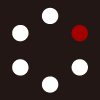
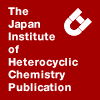
HETEROCYCLES
An International Journal for Reviews and Communications in Heterocyclic ChemistryWeb Edition ISSN: 1881-0942
Published online by The Japan Institute of Heterocyclic Chemistry
e-Journal
Full Text HTML
Received, 14th March, 2009, Accepted, 14th August, 2009, Published online, 18th August, 2009.
DOI: 10.3987/COM-09-11708
■ A Novel Molecular Cleft Based on Dioxocin Ring, Part I: Synthesis and Conformational Analysis
Bahareh Vafakish and Parviz Rashidi-Ranjbar*
School of Chemistry, College of Science, University of Tehran, Tehran 14155-6455, Iran
Abstract
The Molecular cleft 1 using 8-methyl-16H-dinaphtho[2,1-d:1,2-g][1,3]dioxocin-2,14-diol as a spacer was synthesized. Density functional calculations at B3LYP/6-31G level of theory indicates that 8-methyl-16H-dinaphtho[2,1-d:1,2-g][1,3]dioxocin, the central part of 1, could be populated in two stable conformations, i.e. BC and DB with difference in total energy of 0.12 kcal/mol. 1H-NMR spectroscopy, based on geminal coupling constants, shows that DB form is the most populated conformation of 1 in solution.INTRODUCTION
One of the most important features of supramolecular chemistry is molecular recognition, by which molecules selectively bind to form well-defined structures held together by intermolecular forces of noncovalent nature.1 The field emerged from this area is host-guest chemistry. The past decade has witnessed an explosive growth in research involving this area,2 and a large number of molecular receptors of varying sizes, shapes and functionalities have been synthesized and their interaction with guests have been assessed.3
The design of receptors is based on the fundamental molecular interactions exhibited by biological systems, particularly enzymes.4,5 Simple synthetic receptors with molecular pockets or cavities can act as models for complicated biological systems which are important in molecular recognition of substrates by enzymes.6,7 The study of artificial receptors should provide information about structure and stability of receptor-substrate complexes and non-covalent interactions responsible for their complex formation.
Molecular clefts,8-27 a subgroup of hosts, are generally the combination of a semirigid cavity to which tunable arms can be appended in predictable geometries. This makes a pocket suitable for binding molecules. The concept has been known for a while, and its applicability has been proven as a viable means of constructing a molecular receptor.28 This class of molecules was shown to form sandwich complexes with guests through noncovalent interactions.29-32
We have recently synthesized the molecular cleft 1 having 1,3-dioxocin ring as the nucleus of the spacer in the cleft (Scheme 1).
The experimental studies on the conformations of 1,3,2-dioxasilocines,33 1,3,2-diazaphosphocines,34 azocines35 and dioxocins36 have been carried out both in liquid and crystalline phases. The low energy conformations of boat-chair (BC), distorted boat (DB), twist (T), twist-boat (TB) and boat-boat (BB) are reported for eight-membered ring in 1,3-dioxocin.37 The presence of planar residues could decrease the number of possible conformations which may be realized in these structures compared with saturated systems,38 the most populated form was found to be depended on the nature of planar residue and on the type of bridging group.39 In the structure of 1, the eight-membered cyclic nucleus, has 1,4-diplanar residue with CH2 group as a bridge. The dinaphtho dioxocin can act as a rigid cleft with two appended arms generating a synthetic receptor.
RESULTS AND DISSCUTION
Design, Molecular Modeling and Conformational Analysis
Continuing our studies on the dinaphtho[1,3]dioxocins,40 8-methyl-16H-dinaphtho[2,1-d:1,2-g][1,3]-dioxocin-2,14-diol was selected as a spacer to make 2,14-bis(benzyloxy)-8-methyl-16H-dinaphtho- [2,1-d:1,2-g][1,3]dioxocin 1 as an artificial cleft.
