess, Askew, Windaus, and colleagues found that vitamin D2 (generic name: ergocalciferol, 1) and vitamin D3 (generic name: cholecalciferol, 2) are created when ergosterol and 7-dehydrocholesterol are irradiated with ultraviolet rays. The structures of vitamin D2 (1) and vitamin D3 (2) were elucidated for the first time. It was also confirmed that their deficiencies cause rachitis, proving that vitamin D plays an important role for bone and calcium metabolism.
Although it was indeed a significant discovery that marked the first milestone, nothing yet was known at the time about the mechanism of vitamin D and its involvement in such a metabolic process. Vitamin D usually refers to vitamin D2 (1) and/or vitamin D3 (2) (Figure 1).1,2
2-2. DISCOVERY AND CLINICAL APPLICATION OF ACTIVE VITAMIN D IN THE SECOND MILESTONE
The second milestone was marked by the discovery of active vitamin D by DeLuca, Kodicek, and colleagues. The results of their studies suggest that physiologically inactive vitamin D is first hydroxylated to 25-hydroxyvitamin D in the liver, then further hydroxylated in the kidney to 1,25-dihydroxyvitamin D, which is physiologically active vitamin D. It was also discovered that various physiological adjustments are involved in hydroxylation in the kidney, bringing dramatic progress to the pathophysiology of vitamin D.
They also revealed deficiencies in hydroxylation of 25-hydroxyvitamin D in patients with kidney disorders, resulting in vitamin D deficiency caused by poor vitamin D activation. Clinical applications of 1,25-dihydroxyvitamin D3 (3) or its prodrug, 1α-hydroxyvitamin D3 (4), were introduced in an attempt to make up for the deficiency. It was also confirmed that 1α-hydroxyvitamin D3 (4) is hydroxylated at the 25-position to 1,25-dihydroxyvitamin D3 (3) in the liver. In Japan, 1α-hydroxyvitamin D3 (4) was
released under the generic name alfacalcidol (trade names: Alfarol, Onealfa, etc.) as a treatment for renal bone diseases or hypoparathyroidism. Its indication was later expanded to include osteoporosis. As a pharmaceutical, 1,25-dihydroxyvitamin D3 (3) was also released under the generic name calcitriol (trade names: Rocalltrol, etc.) in Japan, the US and Europe. Consequently, much enthusiasm resulted in studying clinical applications of active vitamin D after the second milestone (Figure 1).3
2-3. DRUG DISCOVERY RESEARCH ON ACTIVE VITAMIN D DERIVATIVES IN THE THIRD MILESTONE
Alfacalcidol (4) was first introduced to the Japanese pharmaceutical market in 1981. Following the elucidation of the activation pathway of vitamin D and the development of pharmaceutical drugs for supplementing vitamin D deficiency, research and development toward vitamin D was generally believed to have achieved its goal. Coinciding with the market release of alfacalcidol (4), Suda, Abe, and colleagues made the momentous discovery that calcitriol (3) differentiates myelogenous leukemia cells to normal macrophages in mice and human, opening a new page in the history of vitamin D research.4 At the same time, it was also discovered that vitamin D receptors exist in various organs and tissues in the body.5 In addition to the exiting research finding that active vitamin D is only involved in bone and calcium metabolism, studies revealed that this substance also contributes to numerous other physiological reactions, such as differentiation-inducing activity, cell growth inhibition, and immunomodulation. Furthermore, there were an increasing number of patients given alfacalcidol (4) for the treatment of vitamin D deficiency and hypocalcemia, whose prognosis for rheumatoid arthritis (RA) and psoriasis vulgaris (Pso), a therapy-resistant dermal disease, was clinically promising. It was found, however, that the increase in alfacalcidol (4) dosage to enhance the therapeutic effects on these comorbidities could not achieve the target effect but only produced a side effect, blood calcium increase. Although it was not known why alfacalcidol (4) worked for Pso and RA, there were an increasing number of cases discovered in fundamental research showing the involvement of the differentiation-inducing actions of the calcitriol (3). Around the world, new derivatives were actively synthesized in drug development research that focused on separating the biological actions between in vitro differentiation-inducing effects (main effect index) and in vivo calcium actions (side effect index).6 The title of this chapter, pharmaceutical studies, culminates in drug discovery as the third milestone.
