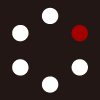
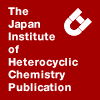
HETEROCYCLES
An International Journal for Reviews and Communications in Heterocyclic ChemistryWeb Edition ISSN: 1881-0942
Published online by The Japan Institute of Heterocyclic Chemistry
e-Journal
Full Text HTML
Received, 28th July, 2009, Accepted, 4th September, 2009, Published online, 8th September, 2009.
DOI: 10.3987/COM-09-S(S)83
■ Novel Carbohydrazide and Hydrazone Biomarkers Based on 9-Substituted Acridine and Anthracene Fluorogens
Zdenka Bedlovičová, Ján Imrich,* Pavol Kristian, Ivan Danihel, Stanislav Böhm, Danica Sabolová, Mária Kožurková, Helena Paulíková, and Karel D. Klika
Institute of Chemistry, Faculty of Science, P. J. Šafárik University, Moyzesova 11, SK-041 67 Košice, Slovak Republic
Abstract
Three series of carbohydrazides or hydrazones bearing either acridine or anthracene pharmacophores were synthesized as potential noncovalent DNA-binding antitumor agents. Carbohydrazides with an acridine or anthracene moiety were prepared from appropriate acridine or anthracene carbaldehydes via cyclocondensation with selected hydrazides whilst hydrazones with a 10H-acridin-9-ylidene moiety were obtained by condensation of (acridin-9-yl)hydrazine with various aldehydes or ketones. The spectroscopic properties of the first two series revealed efficient fluorescence implying that the compounds could be amenable for use as biomarkers. The structures of the compounds were characterized by spectral methods (UV-vis, fluorescence, IR, and 1H, 13C, and 2D NMR) and quantum-chemical calculations (DFT, ZINDO, and AM1). The first carbohydrazide series was also tested against human leukemia cell line HL-60 wherein the phenyl-substituted derivative was found to possess the highest activity.INTRODUCTION
The chemistry and biological properties of acridines1−3, especially their proclivity towards spiro−open-chain tautomerism1g−j as well as intercalating1a−g, fluorescent4, and antitumor activities5, have been the focal point of our research interest over two decades. Similarly too, because of their intercalating and fluorescent properties, we have paid attention to analogous anthracene substrates.6 Analogously, azomethines represent a class of compounds which are interesting not only from chemical1b and spectroscopic2 points of view, but also because they are the subject of biological and pharmaceutical research endeavors.7,8 Structural studies on the imine double bond of azomethines and ketimines have been conducted9 where the azomethines were found to occur as only one geometrical isomer whilst the ketimines were found to be present in the reaction mixture as two geometrical isomers. Recently, we have reported the physico-chemical properties of potentially intercalating 4-acridonecarboxamide imines.2
The aim of this contribution is to explore the effect of acridine vs. anthracene and the effect of substitution in the side-chain on the properties of analogous 9-substituted acridine/anthracene carbohydrazide and hydrazone compounds with respect to conjugation, prototropic tautomerism, intra- and interunit geometry, and spectral properties, in particular fluorescence, as well as their biological activities. The three series of novel carbohydrazides and hydrazones prepared here, together with a previous set,2 afforded a comparison between the various permutations regarding the positional placement of the tricyclic aromatic system, i.e. the acridine or anthracene moiety, with respect to the substituent unit.
