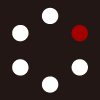
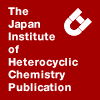
HETEROCYCLES
An International Journal for Reviews and Communications in Heterocyclic ChemistryWeb Edition ISSN: 1881-0942
Published online by The Japan Institute of Heterocyclic Chemistry
e-Journal
Full Text HTML
Received, 2nd July, 2009, Accepted, 28th July, 2009, Published online, 29th July, 2009.
DOI: 10.3987/COM-09-11788
■ Synthetically Useful Transformations of δ-Sultones and Thiane-1,1-dioxides Obtained by C-H Insertion
Christian S. Jungong, Jinu P. John, Joseph P. Bequette, and Alexei V. Novikov*
Chemistry Department, University of North Dakota, 151 Cornell St. Stop 9024, Grand Forks, ND 58201, U.S.A.
Abstract
δ-Sultones and thiane-1,1-dioxides that carry a carbethoxy group at α-position, easily accessible by C-H insertion, are potentially useful synthetic intermediates. Their stereoselective alkylation, rearrangement and conversion to γ-lactones have been demonstrated.INTRODUCTION
We recently reported a novel modification of C-H insertion that leads to selective formation of six-membered heterocycles (δ-sultones or thiane-1,1-dioxides, depending on the substrate used).1 Another report on formation of δ-sultones by this method has been published by Du Bois.2 Most notably, the usual overwhelming preference for formation of five-membered rings3,4 is overridden in this case (Scheme 1).
Later we also discovered that with a modification of structure and catalyst, certain thiolane-1,1-dioxides can be reached using this method.5 Only a few of δ-sultones and thiane-1,1-dioxides carrying a carbalkoxyl substituent at α-position have been reported, and even fewer of their chemical transformation have been performed. Meanwhile, these compounds show a lot of promise as synthetic intermediates. In the following report we describe our studies of the transformations of these compounds for purposes of use in synthesis.
RESULTS AND DISCUSSION
For δ-sultones, a particularly useful transformation would be a reductive scission of the C-S bond, as it would permit a “remote alkylation” at a γ-position relative to the alcohol functionality (Scheme 2).
While numerous examples of desulfonation have been performed with α-arylsulfonyl esters, no examples of this transformation are known for α-alkoxysulfonyl substituted esters. Presence of the aryl group on sulfur appears to be critical for the reaction, as common reagent for this reduction (Al/Hg,6 Na/Hg,7 Mg-MeOH,8 SmI2-MeOH9) did not induce any change in δ-sulfone 1. A report on α-desulfonation of fluoroalkylsulfonyl oxindoles quotes use of samarium(II) iodide at prolonged periods of time.10 Eventually, we have found that SmI2 in a combination with DMPU would effect this transformation (Table 1).
Use of HMPA produced similar results. The products isolated were valerolactones, which form, apparently, by the cyclization of the initially forming 5-hydroxyesters. The yields of lactones 1a and 2a are low apparently due to their volatility and a small scale of the reaction. The reaction appears to be sensitive to SmI2 source. Mixed success was achieved with commercial SmI2; we found the reaction most reliably working with SmI2 prepared by reaction of samarium metal with mercury iodide11 by modification of the reported procedure for preparation of SmCl3.12
Then, we moved on to study the potential uses of carbethoxy thiane-1,1-dioxides. These compounds are fairly strong C-H acids, permitting alkylation and similar reactions (although, notably, they are configurationally stable in absence of a base). We were particularly interested in the effect of the neighboring center (which is installed by C-H insertion).
The steric congestion in substrate 4, imposed by the quaternary center, did not impede the alkylation, which was possible with the use of active electrophiles under mild conditions (sodium hydride, alkylating agent, THF, rt, 16 h). Under these conditions it was also possible to alkylate sultone 1, without affecting the sulfonate, although Du Bois demonstrated that δ-sultones are susceptible to nucleophilic substitution.2 Also, gratifyingly, high diastereoselectivity (the other diastereomer was not detected) was observed in the alkylation of substrates 5 and 6 (Table 2).
The stereochemistry of alkylation products 5a and 5b was deduced from NOE correlations (Figure 1). The stereochemistry of 6a was deduced from NOEs of the alcohol 6b, obtained after DIBALH reduction.