The author also started early on in this research and invented maxacalcitol (5), a derivative whose side chain is modified to have potent differentiation-inducing effects but weak blood calcium raising effects. Maxacalcitol (5) was released onto the market as an injection for treating secondary hyperparathyroidism (2HPT) and as an ointment for Pso and is now used in clinical practice. Please refer to the relevant study for details (Figure 2).7,8
On the other hand, a derivative with the opposite balance of biological actions to maxacalcitol (5) was found among A-ring modified candidates. In other words, modification of the A-ring led to the discovery of a derivative with weak in vitro differentiation induction properties but potent calcemic actions in vivo. Assuming that potent calcemic activity is accompanied by an intense effect on bone, such effects of a derivative were examined using osteoporosis model animals, which would show greater effects on bone than calcitriol (3) and alfacalcidol (4). The derivative is eldecalcitol (developing code: ED-71 6). A phase 3 clinical trial with eldecalcitol (6) has been completed with very good results as an oral medication for treating osteoporosis. The hope is that it will, in the near future, help osteoporosis patients as a characteristically new active vitamin D derivative (Figure 2).7,8
Figure 3 shows active vitamin D derivatives marketed, namely calcipotriol (7), tacalcitol (8), paricalcitol (9), doxercalciferol (10), and falecalcitriol (11). Most are derivatives that have a similar balance of biological action to maxacalcitol (5), and are used mainly to treat 2HPT or Pso. Although scientists have reportedly attempted to research and develop active vitamin D derivatives for the treatment of cancer and Alzheimer’s disease, none have yet been released onto the market (Figure 3).
3. PRACTICAL SYNTHESES OF SIX COMMERCIALLY AVAILABLE DERIVATIVES
In this chapter, the practical syntheses of six vitamin D derivatives found in the third milestone that are currently contributing to clinical treatment are summarized. Although the detailed and industrial syntheses of each derivative are not necessarily disclosed in the literature and patents, the most practical syntheses of these derivatives are summarized below from the literature. The common synthetic feature for each derivative is based on a linear synthesis with a variety of starting materials used, e. g. dehydroepiandrosterone (12) for maxacalcitol (5), elgocalciferol (1) for calcipotriol (7), fucosterol (31) for tacalcitol (8), 25-hydroxyergocalciferol (40) for paricalcitol (9), ergosterol (48) for doxercalciferol (10), and cholenic acid (56) for falecalcitriol (11). Convergent methodologies have not been employed for the practical production of each derivative.
3-1. MAXACALCITOL
Maxacalcitol (5), developed by Chugai Pharmaceutical Co., Ltd, was launched as the brand name of Oxarol injection for the treatment of 2HPT in 2000 and Oxarol ointment for Pso in 2001, both in Japan.
Detailed process development for the industrial synthesis of maxacalcitol (5) is extensively described in the literature by the Chugai group.9 The hydroxyl groups in 1α-hydroxydehydroepiandrosterone (13), prepared from dehydroepiandrosterone (12) by known microbial oxidation,10 were protected as their tert-butyldimethylsilyl (TBS) ether in 74% yield. Wittig reaction of bis-TBS ether 14 gave the stereoselective formation of Z-olefin 15 in 90% yield. Transformation of Z-olefin 15 into secondary alcohol 16 was carried out in regio- and stereoselective manner by hydroboration with 9-BBN and oxidation in 90 % yield. After several attempts,11-13 the side chain introduction of maxacalcitol (5) was successfully achieved in almost quantitative yield (99%) by ether formation using the epoxy bromide 17 and subsequent epoxide reduction with L-Selectride. The 5,7-diene formation by bromination with NBS/AIBN and dehydrobromination with γ-collidine afforded 19 in 43% yield. After deprotection of the TBS ether in 19, photolysis of penultimate intermediate 20 in THF by irradiation with a high-pressure mercury lamp at 10 °C and subsequent thermal isomerization in THF 25 °C furnished maxacalcitol (5) in 16% yield. The present method allowed the production of the key intermediate 19 in 26% from 13 on a kilogram scale conversion without formation of any undesired byproducts employing neither difficult reaction conditions nor chromatographic purification during the conversions involved (Scheme 1).