RESULTS AND DISCUSSION
Synthesis
The synthesis of the first series of carbohydrazides 5a−e (Scheme 1) started from commercially available diphenylamine (1) giving, after modification of Bernthsen’s reaction,10 9-methylacridine (2). Following radical bromination of 2, 9-bromomethylacridine (3) was obtained11 and this provided (acridin-9-yl) carbaldehyde (4) after oxidation with sodium and 2-nitropropane.12 Subsequent condensation with various hydrazides (methyl, a; phenyl, b; 3-pyridyl, c; 4-pyridyl, d; and (9-oxoacridin-4-yl), e) then yielded substituted N'-(acridin-9-ylmethylene)hydrazides 5a−e in yields up to 74%. Anthracenyl derivatives of the second series were prepared by the condensation of commercially available 9-anthraldehyde (6) with the same set of hydrazides to provide substituted N'-(anthracen-9-ylmethylene)hydrazides 7a−e in excellent yields up to 90%. The third series of disubstituted hydrazones of 9,10-dihydroacridine, together with two trisubstituted ones, were prepared as indicated in Scheme 2 starting from the Ullmann−Jourdan condensation of o-chlorobenzoic acid (8) and aniline (9) to provide N-phenylanthranilic acid (10). Compound 10 upon treatment with POCl3 yielded
9-chloroacridine (11), which then readily yielded (acridin-9-yl)hydrazine (12) by the application of hydrazine monohydrate. Disubstituted 13a−e and trisubstituted 9-(alkyl/benzylidenehydrazono)-9,10- dihydroacridines 13f,g were then prepared by condensation of 12 with a set of aldehydes and ketones (benzaldehyde, a; 2,4,6-trimethoxybenzaldehyde, b; 4-bromobenzaldehyde, c; 4-methoxybenzaldehyde, d; 2,4,6-trimethylbenzaldehyde, e; acetone, f; acetophenone, g) under acidic catalysis in yields of 41−93% for the 13a−e and 38% and 42% for 13f,g, respectively.
NMR spectroscopy
In general, the N-10 nitrogen located in the structural framework of the acridine moiety has an extremely strong tendency to retain a proton,1b,f,g,i−n,3,13 i.e. if tautomeric interchanges are possible, then the H-10 tautomer usually dominates and the predominance of the equilibrium can easily be ascertained by standard methods of appraisal (correlations and diagnostic NMR chemical shifts compared to previous reports1b,f−n,3). Thus determination that the H-13 tautomer as depicted in Scheme 1 dominates over the H-10 tautomer for the compounds of the acridine series 5a−e provides a rare exception1h against this general rule, and despite the potential gain from the additional conjugation with the carbonyl double bond that would arise. The H-13 tautomeric structure permits free rotation about the C9−C11 bond and as a consequence the outer rings of the acridine moiety are rendered equivalent on the NMR timescale.
Interestingly, the only two compounds of the three series to displayed two sets of signals in solution for a major and minor species, the first one, 5a, in the integral intensity ratio 67 : 33 (Figure 1). Upon heating to 80° C the corresponding signals in the 1H NMR spectrum started to coalescence but resumed their normal appearance upon lowering the temperature back to 25° C. It appears that this equilibrium is due to E/Z configurational interchange about the amide bond, i.e. geometrical isomerism, based on the chemical shift differences which exist only in the side-chain for these two species. The other derivatives
5b−e in the series and compounds 7b−e in the second series are likely to be simply strongly biased in this respect and thus they exhibit only the one set of signals. The corresponding methyl-substituted derivative 7a in the anthracene series also showed two species in the ratio 69 : 31, the minor one characterized by some typical chemical shifts with other signals overlapped by the signals of the major species.
For the predominant tautomeric structures of 5a−e, there are two competing mechanisms for conjugation of the lone pair of the N-13 nitrogen atom (structure A, Figure 2). The NMR chemical shifts for C-9, C-11, and the carbonyl carbon C-14 as well as the chemical shifts of the protons in the side-chain are all strongly affected by these mechanisms, in particular if there is a dominant one in effect.