Reduction of the ester group without affecting the sultone functionality in 1 can also be performed using DIBALH, although care needs to taken, as prolonged reaction times and higher temperatures degrade the yield. Borane-dimethyl sulfide also proved effective, although use of lithium aluminum hydride caused complication with both sulfones and sulfonates – low yields were obtained with 1, and apparently a complete hydrogenolysis of the ester functionality to the methyl group was happening with 5.
And finally, we have discovered that alkylated products can be rearranged by migration of carbethoxy group in a fashion similar to that of β-ketoesters,13 upon treatment with NaHMDS (Scheme 3).
This opens more possibilities for simple differential alkylation of thiane-1,1-dioxides, as well as construction of bicyclic sulfur-containing heterocycles (which could be converted to medium-sized carbocycles by desulfonation).
Thus, stereoselective alkylation of δ-sultones and thiane-1,1-dioxides, rearrangements of the alkylated products, and conversion of δ-sultones to valerolactones have been demonstrated. Further studies directed at the application of these compounds in synthesis are being performed and will be reported in due course.
EXPERIMENTAL
All reactions were carried out under an inert atmosphere of dry nitrogen in oven or flame-dried glassware. Proton magnetic resonance spectra were recorded at 500 MHz on an Avance 500 Bruker spectrometer. Carbon magnetic resonance spectra were recorded at 125 MHz on an Avance 500 Bruker spectrometer. All chemical shifts were reported in δ units relative to tetramethylsilane. High resolution mass spectral data were obtained on the Agilent 61969A TOF high resolution mass spectrometer using electrospray ionization, direct infusion, 10 mL/min in 50% MeOH 5mM ammonium formate. Melting points were determined on a MEL-TEMP melting point apparatus. Flash column chromatography was performed using 40-63 μm silica gel (Merck, Geduran, no. 11567-1) as the stationary phase. Tetrahydrofuran (THF) was dried by distilling from sodium–benzophenone in a continuous still under an atmosphere of nitrogen.
General procedure for reduction of δ-sultones with SmI2/DMPU.
To the solution of sultone (0.1 mmol) in dry THF (0.5 mL) containing DMPU (50 mg, 0.4 mmol) was added 0.1 M solution of SmI2 (commercially available, or prepared by dissolving solid SmI2, prepared by reaction of samarium metal with HgI2,11 in dry THF) until the blue color persisted, and 2 mL after that (typically around 5 mL). The reaction mixture was stirred at rt for the specified time, after which 1N HCl was added (5 mL). Hexane (30 mL) was added, and the reaction mixture was stirred for 15 min at rt. The layers were separated, the aqueous layer was washed with hexane (10 mL). The combined organic layers were washed with water (10 mL), dried over Na2SO4, and carefully concentrated. Flash chromatography (hexane-Et2O) provided the product.
The obtained lactones have been previously reported.14,15,16 The spectral data for the obtained compounds match those reported in the literature, as that of the independently prepared authentic samples.
General procedure for alkylation of δ-sultones and thiane-1,1-dioxides.
Sodium hydride (20 mg, 60% dispersion in mineral oil, 0.5 mmol) was washed with THF (3x1 mL), and a solution of thiane-1,1-dioxide (0.2 mmol) in THF (1 mL) was added to it. After stirring for 15 min (when gas evolution subsided), the corresponding alkyl halide (allyl bromide, ethyl iodide or p-chlorobenzyl chloride, 0.4 mmol) was added, and the reaction mixture was allowed to stir at rt for 16 h. 1N HCl (5 mL) was added, and the mixture was extracted with EtOAc (2x20 mL). The organic layer was dried with Na2SO4, and concentrated under reduced pressure. The product was purified by flash chromatography (hexane-EtOAc).