3-2. CALCIPOTRIOL
Calcipotriol (7), developed by Leo Pharmaceutic Products, was launched with sales name of Dovonex for the treatment of Pso in 1991 in Europe. The key structural feature of calcipotriol (7) is the C-22,23 unsaturated and 24(S)-hydroxylated side chain with a cyclopropyl substituent. It is reported that biological effects of an epimer of calcipotriol (7) at C-24 on cell proliferation and differentiation are at least 10 times less than 7.14
A detailed synthetic route to calcipotriol (7) starting from ergocalciferol (1) is delineated in a literature report by Calverley14 The reactions transforming 1 to 1α-hydroxylated derivative 24 are essentially described by Hesse’s group.15 Thus, ergocalciferol (1) was converted to epimeric SO2-adducts 21 by simply dissolving 1 in SO2, which was protected as its TBS ether 22 in 94% yield from 1. Cheletropic extraction of SO2 in refluxing EtOH containing NaHCO3 converted epimeric 21 to the single 5(E)-derivative 23, which was submitted to a selenide mediated allylic hydroxylation reaction using SeO2 and N-methylmorpholine N-oxide (NMO) to give 1α-hydroxylated product 24 in 35% yield from 1 after TBS protection. The process was accompanied by formation of the 1β-isomer in 7% yield. Treatment of 24 with liquid SO2 quantitatively afforded an epimeric mixture (3:1) of SO2-adducts 25. Ozonolysis of 25 provided aldehyde 26 in 81% yield, from which thermal cheletropic extraction of SO2 in the presence of NaHCO3 gave rise to key intermediate 27 in 90% yield after separation from the C-20 epimer (5%). Horner-Emmons reaction of 27 with cyclopropylcarbonylmethylenetriphenyl- phosphorane led to pure enone 28 in 63% yield. Reduction of 28 with NaBH4 gave the 24(S)-alcohol 29 in 39% yield accompanying with 24(R)-alcohol (39%) and C22,23 saturated epimeric alcohol (13%), which were separated by chromatography and recrystallization. Photoisomerization of 29 in toluene using anthracene as a triplet sensitizer was successfully carried out to give 30 in 92% yield, which was finally converted to calcipotriol (7) by deprotection of TBS groups (Scheme 2).
3-3. TACALCITOL
Tacalcitol (8), developed by Teijin Institute for Bio-Medical Research, was launched with sales name of Bonalfa for the treatment of Pso in 1993 in Japan. The structural feature of tacalcitol (8) is the 24(R)-hydroxylated side chain and 24(S)-isomer of 8 which is also reported to show weak biological activities compared to 8.16 Note, similar relationships to calcipotriol (7) and C-24 epimer concerning the configuration at the C-24 hydroxylated center and biological response were observed.17
Synthetic route to tacalcitol (8) starting from fucosterol (31) is described in the literature by Teijin’s and Ikekawa’s groups.18 24-Oxocholesterol (32), which is readily obtained from fucosterol (31) by ozonolysis,19 was oxidized with 3.3 equiv. of DDQ to 1,4,6-trien-3,24-dione 33 in 52% yield. Treatment of 33 with alkaline H2O2 gave α-epoxide 34 in 71% yield, which was reduced with Li-NH4Cl in liquid NH3-THF yielding epimeric triol 35 in 68% yield. When 35 was treated with 2.5 equiv. of benzoyl chloride, three products were obtained, 24(R)-dibenzoate 37, 24(S)-dibenzoate, and epimeric tribenzoate. These products can be separated by chromatography. 24(R)-Dibenzoate 37 was sequentially treated by acetylation, bromination with NBS, dehydrobromination with trimethyl phosphate and 4-pheny-1,2,4-triazoline-3,5-dione (PTAD) to afford the PTAD adduct 38. LiAlH4 reduction of 38 gave 5,7-diene 39, which was converted to tacalcitol (8) by irradiation with a medium-pressure mercury lamp causing subsequent thermal isomerization in 12% yield (Scheme 3).