Conjugation of the considered lone pair with the carbonyl oxygen is affected by the substituents R attached to the carbonyl carbon whilst conjugation extending down into the tricyclic aromatic system is obviously determined to a large extent by whether an acridine or an anthracene moiety is present since replacement of the nitrogen atom N-10 in the acridinyl series by a carbon atom in the anthracenyl series will clearly mitigate any conjugation arising from the tricyclic aromatic system. Hence, comparison of the observed nuclear shifts in series 5a−e with the analogous series 7a−e should thus allude to any dominating mechanism. The pertinent chemical shifts presented in Table 1 are in accordance with the expected electronic character of the substituents. For example, the effect of the electron donating character of the methyl substituent in 5a on carbon C-11 is unequivocal (138.9 ppm) whilst the electron accepting character of the 4-pyridinyl-substituent of 5d (146.1 ppm) is also evident. Conversely, a reverse substituent effect is obvious for the carbonyl carbon C-14, cf. 5a, 172.4 ppm and 5d, 161.9 ppm, and this
same effect is also observed for the acridinyl carbon C-9. While the chemical shifts of carbons C-4a and C-10a are affected only very slightly by the substituents, those of carbons C-8a and C-9a are not affected at all. The effects of the substituents on the chemical shifts of the amidic hydrogen H-13 are likewise in concert with the electronic character of the substituents, e.g. cf. 5a, 11.79 ppm and 5d, 12.56 ppm.
Similar substituent effects are also manifested in the anthracene series 7a−e. For example, the chemical shift of C-14, cf. 7a, 171.9 ppm and 7d, 161.5 ppm, are comparable to the comparison of 5a. The evident slight difference between the two series is due to the electron accepting character of the N-10 nitrogen atom in acridine compared to the C-10 carbon atom in the anthracene unit.
Thus, from the chemical shift values, it can be concluded that there is an efficient transfer mechanism of electronic effects from the substituents throughout the molecules in these two series of compounds 5a−e and 7a−e but it appears there is considerable participation from both mechanisms with neither dominating.
In the case of derivatives 13a−g, the values of various diagnostic 13C NMR chemical shifts (e.g. C-4a and C-10a, 138−142; C-8a and C-9a, 114−120; and, in particular,1i,3 C-4 and C-5, 115−116 ppm) in comparison to previous reports1b,f−n,3 clearly indicate that the dihydroacridine structure of the acridine moiety is predominant, i.e. the H-10 tautomer dominates over the H-11 tautomer. For the H-10 tautomer, interchange between the conformers is slow and as a consequence the outer rings of the acridine moiety are rendered inequivalent on the NMR timescale.
The conjugation between the acridine unit, emanating from the nitrogen heteroatom, and the side-chain is mitigated by the substituents R1 and R2 with the phenyl substituents aiding conjugation whilst the methyl substituents in ketimines 13f,g reduce this conjugation by their inductive effects. These inductive effects manifest themselves on the chemical shift of the carbon of the azomethine group (C=N) with some deshielding evident for C-13 in 13g (phenyl and methyl substituents present) and with greater deshielding for C-13 in 13f (two methyl substituents present). Conversely, there is some shielding observed for C-9 in 13g in comparison to the very consistent values for aldimines 13a−e (149−150 ppm) with greater deshielding evident for the carbon atom C-9 in 13g. The aiding of conjugation by the phenyl substituents was also evident from the UV spectra (vide infra).
UV-vis absorption spectroscopy
Electronic absorption spectra of compounds 5a−d, 7a−e, and 13a−g measured in methanol are presented in Table 2. Compounds 5a−d exhibited broad absorption bands with their principal maxima lying in the range 363−367 nm together with a shoulder in the range 402−407 nm. Compounds 7a−d each displayed only a clear single band in the range 386−413 nm whilst compounds 13a−g each displayed a long wavelength maximum in the range 406−441 nm together with one or more two bands at ca. 320 and 280 nm. These absorbances are characteristic for n→π* transitions and the long wavelength maxima of compounds 13 are shifted to longer wavelengths compared to series 5a−d and 7a−e as a consequence of extended conjugation (cf. Figure 2).
In compounds 5a−d, the positions of the absorption maxima are only slightly influenced by the nature of the substituents (Figure 3) whereas by comparison the changes were more notable for 7a−e and 13a−g (Table 2). The largest bathochromic shift was observed for 13c due to the presence of the 4-bromophenyl substituent which not only extends the conjugation by way of the phenyl unit, but the large effect of which could be attributed also to the electron accepting character of the bromine atom. On the other hand, the shorter conjugation system due to the absence of the phenyl ring in 13f was confirmed by a distinct hypsochromic shift of the long wavelength maximum to 406 nm.