The design of 1 was done taking into account a number of structural features commonly used in the design of host molecules i.e. 1) the presence of a spacer that prevents self-association 2) a spacer that establishes a suitable distance between the pincers (plane to plane or centroid to centroid) and 3) capability of strong noncovalent interactions which stabilized the assembly of complex molecular architecture.41
8-Methyl-16H-dinaphtho[2,1-d:1,2-g][1,3]dioxocin 2 (Scheme 1) was selected as a model compound for conformational analysis of the dioxocin ring as the nucleus of the spacer in cleft 1. The solution and/or the crystal structures of a number of dioxocins are known.42
6H,12H-Dinaphtho[d,g][1,3]dioxocin 3 (Scheme 1) could adopt a boat-chair (BC) conformation of Cs symmetry and a distorted boat (DB) conformation of C1 symmetry.43 In most derivatives of 3, which were studied previously, the DB conformation has been found in the solid state.44 In some cases the BC conformation is reported as the sole form in the solid state as well as in solution.45 Geometry optimization at B3LYP/6-31G46 level of theory using Gaussian 9847 on 2 predict that DB conformation with exo methyl substitution (DB-exo) is the most stable form compared with BC with equatorial methyl (BC-e), DB form with endo methyl substitution (DB-endo) and BC with axial methyl (BC-a) (Figure 1). These conformations are true minima based on vibrational frequency calculations. The results of calculations on stable conformations performed with B3LYP/6-31G method are summarized in Table 1.
Calculated internal and torsion angles of DB-exo, DB-endo, BC-a and BC-e forms are given in Table 2. Although the axial and equatorial BC conformations have similar torsion angles, these angles are up to 30 degrees different in exo and endo DB forms.
The transition state for conversion of BC and DB forms is a half-chair(HC) conformation and the transition state between DB-endo and DB-exo is a boat-boat(BB) conformation as reported previously.48-50 The activation energies for these interconversions were calculated with semiempirical AM151 method and the conformational interconversion diagram is shown in Figure 2.
The required energy for interconversion of DB-exo to BC-e is 16.8 kcal/mol and for DB-endo to BC-a is 17.4 kcal/mol. Calculations reveal that these barriers will raise about 4 kcal/mol if oxygens in 2 are replaced by carbons. The calculated barrier for interconversion of DB-exo/DB-endo is 10.7 kcal/mol. These results show that the eight membered ring in 2 could adopt the DB or BC conformations. The eight membered ring in 1 resemble very closely the eight membered ring in 2, however, The DB conformation in 1 seems to be the only populated one based on coupling constant analysis of the 1H-NMR spectra (see the NMR analysis section). In 1 a very rapid equilibrium between the DB-exo and DB-endo is expected with the exo form as the predominant form in the equilibrium.
The optimized structure of 1 at B3LYP/6-31G level of theory based on DB-exo in dioxocin ring is shown in Figure 3a. The optimized structure of 1 in BC-e form shows that there is no plane of symmetry and the naphthalene rings are partially moved up and down. This unsymmetrical structure is probably due to the disfavored interaction of the peri hydrogens 1 and 15 of the naphthalene rings (Figure 3b).
Calculations show that the resultant cleft 1 could be used to allow recognition and binding of special guest molecules. This cleft is suitable to bind guests with capability of hydrogen bond formation as well as π-π interaction. Pharmacologically important guests such as phenethylamine, amphetamine, methamphetamine and ecstacy are the proper candidate which could have host:guest interaction with 1 via hydrogen bonding and π-π interactions.
NMR Analysis
The 500 MHz 1H-NMR spectra of 1 was recorded in CDCl3 at 298K. This well resolved spectrum (Figure 4) clearly reflects the C1 symmetry of molecule. The AB quartet of 2.9-4 ppm with 2JHCH=17.05 Hz belongs to Ar-CH2-Ar protons of dioxocin ring. This big 2J in similar dioxocin rings or other eight-membered heterocycles contained phosphorus and silicon is attributed to DB conformation,52 2J in BC form is reported to be about 13 Hz.53 Although the calculated difference in relative energies of DB-exo and BC-e is 0.12 kcal/mol, however, the 2J coupling constant of 17.05 Hz suggest the DB-exo being the predominant form, at least in solution.
The benzylic methylene groups of the pendant arms give rise to two separate AB quartet systems at 4.6-4.8 and 5.1-5.2 ppm respectively, which are assigned based on NMR calculations performed by Gauge-Including Atomic Orbital (GIAO)54 method on cleft 1 with DB-exo form of dioxocin ring. To compare the calculated data with the experimental ones, the calculated absolute shieldings have been subtracted from the calculated absolute shielding of TMS protons.