3-4. PARICALCITOL
Paricalcitol (9), developed by Abbott Labs., was launched with sales name of Zemplar for the treatment of 2HPT in 1998 in US. Structural features of paricalcitol (9) lack the exocyclic C-19 carbon and has
an active vitamin D2 side chain, namely 1,25-dihydroxy-19-norvitamin D2. It is reported that although paricalcitol (9) is approximately 10 times less calcemic than calcitriol (3), however, it is equipotent to 3 in suppressing parathyroid hormone (PTH) as anti-2HPT agent.20,21
The synthetic route to paricalcitol (9) is outlined in a patent by DeLuca’s group, although detailed yields are not fully disclosed.22 The starting material, 25-hydroxyergocalciferol (40),23 was converted to 3,5-cyclovitamin D2 derivative 41 by buffered methanolysis after tosylation of 40.24 Allylic oxidation of 41 with SeO2 and t-BuOOH gave 1α-hydroxylated derivative 42 after acetylation,24 which was treated with OsO4 in pyridine to give diol 43.25 Oxidative cleavage of 1,2-diol moiety in 43 with periodate furnished ketone 44.25 The carbonyl function in 44 was reduced to alcohol 45, which was transformed to a mixture of 3-acetoxy-1α-hydroxy derivative 46 and 1α-acetoxy-3-hydroxy derivative 47 by mesylate formation, LiAlH4 reduction and acetolysis reaction, although detailed reaction conditions are not described. Subsequent hydrolysis of acetates both in 46 and 47 gave rise to paricalcitol (9) as a sole product (Scheme 4).
3-5. DOXERCALCIFEROL
Doxercalciferol (1α-hydroxyvitamin D2 10), developed by Bone Care International, was launched with sales name of Hectorol for the treatment of 2HPT in 1999 in US. Although doxercalciferol (10) is a prodrug of an active vitamin D2 (1,25-dihydroxyvitamin D2) and, like 1,25-dihydroxyvitamin D3 (calcitriol 3) which is the counterpart of 1α-hydroxyvitamin D3 (alfacalcidol 4), must be activated in vivo,26,27 the mechanism, however, for biological activity is much less understood for doxercalciferol (10) than alfacalcidol (4).27
The ten-step synthetic route to doxercalciferol (10) starting from ergosterol (48) is well documented in the literature by DeLuca’s group.28 Thus, Oppenauer oxidation of ergosterol (48) gave 4,7,22-trien-3-one 49 in 77% yield, which was followed by acid catalyzed double bond conjugation according to the procedure outlined by Shepherd’s group.29 This sequence afforded 4,6,22-trien-3-one 50 in 80% yield. SeO2 oxidation of 50 led to 1,4,6,22-tetraen-3-one 51 in 30% yield. Upon treatment of 51 with alkaline H2O2, α-epoxide 52 was obtained in 77% yield. Reduction of 52 with Li-NH4Cl in liquid NH3-THF furnished, after chromatography and crystallization, a 30% yield of diol 53, which, via its diacetate 54 (54% yield), was converted to the required 5,7-diene 55 by a bromination- dehydrobromination procedure. Irradiation of 55 by a high-pressure mercury lamp followed by thermal isomerization by refluxing in EtOH and diacetate hydrolysis gave doxercalciferol (10) in 16% yield (Scheme 5).
3-6. FALECALCITRIOL
Falecalcitriol (11), developed by Taisho Pharmaceutical Co., Ltd, and Sumitomo Pharmaceuticals Co., Ltd, was launched with the sales name of Hornel (Taisho) and Fulstan (Sumitomo) for the treatment of 2HPT in 2001 in Japan. Falecalcitriol (11) is a fluorinated derivative of calcitriol (3) at C-26 and C-27 and displays higher activity than 3 in vivo due to its slower metabolism compared to 3.30-32
The synthetic route to falecalcitriol (11) starting from cholenic acid (56) is well-summarized in the literature by Ikekawa’s group.33 Methyl cholenate (57) was oxidized with DDQ to give 1,4,6-trien-3-one 58 in 55% yield, which was converted to α-epoxide 59 by the treatment with alkaline H2O2 in 80% yield. Reductive cleavage of α-epoxide 59 by Li-NH4Cl in liquid NH3-THF furnished triol 60 (65% yield). This intermediate was then transformed to bromodiacetate 61 in 38% overall yield by a series of reactions that involve tritylation of the side-chain hydroxyl moiety, acetylation of the 1,3-diol, hydrolysis of trityl group, and tosylation of the resulting hydroxyl moiety followed by bromination. Treatment of 61 with PhSO2Na in DMF afforded sulfone 62 in 92% yield. After conversion of the acetyl protecting groups in 62 to the bis-triethylsilyl (TES) ether 63 (57% yield), lithiation of the sulfone in 63 with LDA followed by introduction of an excess of hexafluoroacetone gas gave adduct 64 in 74% yield as a stereoisomeric mixture.34 Acid hydrolysis and desulfonylation of 64 with sodium amalgam (Na-Hg) in the presence of Na2HPO4 gave hexafluoride 65 in 72% yield. After acetylation (98%), triacetate 66 was brominated with NBS and then dehydrobrominated with γ-collidine to afford 5,7-diene 67 in 25% yield. The 5,7-diene 67 was irradiated with a medium-pressure mercury lamp, thermally isomerized by refluxing in benzene/EtOH and then hydrolyzed to falecalcitriol (11) in high yield (90%) (Scheme 6).