Conjugation and transitions in the UV-vis spectra for the phenyl-substituted compounds 5b, 7b, and 13a were interpreted by orbital analysis using the semiempirical ZINDO method on structures optimized by DFT at the B3LYP/6-311+G(d,p) level of theory. From the orbitals depicted in Figure 4 it follows that the
HOMO and LUMO represent net π and π* orbitals and these contribute strongly to the conjugation of the acridine moiety and the side-chain. Intriguingly, the phenyl substituent in 5b and 7b does not participate in the extended conjugation in contrast to 13a where the phenyl substituent does appear to participate.
In the case of 5b, the contribution to the absorbance band of the lone electron pair of N-10 in the acridine moiety is via the HOMO-4 and HOMO-5 orbitals. A similar contribution by the analogous lone pair in 13a was not found and it participates via the π (and π* accordingly) HOMO, HOMO-1, HOMO-2, and LUMO orbitals.
Fluorescence emission spectroscopy
Due to the planar aromatic units they contain, acridine and anthracene derivatives express unique chemical and physical properties with substitution at the C-9 position able to improve their luminescent properties.14 Fluorescence emission spectra taken in methanol of compounds 5a−d, 7a−d, and 13b,c were
recorded at excitation wavelengths corresponding to their absorbance maxima. It can be seen from Figure 5 that compounds 5a−d each had one emission maximum in the range 479−482 nm but that of the reference compound 9-isothiocyanatoacridine (acridine-ITC) exhibited three emission peaks at 479, 457, and 429 nm (Table 3). The highest fluorescence observed, that for derivative 5c, is almost 14-fold higher by comparison to acridine-ITC, a compound known as a fluorescence marker for amino and thiol groups present in biomacromolecules.15 The fluorescence emission spectra of compounds 4, 7a−d, 13b,c, and the reference compound acridine are summarized in Table 4 wherein it can be seen that prepared compounds
excepting 13b,c exhibited stronger fluorescence than the reference. For samples of varying concentration, the observation of a non-linear concentration dependence of fluorescence is an indication of a change in the oligomeric state of a fluorogen with the most common oligomeric forms of fluorogens, J-aggregates and excimers, evident by the sharpening of their absorbance spectra and broad red-shifted fluorescence, respectively.16 At high concentration, 3.8 x 10-3 M, a red-shifted fluorescence ca. 40–70 nm was observed for the acridine derivative 5d as well as for the analogous anthracene derivative 7d (Figure 6) but, notably, without any significant perturbations in their absorbance spectra. These changes in the fluorescence spectra are thus ascribed15,16 to the formation of excimer species for these two compounds (5d* and 7d*, respectively).
The observed reduced fluorescence in 13b,c and its underlying cause is of interest and the explanation may lie in the structural differences between the ground and the first excited states. For the DFT-optimized structures at the B3LYP/6-311+G(d,p) level of theory of the methyl-substituted derivatives 5a and 7a, amongst the probable structures, the most preferable were found to be the E,E-configurations (Figure 7a,b) where the HC=N−N−C=O segment is also planar. By contrast, whilst the acridine and anthracene moieties in 5a and 7a, respectively, are perfectly planar, the dihydroacridine moiety in the dimethyl-substituted derivative 13f is considerably deformed (Figure 7c) and no longer forms an ideal plane and instead it forms a shallow and a slightly twisted butterfly conformation folded about the C-9···N-10 axis. This has been confirmed by X-ray analysis in the case of spiroacridine and acridinium derivatives containing a dihydroacridine ring.17 The calculated first excited state (Figure 7d), however, is essentially planar and thus the supposition that the structural differences between the ground and the first excited states may limit the ability for fluorescence to occur appears to be valid.