The difference in chemical shifts of H6 (6.25 ppm) and H10 (6.92 ppm) (Table 3) is intresting as H6 is shielded considerably. GIAO calculations show a difference of 0.2 ppm and predict the difference in the right order.
Pulay et al55 in a discussion of the GIAO method noted that since the chemical shift range of hydrogen is the smallest of all atoms, it will be very sensitive to variation in the geometry, methodology and basis set. Also, since the hydrogens are located on the periphery of the molecule, their chemical shift will be more sensitive to intermolecular interactions i.e. solvent effects, which have so far not been included in the NMR calculations.
The experimental and theoretical results, with their differences are presented in Table 3. The calculated and experimental 1H-chemical shifts are correlated with r2=0.9094. Similar calculations on cleft 1 with DB-endo, BC-e and BC-a forms of dioxocin ring show correlations with r2=0.8672, 0.8574 and 0.7858 respectively, which are all less than the DB-exo correlation coefficient.
Synthesis
This cleft was synthesized by the use of molecular "Lego" set consisting of naphthalene diol, benzyl chloride, formaldehyde and vinyl acetate.
The key precursor in the synthesis of host 1 was the [1,3]dioxocin ring, which is prepared according to the route depicted in the Scheme 2. In brief, 2,7-naphthalenediol 4 was allowed to react with 1.2 equivalent benzyl chloride in DMF using potassium carbonate as base following a literature procedure56 to produce 5. The subsequent coupling of 5 with formaldehyde in the presence of sodium acetate in refluxing MeOH afforded compound 6 in an overall yield of 63%. Compound 6 upon reaction with vinyl acetate and bis(acetonitrile)dichloro palladium as catalyst in dry THF at 60 °C for 12 h underwent cyclization to yield molecular cleft 1 in 25% yield.
CONCLUSION
In summary, molecular cleft 1 based on dioxocin ring was synthesized in three steps and its conformation is characterized by NMR spectroscopy. The conformation of dioxocin ring was assigned DB based on the geminal 2JHH coupling constant. Conformational analysis of model compound 2 reveals that the most populated forms is DB-exo, with BC-e, DB-endo and BC-a being 0.12, 0.754 and 2.66 kcal/mol higher in energy, respectively. This compound is of interest in view of its potential to act as a host.
COMPUTATIONAL METHODS
The geometry optimization was performed at the B3LYP/6-31G level of theory using the Gaussian 98.47
The proton chemical shifts were calculated at the B3LYP/6-31G* level of theory by GIAO. AM1 Semiemprical calculations were carried out by Polak-Ribiere algorithm in the Hyperchem program.57
EXPERIMENTAL
Materials and Spectroscopy. All chemicals and solvents were obtained from Merck and were used as received without further purification. Analytical and preparative TLC was performed on precoated silica gel plates purchased from Merck. All reactions were carried out in oven-dried glassware. Column chromatography was run on silica gel(60 mesh). 1H-NMR and 13C-NMR spectra were recoded on a DRX Avance Bruker-500 MHz spectrometer. IR spectra were recorded on Bruker model Equinox 55 spectrophotometer.
7-(Benzyloxy)-2-naphthol 5. In a 25 mL round bottom flask was dissolved 2,7-naphthalenediol 2 (0.5g, 3.12 mmol) and K2CO3 (0.57 g, 4.1 mmol) in DMF (10 mL) and the mixture purged with N2. Benzyl chloride (0.6 mL, 5.1 mmol) was added under N2 atmosphere and the reaction was stirred at 60 °C for 24 h. The solution was cooled and filtered. The white precipitate was washed with acetone and dried under vacuum, MeOH (20 mL) was added and boiled and filtered to separate the dibenzylated derivative which is insoluble in MeOH. The filtrate was concentrated under vacume. The crude product was purified by column chromatography (silicagel 60 mesh, 40g, 40×2cm) using 95:5 hexane:EtOAc as eluent. Yield was 45%. 1H-NMR (CDCl3): 5.1 (s, 2H), 7.01-7.03 (d, J = 8.74Hz, 1H), 7.08-7.12 (m, 3H), 7.38-7.53 (m, 5H), 7.68-7.70 (m, 2H), 8.08 (s, 1H); 13C-NMR (CDCl3): 70.46, 106.52, 109.27, 115.73, 117.09, 124.96, 128.00, 128.45, 129.05, 129.78, 130.03, 136.34, 137.34, 154.38, 157.92; IR (KBr; cm-1): 3165 (m), 1738 (w), 1626 (m), 1552 (m), 1448 (m), 1377 (m), 1354 (m), 1282 (w), 1201 (s), 1158 (m), 1118 (m), 999 (m), 953 (w), 864 (m), 800 (w), 750 (m), 700 (m).