2-Allyl-2-carbethoxy-3,3-dimethylthiane-1,1-dioxide (4a): Pale yellow oil. 1H NMR (CDCl3, 500 MHz): δ 6.08-6.17 (m, 1H), 5.23 (dd, J = 17, 1.5 Hz, 1H), 5.14 (dd, J = 10, 1.5 Hz, 1H), 4.25-4.36 (m, 2H), 3.81 (td, J = 13.5, 4 Hz, 1H), 2.97-3.04 (m, 2H), 2.82 (dd, J = 15, 7 Hz, 1H), 2.12-2.29 (m, 2H), 1.92-1.99 (m, 1H), 1.33-1.39 (m, 4H), 1.36 (t, J = 7 Hz), 1.31 (s, 3H), 1.00 (s, 3H). 13C NMR (CDCl3, 125 MHz): δ 168.3 (C), 134.2 (CH), 118.6 (CH2), 78.0 (C), 62.2 (CH2), 51.2 (CH2), 40.1 (C), 36.4 (CH2), 31.8 (CH2), 28.8 (CH3), 23.0 (CH3), 19.4 (CH2), 14.2 (CH3). HRMS (ESI) calcd for C13H26NO4S (M+NH4)+ 292.1577, found 292.1559.
2-Ethyl-2-carbethoxy-3,3-dimethylthiane-1,1-dioxide (4b): Pale yellow oil. 1H NMR (CDCl3, 500 MHz): δ 4.33-4.39 (m, 1H), 4.24-4.32 (m, 1H), 3.84 (td, J = 13.5, 4 Hz, 1H), 3.00 (dt, J = 13.5, 3.5 Hz, 1H), 2.24-3.31 (m, 1H), 2.20 (ddd, J = 14, 3.5, 3 Hz, 1H), 2.03-2.11 (m, 2H), 1.90-1.96 (m, 1H), 1.36 (t, J = 7.3 Hz, 3H), 1.29-1.35 (m, 1H), 1.27 (s, 3H), 1.26 (t, J = 7.3 Hz, 3H), 0.96 (s, 3H). 13C NMR (CDCl3, 125 MHz): δ 168.4 (C), 78.7 (C), 62.0 (CH2), 52.0 (CH2), 40.2 (C), 36.3 (CH2), 28.7 (CH3), 23.3 (CH3), 20.9 (CH2), 19.4 (CH2), 14.3 (CH3), 11.1 (CH3). HRMS (ESI) calcd for C12H26NO4S (M+NH4)+ 280.1577, found 280.1577.
3-Allyl-3-carbethoxy-4,4-dimethyl-1,2-oxathiane-2,2-dioxide (1b): Pale yellow oil. 1H NMR (CDCl3, 500 MHz): δ 6.05 (ddt, J = 14, 10, 7 Hz, 1H), 5.27 (dd, J = 17, 1.5 Hz, 1H), 5.18 (dd, J = 10, 1.5 Hz, 1H), 4.72 (td, J = 12, 2 Hz, 1H), 4.44 (ddd, J = 11.5, 4.5, 2.5 Hz, 1H), 4.29-4.38 (m, 2H), 2.86-2.96 (m, 2H), 2.61 (td, J = 13.5, 4 Hz, 1H), 1.43 (s, 3H), 1.33-1.39 (m, 4H), 1.36 (t, J = 7 Hz), 0.99 (s, 3H). 13C NMR (CDCl3, 125 MHz): δ 166.5 (C), 133.4 (CH), 119.5 (CH2), 76.8 (C), 69.3 (CH2), 62.6 (CH2), 39.6 (CH2), 36.7 (CH2), 34.3 (CH2), 27.8 (CH3), 23.6 (CH3), 14.2 (CH3). HRMS (ESI) calcd for C12H24NO5S (M+NH4)+ 294.1369, found 294.1388.
(2r,3s)-2-Allyl-2-carbethoxy-3-methylthiane-1,1-dioxide (5a): Pale yellow oil. 1H NMR (CDCl3, 500 MHz): δ 6.10-6.19 (m, 1H), 5.20 (dq, J = 17, 1 Hz, 1H), 5.14 (d, J = 10 Hz, 1H), 4.26-4.37 (m, 2H), 3.79 (td, J = 13.5, 4.5 Hz, 1H), 3.11 (dd, J = 15, 5.5 Hz, 1H), 2.98 (dt, J = 13.5, 3.5 Hz, 1H), 2.82 (dd, J = 15.5, 8.5 Hz, 1H), 2.24-2.31 (m, 1H), 2.03-2.17 (m, 2H), 1.80 (qd, J=13, 3.5 Hz, 1H), 1.60 (dq, J = 14.5, 3.5 Hz, 1H, overlapped with water peak), 1.36 (t, J = 7.5 Hz, 3H), 0.99 (d, J = 6.5 Hz, 3H). 13C NMR (CDCl3, 125 MHz): δ 167.5 (C), 133.3 (CH), 119.0 (CH2), 75.5 (C), 62.4 (CH2), 50.7 (CH2), 39.1 (CH), 34.8 (CH2), 29.1 (CH2), 23.0 (CH2), 17.4 (CH3), 14.3 (CH3). HRMS (ESI) calcd for C12H21O4S (M+H)+ 261.1155, found 261.1171.