Biological activities
To evaluate the biological activity potential of the compounds, preliminary investigations were conducted by testing compounds 5a−d for their cytotoxic effect against human leukemia cell line HL-60. From the concentrations that produced 25% inhibition (IC25) of cell viability (Table 5), it is evident that the tested
derivatives do clearly possess mild cytotoxic properties wherein the most significant activity was observed for the phenyl-substituted derivative 5b. A more than 100-fold increase of IC50 compared to IC25 in all studied compounds evidences undoubtely a good HL-60 cell reparation at higher concentrations of 5. This can be ascribed either to a hampered incorporation of 5 into tumor cells nuclei due to a binding into other cell organelles or an induction of a repair cell mechanisms. For example, enzymatic pathways enhancing the synthesis of low-molecular intracelular thiols as glutathione (GSH), which can interact by its thiol group with studied derivatives to protect DNA, were proven in a similar case.18 The protection extent may be high when glutathione is bound to strongly intercalating compound.
EXPERIMENTAL
General
Melting points were determined on a Boetius block and are uncorrected. Elemental analyses were performed using a Perkin−Elmer CHN 2400 analyzer. IR spectra were recorded on a Nicolet 6700 FT IR instrument.
NMR spectra were obtained at room temperature in hexadeuteriodimethyl sulfoxide (DMSO-d6) using a Varian Mercury Plus NMR spectrometer operating at 400.13 MHz for 1H and 100.61 MHz for 13C. Tetramethylsilane was used as an internal standard for both 1H and 13C nuclei (δTMS = 0.00 ppm for both). NMR chemical shift assignments were rendered by standard application of 1-D and 2-D correlation spectra wherein heteronuclear HSQC and HMBC experiments were optimized on 145 Hz for one-bond couplings and on 8 Hz for long-range couplings. Full descriptions of the NMR methodology have been described elsewhere.2,3,19,20
UV-vis spectra were measured on a Varian Cary 100 UV-vis spectrophotometer in 0.01 m Tris buffer (pH 7.4). The working concentration of all compounds was 4.8 × 10−6 m. All measurements were performed at 24 °C.
Fluorescence measurements were scanned on a Varian Cary Eclipse spectrofluorimeter. The excitation and emission slits were 10 nm for compounds 5a−d and 9-isothiocyanatoacridine and 20 nm for compounds 4, 7a−d, 13b,c and acridine. Emission spectra were recorded in the range 350−600 nm at concentrations of 6.0 × 10−6 and 3.8 × 10−3 m. The excitation wavelengths, λex, were: 4, 400; 5a−d, 384; 7a, 388; 7b, 434; 7c, 394; 7d, 397; 13b, 436; 13c, 440; acridine, 355; and 9-isothiocyanatoacridine, 400 nm. Fluorescence intensities are expressed in arbitrary units. All measurements were performed at 24 °C.
Semiempirical AM1 calculations21 were first performed to obtain the input geometries for DFT calculations at the B3LYP/6-311+G(d,p) level of theory22 using Gaussian03 software.23 UV spectra were analyzed using the semiempirical ZINDO method.24
(Acridin-9-yl)carbaldehyde (4)
Compound 4 was prepared according to literature methodology starting from commercially available diphenylamine 1 giving, after modification of Bernthsen’s reaction,10 9-methylacridine (2). Following radical bromination of 2, 9-bromomethylacridine (3) was obtained11 which then provided (acridin-9-yl) carbaldehyde (4) after oxidation using sodium and 2-nitropropane.12
General procedure for the preparation of carbohydrazides 5a−e
To a solution of the appropriate hydrazide (1.46 mmol) in anhydrous methanol, one drop of conc. aq. HCl and (acridin-9-yl)carbaldehyde (4, 1.47 mmol) was added. The reaction mixture was left to stir ca. 3½ h at rt until a precipitate had formed. The precipitate was filtered off, washed with methanol and then dried followed by recrystallization from methanol.