7-(Benzyloxy)-1-{[7-(benzyloxy)-2-hydroxy-1-naphthyl]methyl}-2-naphthol 6. Compound 3 (0.1 g, 0.4 mmol) was dissolved in MeOH (2 mL), formaldehyde as aqueous solution 35% (38 µL, 0.4 mmol) and sodium acetate trihydrate (0.052 g, 0.4 mmol) were added. The mixture was refluxed for 2 h, cooled and filtered. MeOH was removed under reduced pressure and the residue was purified by column chromatography (silica gel 60 mesh, 20 g, 20×1.5cm) using 95:5 hexane:EtOAc as eluent with yield of 70%. 1H-NMR (CDCl3): 4.75 (s, 2H), 4.78 (s, 4H), 6.40 (s, 2H), 6.95 (d, J = 8.69 Hz, 2H), 7.07 (d, J = 8.85 Hz, J = 2.28 Hz, 2H), 7.30-7.39 (m, 10H), 7.59 (d, J = 8.70 Hz, 2H), 7.67 (d, J = 2.15 Hz, 2H), 7.71 (d, J = 8.88 Hz, 2H); 13C-NMR (CDCl3): 70.41, 104.51, 115.95, 116.15, 117.02, 125.609, 127.97, 128.30, 128.56, 128.86, 129.00, 130.91, 135.33, 137.28, 152.38, 158.26; IR (KBr; cm-1): 3352 (m), 3119 (m), 3078 (w), 1923 (w), 1732 (w), 1611 (w), 1590 (s), 1490 (s), 1332 (s), 1290 (s), 1200 (s), 1169 (m), 1110 (s), 955 (m), 865 (m), 843 (m), 795 (s), 751 (s); Anal. Calcd for C35H28O4. (512): C, 82.02; H, 5.69.
Found: C, 80.37; H, 5.35.
2,14-Bis(benzyloxy)-8-methyl-16H-dinaphtho[2,1-d:1,2-g][1,3]dioxocin 1. To a stirred solution of vinyl acetate (0.5 mL, 5.2 mmol) and PdCl2(MeCN)2 (0.03 g, 0.1 mmol) in dry THF (2 mL) was added compound 6 (0.096 g, 0.2 mmol). This was stirred in an oil bath at 60 °C for 12 h. Volatiles were removed under reduced pressure and the crude product was purified by column chromatography (silica gel 60 mesh, 40 g, 40×1.5 cm) using 95:5 hexane:EtOAc as eluent. Yield was 45%. 1H-NMR: 1.09(d, J = 6.25 Hz, 3H), 2.98 (d, J = 17.05 Hz, 1H), 4.02 (d, J = 17.05 Hz, 1H), 4.21 (q. J = 6.26 Hz, 1H), 4.63 (d, J = 12.34 Hz, 1H), 4.85 (d, J = 12.34 Hz, 1H), 5.13 (d, J = 11.34 Hz, 1H), 5.18(d., J = 11.34 Hz, 1H), 6.25 (d, J = 9.73 Hz, 1H), 6.82 (d, J = 2.45 Hz, 1H), 6.91-6.94 (m, 3H), 7.04 (t, J = 7.51Hz, 1H), 7.13-7.13 (m, 3H), 7.21-7.23 (d, J = 8.72 Hz, J = 2.41 Hz, 1H), 7.31-7.33 (m, 2H), 7.38-7.39 (m, 1H), 7.43-7.46 (t, J = 7.65 Hz, 2H), 7.51-7.52 (d, J = 7.13 Hz, 2H), 7.57 (d, J = 9.75 Hz, 1H), 7.71 (d, J = 8.79 Hz, 1H), 7.82 (d, J = 8.89 Hz, 1H); 13C-NMR: 15.85, 33.76, 53.78, 69.79, 70.34, 114.29, 114.97, 115.22, 116.21, 116.65, 123.07, 125.09, 127.64, 128.03, 128.12, 128.42, 128.66, 128.96, 130.66, 131.59, 134.37, 136.30, 137.18, 144.44, 147.07, 153.13, 158.05, 160.55; IR (KBr; cm-1): 3413 (w), 3030 (w), 2932 (w), 1894 (w), 1681 (m), 1620 (m), 1595 (m), 1511 (m), 1456 (m), 1434 (m), 1391 (m), 1370 (m), 1267 (m), 1208 (s), 1061 (s), 1019 (s), 832 (s), 741 (s), 727 (s); Anal. Calcd for C37H30O4. (538): C, 82.53; H, 5.58. Found: C, 81.03; H, 5.49.