(2r,3s)-2-Carbethoxy-2-(4-chlorobenzyl)-3-methylthiane-1,1-dioxide (5b): Pale yellow oil. 1H NMR (CDCl3, 500 MHz): δ 7.42 (d, J = 8 Hz, 2H), 7.22 (d, J = 8 Hz, 2H), 4.30-4.40 (m, 2H), 3.76 (td, J = 13.5, 4 Hz, 1H), 3.66 (d, J = 14.5 Hz, 1H), 3.34 (d, J = 14.5 Hz, 1H), 2.96 (dt, J = 14, 3.5 Hz, 1H), 2.33-2.41 (m, 1H), 2.08-2.19 (m, 1H), 2.00-2.07 (m, 1H), 1.63-1.72 (m, 2H), 1.37 (t, J = 7 Hz, 3H), 1.07 (d, J = 7 Hz, 3H). 13C NMR (CDCl3, 125 MHz): δ 167.1 (C), 134.1 (C), 133.2 (CH), 133.1 (C), 128.0 (CH), 77.2 (C), 62.6 (CH2), 51.5 (CH2), 40.4 (CH), 35.6 (CH2), 29.6 (CH2), 22.9 (CH2), 18.1 (CH3), 14.4 (CH3). HRMS (ESI) calcd for C16H25NO4SCl (M+NH4)+ 362.1187, found 362.1172.
(2r,3s)-2-Carbethoxy-2-(4-chlorobenzyl)-3-methylthiolane-1,1-dioxide (6a): Pale yellow oil. 1H NMR (CDCl3, 500 MHz): δ 7.39 (d, J = 8.5 Hz, 2H), 7.25 (d, J = 8.5 Hz, 2H), 4.30 (qd, J = 7, 1.5 Hz, 2H), 3.44-3.52 (m, 2H), 3.27 (d, J = 14.5 Hz, 1H), 3.14-3.21 (m, 1H), 2.47-2.55 (m, 1H), 2.21-2.29 (m, 1H), 1.96-2.05 (m, 1H), 1.33 (t, J = 7.3 Hz, 3H), 0.90 (d, J = 7 Hz, 3H). 13C NMR (CDCl3, 125 MHz): δ 166.6 (C), 133.6 (C), 133.3 (C), 132.6 (CH), 128.5 (CH), 74.5 (C), 62.5 (CH2), 50.7 (CH2), 40.1 (CH), 35.2 (CH2), 27.0 (CH2), 17.0 (CH3), 14.3 (CH3). HRMS (ESI) calcd for C15H23NO4SCl (M+NH4)+ 348.1031, found 348.1035.
DIBALH reduction of 1 and 6a.
To the solution of 1 or 6a (0.1 mmol) in CH2Cl2 (0.5 mL), solution of DIBALH (0.5 mL, 1M in hexane, 0.5 mmol) was added at 0 oC. The reaction mixture was kept at 0 oC for 1h (1), or 10 h at rt (6a). Concentrated aqueous solution of sodium potassium tartrate was added (10 mL), and the reaction mixture was stirred for 1h. The layers were separated, the aqueous layer was washed with EtOAc (20 mL). The combined organic layers were washed with brine, dried over Na2SO4, and concentrated. Flash chromatography (hexane-EtOAc) provided the product.