4. FUTURE PROSPECTS FOR FURTHER DRUG DEVELOPMENT
Since the beginning, vitamin D studies by scientists, including the author, have focused much attention to calcitriol (3) and alfacalcidol (4), based on the recognition of 3 as the biologically active metabolite. In retrospect, perhaps too much attention has been paid in this regard since in the body, there are many metabolites of vitamin D whose roles are yet unknown. They may have physiological actions that maintain homeostasis. As discussed in the previous section, the third milestone focused on the in vitro main effect and the in vivo side effect as indices, in which the author experienced the struggle of having to select indications for treatment. With active vitamin D derivatives, calcemic action involving increase in blood calcium level is the only side effect observed at all times. If the main effects can be seen in model animals with specific diseases whose blood calcium is monitored in the next generation of drug discovery research, it will make clinical application prospects much more promising.
Over the past several years, research data suggesting an association of active vitamin D with various diseases, such as cancer and diabetes, have been reported. In conclusion, the future for vitamin D drug discovery is very promising. The various findings accumulated throughout the third milestone, drug discovery activities, will likely lead to greater in-depth molecular understanding and the development of an array of new drugs in this field.7,8
5. ACKNOWLEDGMENTS
The author would like to express his sincere appreciation to Professor David A. Horne of the Department of Molecular Medicine, Beckman Research Institute at City of Hope for helpful suggestions and reading of the manuscript.
References
1. H. F. DeLuca, ‘Historical overview’ Vitamin D, ed. by D. Feldman, F. H. Glorieux, and J. P. Pike, Academic Press, San Diego, 1997, pp. 3-11.
2. H. F. DeLuca, ‘Historical perspective’ Vitamin D Second Edition, ed. by D. Feldman, J. W. Pike, and F. H. Glorieux, Elsevier Academic Press, Burlington, 2005, pp. 3-12. CrossRef
3. R. L. Horst, T. A. Reinhardt, and G. S. Reddy, ‘Vitamin D metabolism’ Vitamin D Second Edition, ed. by D. Feldman, J. W. Pike, and F. H. Glorieux, Elsevier Academic Press, Burlington, 2005, pp. 15-36.
4. E. Abe, C. Miyaura, H. Sakagami, M. Takeda, K. Konno, T. Yamazaki, S. Yoshiki, and T. Suda, Proc. Natl. Acad. Sci. USA, 1981, 78, 4990. CrossRef
5. J. W. Pike and N. K. Shevde, ‘The vitamin D receptor’ Vitamin D Second Edition, ed. by D. Feldman, J. W. Pike, and F. H. Glorieux, Elsevier Academic Press, Burlington, 2005, pp. 167-191.
6. G. H. Posner and M. Kahraman, ‘Overview: rational design of 1α,25-dihydroxyvitamin D3 analogs (deltanoids)’ Vitamin D Second Edition, ed. by D. Feldman, J. W. Pike, and F. H. Glorieux, Elsevier Academic Press, Burlington, 2005, pp. 1405-1422.