ACKNOWLEDGMENTS
This work is supported by grants from the research council of University of Tehran. We express our appreciation to Prof. Uday Maitra (Department of Organic Chemistry, Indian Institute of Science, Bangalore, India) for his helpful discussion. We are also grateful to Prof. S. W. Ng for making us available his software (G98w) and hardware (computational time) facilities.
References
1. J.-M. Lehn, ‘Supramolecular Chemistry, Concepts and Perspectives’, VCH: Weinheim, 1995.
2. J. L. Atwood, J. E. D. Davies, D. D. MacNicol, and F. Vögtle, ‘Comprehensive Supramolecular Chemistry; Eds.’, Pergamon: Oxford, U.K., 1996.
3. M. C. T. Fyfe and J. F. Stoddart, Acc. Chem. Res., 1997, 30, 393. CrossRef
4. L. J. D' Souza and U. Maitra, J. Org. Chem., 1996, 61, 9494. CrossRef
5. a) E. Fischer, Ber. Dtsch. Chem. Ges., 1894, 27, 2985; CrossRef b) D. Yang, M. K. Rosen, and S. L. Schreiber, J. Am. Chem. Soc., 1993, 115, 819; CrossRef c) M. K. Rosen, D. Yang, P. K. Martin, and S. L. Schreiber, J. Am. Chem. Soc., 1993, 115, 821. CrossRef
6. C. W. Chen and H. W. Whitlock, Jr., J. Am. Chem. Soc., 1978, 100, 4921. CrossRef
7. S. K. Burley and G. A. Petsko, Adv. Protein Chem., 1988, 39, 125; CrossRef S. K. Burley and G. A. Petsko, Science, 1985, 229, 23. CrossRef
8. M. Harmata and T. J. Murray, J. Org. Chem., 1989, 54, 3761. CrossRef
9. M. Harmata and C. L. Barnes, Tetrahedron Lett., 1990, 31, 1825. CrossRef
10. M. Harmata and C. L. Barnes, J. Am. Chem. Soc., 1990, 112, 5655. CrossRef
11. M. Harmata, C. L. Barnes, S. R. Karra, and S. Elahmad, J. Am. Chem. Soc., 1994, 116, 8392. CrossRef
12. M. Harmata and S. Tyagarajan, Mol. Recognit. Inclusion Proc. Int. Symp. 9th, 1998, 353.
13. M. Harmata, M. Kahraman, S. Tyagarajan, C. L. Barnes, and C. J. Welch, Mol. Recognit. Inclusion Proc. Int. Symp. 9th, 1998, 109.
14. J. Fleischhauer, M. Harmata, M. Kahraman, A. Koslowski, and C. J. Welch, Tetrahedron Lett., 1997, 38, 8655. CrossRef
15. a) U. Maitra and L. J. D’Souza, J. Chem. Soc., Chem. Commun., 1994, 24, 2793; CrossRef b) N. Vijayalakshmi and U. Maitra, Org. Lett., 2005, 7, 2727. CrossRef
16. U. Maitra, L. J. D’Souza, and P. V. Kumar, Supramol. Chem., 1998, 10, 97. CrossRef
17. T. Tjivikua, A. Muehldorf, G. Deslongchamps, M. Famulok, and J. Rebek, Jr., J. Am. Chem. Soc., 1991, 113, 201. CrossRef