3-Hydroxymethyl-4,4-dimethyl-1,2-oxathiane-2,2-dioxide (1c): Obtained in 90% yield. Clear oil, solidified on standing. m.p. 62-63 oC. 1H NMR (CDCl3, 500 MHz): δ 4.66 (td, J = 11.5, 2.5 Hz, 1H), 4.46 (dt, J = 11.5, 4 Hz, 1H), 4.20 (ddd, J = 12.5, 8, 3 Hz, 1H), 4.02-4.09 (ddd, J = 12.5, 8, 3 Hz, 1H), 3.11 (dd, J = 8, 3 Hz, 1H), 2.41 (dd, J = 8, 3 Hz, 1H), 1.92 (ddd, J = 14.5, 11.5 , 4 Hz, 1H), 1.61 (ddd, J = 14.5, 4, 2.5 Hz, 1H), 1.27 (s, 3H), 1.22 (s, 3H). 13C NMR (CDCl3, 125 MHz): δ 69.9 (CH), 69.7 (CH2), 58.3 (CH2), 38.9 (CH2), 35.8 (C), 29.9 (CH3), 22.1 (CH3). HRMS (ESI) calcd for C7H18NO4S (M+NH4)+ 212.0951, found 212.0950.
(2r,3s)-4-Chlorobenzyl-2-hydroxymethyl-3-methylthiolane-1,1-dioxide (6b): Obtained in 94% yield. Pale yellow oil. 1H NMR (CDCl3, 500 MHz): δ 7.28-7.31 (m, 4H), 3.94 (dd, J = 13, 6 Hz, 1H), 3.75 (dd, J = 13, 8 Hz, 1H), 3.42 (d, J = 14 Hz, 1H), 3.29 (ddd, J = 13, 8.5, 3 Hz, 1H), 3.07 (ddd, J = 13, 10.5, 8.5 Hz, 1H), 2.82 (d, J = 14 Hz, 1H), 2.59 (dd, J = 8, 6 Hz, 1H), 2.31-2.39 (m, 1H), 2.13-2.21 (m, 1H), 1.90-2.00 (m, 1H), 0.85 (d, J = 7 Hz, 3H). 13C NMR (CDCl3, 125 MHz): δ 133.8 (C), 133.5 (C), 132.2 (CH), 128.9 (CH), 68.1 (C), 61.3 (CH2), 51.4 (CH2), 39.5 (CH), 36.5 (CH2), 27.7 (CH2), 15.8 (CH3). HRMS (ESI) calcd for C13H21NO3SCl (M+NH4)+ 306.0925, found 306.0941.
Rearrangement of 4a.
4a (25 mg, 0.091 mmol) was dissolved in dry THF (0.2 mL), and solution of NaHMDS in THF (2M in THF, 90 µL, 0.18 mmol) was added. After stirring at rt for 1.5 h, the reaction was quenched with 1N HCl (5 mL), and extracted with EtOAc (2x20 mL). The organic layer was washed with brine, dried and concentrated. The products were isolated using flash chromatography (EtOAc-hexane). The products isomerise during chromatography (verified by resubjecting pure 7a to chromatography). Typically, small amounts of pure 7a and 7b were isolated, along with a mixture of the two. 7b (the more polar isomer) was determined to be the cis-isomer by diaxial NOE correlation between H2 and H6. Combined yield 20 mg (84%).
trans-2-Allyl-6-carbethoxy-3,3-dimethylthiane-1,1-dioxide (7a): Pale yellow oil. 1H NMR (CDCl3, 500 MHz): δ 5.94-6.03 (m, 1H), 5.16 (dd, J = 17, 1.5 Hz, 1H), 5.07 (dd, J = 10, 1.5 Hz, 1H), 4.21-4.32 (m, 2H), 3.88 (dd, J = 5, 3 Hz, 1H), 3.43 (dd, J = 7, 4 Hz, 1H), 2.78-2.87 (m, 1H), 2.50 (ddd, J = 14, 5, 3 Hz, 1H), 2.38-2.45 (m, 1H), 2.13 (dq, J = 15, 3 Hz, 1H), 2.04 (td, J = 14, 3 Hz, 1H), 1.39 (dt, J = 14.5, 3.5 Hz, 1H), 1.32 (t, J = 7 Hz, 3H), 1.15 (s, 3H), 1.11 (s, 3H). 13C NMR (CDCl3, 125 MHz): δ 167.2 (C), 136.8 (CH), 116.8 (CH2), 66.8 (CH), 63.9 (CH), 62.4 (CH2), 37.4 (C), 34.8 (CH2), 31.0 (CH3), 26.3 (CH2), 23.4 (CH2), 19.9 (CH3), 14.2 (CH3). HRMS (ESI) calcd for C13H23O4S (M+H)+ 275.1311, found 275.1320.