N'-(Acridin-9-ylmethylene)acetohydrazide (5a). Yield 59%; mp 244–247 ºC; IR (KBr) ν: 3211 (NH), 3076 (CHarom), 1668 (C=O), 1633 (C=N) cm−1. Major species: 1H NMR δ: 11.79 (s, 1H, NH), 9.25 (s, 1H, H-11), 8.67 (m, 2H, H-1,8), 8.25 (d, 2H, H-4,5, J = 8.4 Hz), 7.96 (m, 2H, H-3,6), 7.76 (m, 2H, H-2,7), 2.32 (s, 3H, CH3); 13C NMR δ: 172.4 (C-14), 146.7 (C-4a,10a), 138.9 (C-11), 136.8 (C-9), 131.4 (C-3,6), 128.0 (C-4,5), 127.3 (C-2,7), 125.3 (C-1,8), 123.3 (C-8a,9a), 20.4 (CH3). Minor species: 1H NMR δ: 12.16 (s, 1H, NH), 9.42 (s, 1H, H-11), 8.67 (m, 2H, H-1,8), 8.25 (d, 2H, H-4,5, J = 8.4 Hz), 7.96 (m, 2H, H-3,6), 7.76 (m, 2H, H-2,7), 2.13 (s, 3H, CH3); 13C NMR δ: 166.2 (C-14), 142.0 (C-11), 21.7 (CH3); Anal. Calcd For C16H13N3O (263.30): C, 72.99; H, 4.98; N, 15.96. Found: C, 72.61; H, 5.07; N, 15.75%.
N'-(Acridin-9-ylmethylene)benzohydrazide (5b). Yield 43%; mp 262–264 ºC; IR (KBr) ν: 3213 (NH), 3057 (CHarom), 1647 (C=O), 1601 (C=N) cm−1; 1H NMR δ: 12.35 (s, 1H, NH), 9.69 (s, 1H, H-11), 8.78 (d, 2H, H-1,8, J = 7.2 Hz), 8.23 (d, 2H, H-4,5, J = 8.4 Hz), 8.06 (m, 2H, H-2',6'), 7.91 (m, 2H, H-3,6), 7.75 (m, 2H, H-2,7), 7.68 (m, 1H, H-4'), 7.64 (m, 2H, H-3',5'); 13C NMR δ: 163.4 (C-14), 148.4 (C-4a,10a), 144.8 (C-11), 134.6 (C-9), 133.0 (C-1'), 132.2 (C-4'), 130.3 (C-3,6), 129.8 (C-4,5), 128.7 (C-3',5'), 127.7 (C-2',6'), 127.2 (C-2,7), 125.3 (C-1,8), 123.4 (C-8a,9a); Anal. Calcd For C21H15N3O (325.37): C, 77.52; H, 4.65; N, 12.91. Found: C, 77.31; H, 4.87; N, 12.65%.
N'-(Acridin-9-ylmethylene)nicotinohydrazide (5c). Yield 64%; mp 263–266 ºC; IR (KBr) ν: 3209 (NH), 3063 (CHarom), 1651 (C=O), 1620 (C=N) cm−1; 1H NMR δ: 12.96 (s, 1H, NH), 9.85 (s, 1H, H-11), 9.30 (s, 1H, H-2'), 8.87 (m, 1H, H-6'), 8.82 (d, 2H, H-1,8, J = 8.8 Hz), 8.52 (d, 1H, H-4', J = 6.4 Hz), 8.29 (d, 2H, H-4,5, J = 8.8 Hz), 8.02 (m, 2H, H-3,6), 7.81 (m, 2H, H-2,7), 7.70 (m, 1H, H-5'); 13C NMR δ: 161.8 (C-14), 152.3 (C-6'), 148.5 (C-2'), 145.6 (C-4a,10a), 144.9 (C-11), 137.9 (C-9), 136.2 (C-4'), 132.2 (C-3,6), 128.7 (C-3'), 127.6 (C-2,7), 126.9 (C-4,5), 125.7 (C-1,8), 123.9 (C-5'), 123.4 (C-8a,9a); Anal. Calcd For C20H14N4O (326.36): C, 73.61; H, 4.32; N, 17.17. Found: C, 74.01; H, 4.09; N, 16.75%.