18. J. F. Blake and W. L. Jorgensen, J. Am. Chem. Soc., 1990, 112, 7269. CrossRef
19. S. C. Zimmerman, C. M. VanZyl, and G. S. Hamilton, J. Am. Chem. Soc., 1989, 111, 1373. CrossRef
20. T. Korenaga, T. Kosaki, Y. Kawauchi, T. Ema, and T. Sakai, J. Fluorine Chem., 2006, 127, 604. CrossRef
21. S. C. Zimmerman, W. Wu, and Z. Zeng, J. Am. Chem. Soc., 1991, 113, 196. CrossRef
22. S. C. Zimmerman, Top. Curr. Chem., 1993, 165, 71. CrossRef
23. S. C. Zimmerman, Bioorg. Chem. Front., 1991, 2, 33.
24. S. C. Zimmerman, M. Mrksich, and M. Baloga, J. Am. Chem. Soc., 1989, 111, 8528. CrossRef
25. S. C. Zimmerman and W. Wu, J. Am. Chem. Soc., 1989, 111, 8054. CrossRef
26. S. C. Zimmerman and C. M. VanZyl, J. Am. Chem. Soc., 1987, 109, 7894. CrossRef
27. V. K. Potluri and U. Maitra, J. Org. Chem., 2000, 65, 7764. CrossRef
28. F. G. Klarner and B. Kahlert, Acc. Chem. Res., 2003, 36, 919. CrossRef
29. M. Perry, P. J. Stansfeld, J. Leaney, C. Wood, M. Groot, D. Leishman, M. J. Sutcliffe, and J. S. Mitcheson, Mol. Pharmacol. 2006, 69, 509. CrossRef
30. a) M. M. Conn, G. Deslongchamps, J. de Mendoza, and J. Rebek, Jr., J. Am. Chem. Soc., 1993, 115, 3548; CrossRef b) G. A. Jeffrey and W. Saenger, ‘Hydrogen Bonding in Biological Structures’, Springer, Berlin, 1994.
31. T. H. Webb and C. S. Wilcox, Chem. Soc. Rev., 1993, 22, 282. CrossRef
32. a) S. S. Yoon and W. C. Still, J. Am. Chem. Soc., 1993, 115, 823; CrossRef b) L. F. Newcomb, T. S. Haque, and S. H. Gellmann, J. Am. Chem. Soc., 1995, 117, 6509. CrossRef
33. L. P. Burke, A. D. DeBellis, H. Fuhrer, H. Meier, S. D. Pastor, G. Rihs, G. Rist, R. K. Rodebaugh, and S. P. Shum, J. Am. Chem. Soc., 1997, 119, 8313. CrossRef
34. T. S. Cameron, J. Chem. Soc., Perkin Trans. 2, 1972, 5, 591. CrossRef
35. A. D. Hardy and F. R. Ahmed, Acta Cryst. Sect. B, 1974, 30, 1674. CrossRef
36. I. V. Anonimova, K. A. Ilyasov, T. E. Yabukov, E. G. Yarkova, N. R. Safiullina, V. V. Klochkov, and B. A. Arbuzov, Zh. Obshch. Khim., 1991, 6, 173.
37. R. P. Arshinova, A. Kh. Plyamovatyi, R. A. Kadyrov, S. G. Gnevashev, and B. A. Arbuzov, Phosphorus, Sulfur Silicon Relat. Elem., 1989, 41, 449. CrossRef
38. R. N. Renaud, J. W. Bowenkamp, R. Fraser, and J. L. Roustan, Can. J. Chem., 1977, 55, 3456. CrossRef
39. P. Hug, S. Kolly, H. Meir, R. Pitteloud, D. Poppinger, G. Rihs, and G. Rist, Helv. Chim. Acta, 1990, 73, 618. CrossRef
40. P. Rashidi-Ranjbar, A. Khoramabadi-Zad, and M. Roohi, Phosphorus, Sulfur Silicon Relat. Elem., 2000, 159, 229. CrossRef
41. B. J. Whitlock and H. W. Whitlock, Jr., J. Am. Chem. Soc., 1994, 116, 2301 and references cited therein. CrossRef
42. I. V. Anonimova, E. G. Yarkova, R. A. Shaikhutdinov, V. V. Klochkov, and B. A. Arbozov, Zh. Obshch. Khim., 1993, 63, 2405.