cis-2-Allyl-6-carbethoxy-3,3-dimethylthiane-1,1-dioxide (7b): Pale yellow oil. 1H NMR (CDCl3, 500 MHz): δ 5.94-6.03 (m, 1H), 5.14 (dd, J = 17, 1.5 Hz, 1H), 5.07 (dd, J = 10, 1.5 Hz, 1H), 4.25-4.37 (m, 2H), 3.81 (dd, J = 13, 3.5 Hz, 1H), 2.83-2.91 (m, 1H), 2.82 (dd, J = 7, 3 Hz, 1H), 2.37-2.51 (m, 2H), 2.14 (dq, J = 15, 3 Hz, 1H), 1.68 (ddd, J = 14.5, 4.5, 3 Hz, 1H), 1.52 (td, J = 14, 3 Hz, 1H), 1.33 (t, J = 7 Hz, 3H), 1.16 (s, 3H), 1.09 (s, 3H). 13C NMR (CDCl3, 125 MHz): δ 164.2 (C), 136.7 (CH), 117.1 (CH2), 71.6 (CH), 66.1 (CH), 62.8 (CH2), 39.6 (CH2), 37.7 (C), 30.9 (CH3), 26.7 (CH2), 23.5 (CH2), 19.5 (CH3), 14.3 (CH3). HRMS (ESI) calcd for C13H23O4S (M+H)+ 275.1311, found 275.1319.
ACKNOWLEDGEMENTS
This work was supported by the American Chemical Society Petroleum Research Fund under grant No. PRF#46278-G1. We thank Alena Kubatova for HRMS analyses. The work on TOF MS was supported by the National Foundation under grant No. CHE-0216038. Special thanks to Divine Dugah for samarium iodide preparation and handling.
References
1. J. P. John and A. V. Novikov, Org. Lett., 2007, 9, 61. CrossRef
2. S. A. Wolckenhauer, A. S. Devlin, and J. Du Bois, Org. Lett., 2007, 9, 4363. CrossRef
3. D. F. Taber and R. E. Ruckle, Jr., J. Am. Chem. Soc., 1986, 108, 7686. CrossRef
4. M. P. Doyle, L. J. Westrum, W. N. E. Wolthuis, M. M. See, W. P. Boone, V. Bagheri, and M. M. Pearson, J. Am. Chem. Soc., 1993, 115, 958. CrossRef
5. C. S. Jungong, J. P. John, and A. V. Novikov, Tetrahedron Lett., 2009, 50, 1954. CrossRef
6. H. O. House and J. K. Larson, J. Org. Chem., 1968, 33, 61. CrossRef
7. B. M. Trost, H. C. Arndt, P. E. Strege, and T. R. Verhoeven, Tetrahedron Lett., 1976, 3477. CrossRef
8. (a) G. H. Lee, E. B. Choi, E. Lee, and C. S. Pak, Tetrahedron Lett., 1993, 34, 4541; CrossRef (b) A. C. Brown and L. A. Caprino, J. Org. Chem., 1985, 50, 1749. CrossRef
9. G. A. Molander and G. Hahn, J. Org. Chem., 1986, 51, 1135. CrossRef
10. K. M. James, N. Willetts, and D. J. Procter, Org. Lett., 2008, 10, 1203. CrossRef
11. E. E. Delbridge, D. T. Dugah, C. R. Nelson, B. W. Skelton, and A. H. White, Dalton Trans., 2007, 143. CrossRef
12. G. B. Deacon, G. N. Pain, and T. D. Tuong, Inorg. Synth., 1990, 28, 291. CrossRef
13. J. F. Wolfe, T. M. Harris, and C. R. Hauser, J. Org. Chem., 1964, 29, 3249. CrossRef
14. R. D. Little, G. W. Muller, M. G. Venegas, G. L. Carroll, A. Bukhari, L. Patton, and K. Stone, Tetrahedron, 1981, 37, 4371. CrossRef
15. C. G. Chavdarian and C. H. Heathcock, J. Org. Chem., 1975, 40, 2970. CrossRef
16. M. P. Doyle, A. V. Kalinin, and D. G. Ene, J. Am. Chem. Soc., 1996, 118, 8837. CrossRef