N'-(Acridin-9-ylmethylene)isonicotinohydrazide (5d). Yield 74%; mp 249–252 ºC; IR (KBr) ν: 3257 (NH), 3072 (CHarom), 1678 (C=O), 1632 (C=N) cm−1; 1H NMR δ: 12.56 (s, 1H, NH), 9.68 (s, 1H, H-11), 8.87 (d, 2H, H-2',6', J = 4.8 Hz), 8.73 (d, 2H, H-1,8, J = 8.4 Hz), 8.22 (d, 2H, H-4,5, J = 8.8 Hz), 7.95 (d, 2H, H-3',5', J = 4.8 Hz), 7.91 (m, 2H, H-3,6), 7.74 (m, 2H, H-2,7); 13C NMR δ: 161.9 (C-14), 150.5 (C-2',6'), 148.3 (C-4a,10a), 146.1 (C-11), 140.1 (C-4'), 134.3 (C-9), 130.3 (C-3,6), 129.8 (C-4,5), 127.3 (C-2,7), 125.2 (C-1,8), 123.4 (C-8a,9a), 121.6 (C-3',5'); Anal. Calcd For C20H14N4O (326.36): C, 73.61; H, 4.32; N, 17.17. Found: C, 73.97; H, 4.02; N, 16.57%.
N'-(Acridin-9-ylmethylene)-9-oxo-9,10-dihydroacridine-4-carbohydrazide (5e). Yield 28%; mp 331−334 °C; IR (KBr) ν: 3275 (NH), 3066 (CHarom), 1647 (C=Oacridone), 1616 (C=Oamide), 1587 (C=N) cm−1; 1H NMR δ: 12.70 (s, 1H, NH), 11.92 (s, 1H, H-10'), 9.75 (s, 1H, H-11), 8.87 (d, 2H, H-1,8, J = 8.8 Hz), 8.57 (d, 1H, H-1', J = 7.6 Hz), 8.47 (d, 1H, H-3', J = 7.6 Hz), 8.27 (m, 3H, H-4,5,8'), 7.94 (m, 2H, H-3,6), 7.92 (m, 1H, H-5'), 7.79 (m, 3H, H-2,7,6'), 7.50 (m, 1H, H-2'), 7.35 (m, 1H, H-7'); 13C NMR δ: 176,4 (C-9'), 162.7 (C-14), 145.9 (C-11), 134.0 (C-6'), 133.6 (C-3'), 130.8 (C-1'), 130.3 (C-3,6), 129.8 (C-4,5), 127.3 (C-2,7), 125.7 (C-8'), 125.1 (C-1,8), 121.8 (C-7'), 119.9 (C-2'), 118.6 (C-5'); Anal. Calcd For C28H18N4O2 (442.48): C, 76.01; H, 4.10; N, 12.66. Found: C, 76.28; H, 4.27; N, 12.25%.
General procedure for the preparation of carbohydrazides 7a−e
To a solution of the appropriate hydrazide (1.46 mmol) in anhydrous methanol, one drop of conc. aq. HCl and 9-anthraldehyde (6, 1.47 mmol) was added. The reaction mixture was stirred ca. 3½ h at rt. The precipitate that had formed was filtered off, washed with methanol and dried followed by recrystallization from methanol.