43. R. S. Khadiullin, R. P. Arshinova, I. V. Anonimova, A. K. Plyamovatyi, and R. R. Shagidullin, J. Mol. Struct., 1991, 245, 165. CrossRef
44. O. N. Kataeva, I. A. Litvinov, V. A. Naumov, and I. V. Anonimova, Bull. Acad. Sci. USSR Div. Chem. Sci., 1989, 1159. CrossRef
45. O. N. Kataeva, I. A. Litvinov, V. A. Naumov, and I. V. Anonimova, J. Mol. Struct., 1995, 344, 95. CrossRef
46. C. Lee, W. Yang, and R. G. Parr, Phys. Rev. B, 1998, 37, 785; CrossRef B. Miehlich, A. Savin, H. Stoll, and H. Preuss, Chem. Phys. Lett. 1989, 157, 200; CrossRef A. D. Becke, Phys. Pev. A, 1988, 38, 3098. CrossRef
47. M. J. Frisch, G. W. Trucks, H. B. Schlegel, G. E. Scuseria, M. A. Robb, J. R. Cheeseman, V. G. Zakrzewski, J. A. Montgomery, Jr., R. E. Stratmann, J. C. Burant, S. Dapprich, J. M. Millam, A. D. Daniels, K. N. Kudin, M. C. Strain, O. Farkas, J. Tomasi, V. Barone, M. Cossi, R. Cammi, B. Mennucci, C. Pomelli, C. Adamo, S. Clifford, J. Ochterski, G. A. Petersson, P. Y. Ayala, Q. Cui, K. Morokuma, D. K. Malick, A. D. Rabuck, K. Raghavachari, J. B. Foresman, J. Cioslowski, J. V. Ortiz, A. G. Baboul, B. B. Stefanov, G. Liu, A. Liashenko, P. Piskorz, I. Komaromi, R. Gomperts, R. L. Martin, D. J. Fox, T. Keith, M. A. Al-Laham, C. Y. Peng, A.Nanayakkara, C. Gonzalez, M. Challacombe, P. M. W. Gill, B. Johnson, W. Chen, M. W. Wong, J. L. Andres, C. Gonzalez, M. Head-Gordon, E. S. Replogle, and J. A. Pople, Gaussian, Inc., Pittsburgh PA, 1998.
48. J. Wohnert, J. Brenn, M. Stoldt, O. Aleksiuk, F. Grynszpan, I. Thondorf, and S. E. Biali, J. Org. Chem., 1998, 63, 3866. CrossRef
49. F. A. L. Anet and I. Yavari, J. Am. Chem. Soc., 1977, 99, 6986. CrossRef
50. F. E. Elhadi, W. D. Ollis, and J. F. Stoddart, Angew. Chem., Int. Ed. Engl., 1976, 15, 224. CrossRef
51. M. J. S. Dewar and W. Thiel, J. Am. Chem. Soc., 1977, 99, 4499; CrossRef L. P. Davis, J. Comp. Chem. 1981, 2, 433; CrossRef M. J. S. Dewar, M. L. McKee, and H. S. Rzepa, J. Am. Chem. Soc., 1978, 100, 3607; CrossRef M. J. S. Dewar, E. G. Zoebisch, and E. F. Healy, J. Am. Chem. Soc., 1985, 107, 3902. CrossRef
52. R. S. Glass, ‘Conformational Analysis of Medium-Sized Heterocycles’, VCH: New York, 1988.
53. S. D. Pastor and J. D. Spivak, J. Org. Chem., 1984, 49, 1297. CrossRef
54. K. Wolinski, J. F. Hilton, and P. Pauly, J. Am. Chem. Soc., 1990, 112, 8251; CrossRef J. L. Dodds, R. McWeeny, and A. J. Sadlej, Mol. Phys., 1980, 41, 1419; CrossRef R. Ditchfield, Mol. Phys. 1974, 27, 789; CrossRef R. McWeeny, Phys. Rev., 1962, 126, 1028; CrossRef F. London, J. Phys. Radium, Paris, 1937, 8, 397. CrossRef
55. P. Pulay and J. F. Hinton, ‘Encyclopedia of Nuclear Magnetic Resonance’, ed. by D. M. Grant and R. K. Haris, Wiley: New York, 1995, pp. 4334-4345.
56. A. K. Bandyopadhyaya, N. M. Sangeetha, and U. Maitra, J. Org. Chem., 2000, 65, 8239. CrossRef
57. Hyperchem, Release 7.0; Hypercube, Inc, 2002.