7. N. Kubodera, J. Syn. Org. Chem. Jpn, 2005, 63, 728.
8. N. Kubodera, Curr. Bioactive Compds, 2006, 2, 301.
9. H. Shimizu, K. Shimizu, N. Kubodera, T. Mikami, K. Tsuzaki, H. Suwa, K. Harada, A. Hiraide, M. Shimizu, K. Koyama, Y. Ichikawa, D. Hirasawa, Y. Kito, M. Kobayashi, M. Kigawa, M. Kato, T. Kozono, H. Tanaka, M. Tanabe, M. Iguchi, and M. Yoshida, Org. Process Res. Dev., 2005, 9, 278. CrossRef
10. R. M. Dodson, A. H. Goldkamp, and R. D. Muir, J. Am. Chem. Soc., 1960, 82, 4026. CrossRef
11. T. Mikami, T. Iwaoka, M. Kato, H. Watanabe, and N. Kubodera, Synth. Commun., 1997, 27, 2363. CrossRef
12. H. Shimizu, K. Shimizu, N. Kubodera, K. Yakushijin, and D. A. Horne, Tetrahedron Lett., 2004, 45, 1347. CrossRef
13. H. Shimizu, K. Shimizu, N. Kubodera, K. Yakushijin, and D. A. Horne, Heterocycles, 2004, 63, 1335. CrossRef
14. M. J. Calverley, Tetrahedron, 1987, 43, 4609. CrossRef
15. D. R. Andrews, D. H. R. Barton, K. P. Cheng, J.-P. Finet, R. H. Hesse, G. Johnson, and M. M. Pechet, J. Org. Chem., 1986, 51, 1637. CrossRef
16. N. Ikekawa and T. Takeshita, J. Syn. Org. Chem. Jpn, 1979, 37, 809.
17. cf. N. Kubodera, H. Watanabe, K. Miyamoto, M. Matsumoto, S. Matsuoka, and T. Kawanishi, Chem. Pharm. Bull., 1993, 41, 1659.
18. M. Morisaki, N. Koizumi, N. Ikekawa, T. Takeshita, and S. Ishimoto, J. Chem. Soc., Perkin Trans. 1, 1975, 1421. CrossRef
19. M. Morisaki, J. R. Lightbourn, N. Ikekawa, and T. Takeshita, Chem. Pharm. Bull., 1973, 21, 2568.
20. E. Slatopolsky, J. Finch, C. Ritter, M. Denda, J. Morrissey, A. Brown, and H. DeLuca, Am. J. Kidney Dis., 1995, 26, 852. CrossRef
21. F. Takahashi, J. L. Finch, M. Denda, A. S. Dusso, A. J. Brown, and E. Slatopolsky, Am. J. Kidney Dis., 1997, 30, 105. CrossRef
22. H. F. DeLuca, H. K. Schnoes, K. L. Perlman, R. R. Sicinski, and P. J. Martin, EP, 1990, 0387077.
23. cf. Sigma-Aldrich Co., and Hydragon Pharma Ltd., have announced that 25-hydroxyergocalciferol (40) has been commercially available.
24. H. E. Paaren, H. F. DeLuca, and H. K. Schnoes, J. Org. Chem., 1980, 45, 3253. CrossRef
25. H. E. Paaren, H. K. Schnoes, and H. F. DeLuca, J. Org. Chem., 1983, 48, 3819. CrossRef
26. H.-Y. P. Lam, H. K. Schnoes, and H. F. DeLuca, Science, 1974, 186, 1038. CrossRef
27. A. J. Brown, A. S. Dusso, and E. Slatopolsky, Nephrol. Dial. Transplant., 2002, 17, 10. CrossRef
28. H.-Y. Lam, H. K. Schnoes, and H. F. DeLuca, Steroids, 1977, 30, 671. CrossRef
29. D. A. Shepherd, R. A. Donia, J. A. Campbell, B. A. Johnson, R. P. Holysz, G. Slomp, Jr., J. E. Stafford, R. L. Pederson, and A. C. Ott, J. Am. Chem. Soc., 1955, 77, 1212. CrossRef
30. P. A. Stern, Y. Tanaka, H. F. DeLuca, N. Ikekawa, and Y. Kobayashi, Mol. Pharmacol., 1981, 20, 460.
31. Y. Tanaka, H. F. DeLuca, Y. Kobayashi, and N. Ikekawa, Arch. Biochem. Biophys., 1984, 229, 348. CrossRef
32. P. H. Stern, T. Mavreas, Y. Tanaka, H. F. DeLuca, N. Ikekawa, and Y. Kobayashi, J. Pharmacol. Exp. Ther., 1984, 229, 9.
33. Y. Kobayashi, T. Taguchi, S. Mitsuhashi, T. Eguchi, E. Ohshima, and N. Ikekawa, Chem. Pharm. Bull., 1982, 30, 4297.
34. cf. Y. Kobayashi, T. Taguchi, N. Kanuma, N. Ikekawa, and J. Oshida, J. Chem. Soc., Perkin Trans. 1, 1980, 459. CrossRef