N'-(Anthracen-9-ylmethylene)acetohydrazide (7a). Yield 85%; mp 231–235 °C; IR (KBr) ν: 3199 (NH), 3066 (CHarom), 1666 (C=O), 1624 (C=N) cm−1; Major species: 1H NMR δ: 11.47 (s, 1H, NH), 9.21 (s, 1H, H-11), 8.70 (s, 1H, H-10), 8.59 (d, 2H, H-1,8, J = 8.4 Hz), 8.15 (d, 2H, H-4,5, J = 8.4 Hz), 7.65 (m, 2H, H-2,7), 7.58 (m, 2H, H-3,6), 2.28 (s, 3H, CH3); 13C NMR δ: 171.9 (C-14), 141.4 (C-11), 130.8 (C-4a,10a), 129.4 (C-8a,9a), 129.1 (C-10), 128.9 (C-4,5), 127.0 (C-2,7), 125.4 (C-3,6), 125.1 (C-9), 124.5 (C-1,8), 20.4 (CH3); Minor species: 1H NMR δ: 11.69 (s, 1H, NH), 9.34 (s, 1H, H-11), 8.64 (s, 1H, H-10), 2.08 (s, 3H, CH3); 13C NMR δ: 165.6 (C-14), 144.5 (C-11), 21.7 (CH3). Anal. Calcd For C17H14N2O (262.31): C, 77.84; H, 5.38; N, 10.68. Found: C, 77.42; H, 5.07; N, 10.45%.
N'-(Anthracen-9-ylmethylene)benzohydrazide (7b). Yield 90%; mp 279–282 ºC; IR (KBr) ν: 3219 (NH), 3061 (CHarom), 1647 (C=O), 1622 (C=N) cm−1; 1H NMR δ: 12.14 (s, 1H, NH), 9.71 (s, 1H, H-11), 8.79 (d, 2H, H-1,8, J = 8.4 Hz), 8.74 (s, 1H, H-10), 8.17 (d, 2H, H-4,5, J = 8.0 Hz), 8.06 (d, 2H, H-2',6', J = 7.2 Hz), 7.69–7.58 (m, 7H, H-2,3,6,7,3'−5'); 13C NMR δ: 163.0 (C-14), 146.9 (C-11), 133.3 (C-1'), 131.8 (C-4'), 130.9 (C-4a,10a), 129.6 (C-10), 129.6 (C-8a,9a), 128.9 (C-4,5), 128.5 (C-2,7), 127.6 (C-2',6'), 127.1 (C-3',5'), 125.5 (C-3,6), 125.0 (C-9), 124.8 (C-1,8); Anal. Calcd For C22H16N2O (324.38): C, 81.46; H, 4.97; N, 8.64. Found: C, 81.08; H, 4.67; N, 9.02%.
N'-(Anthracen-9-ylmethylene)nicotinohydrazide (7c). Yield 83%; mp 265–268 °C; IR (KBr) ν: 3202 (NH), 3084 (CHarom), 1643 (C=O), 1624 (C=N) cm−1; 1H NMR δ: 12.34 (s, 1H, NH), 9.69 (s, 1H, H-11), 9.28 (s, 1H, H-2'), 8.88 (m, 1H, H-6'), 8.73 (m, 3H, H-1,8,10), 8.39 (m, 1H, H-4'), 8.13 (m, 2H, H-4,5), 7.65 (m, 1H, H-5'), 7.64 (m, 2H, H-2,7), 7.57 (m, 2H, H-3,6); 13C NMR δ: 161.7 (C-14), 152.2 (C-6'), 148.5 (C-2'), 147.7 (C-11), 135.5 (C-4'), 130.8 (C-4a,10a), 129.8 (C-10), 129.6 (C-8a,9a), 129.0 (C-4,5), 127.2 (C-2,7), 125.5 (C-3,6), 124.8 (C-1,8), 124.7 (C-3',5'), 124.5 (C-9); Anal. Calcd For C21H15N3O (325.37): C, 77.52; H, 4.65; N, 12.91. Found: C, 77.23; H, 4.21; N, 12.42%.
N'-(Anthracen-9-ylmethylene)isonicotinohydrazide (7d). Yield 85%; mp 263–265 °C; IR (KBr) ν: 3205 (NH), 3086 (CHarom), 1693 (C=O), 1635 (C=N) cm−1; 1H NMR δ: 12.34 (s, 1H, NH), 9.70 (s, 1H, H-11), 8.88 (m, 2H, H-2',6'), 8.77 (d, 2H, H-1,8, J = 8.4 Hz), 8.75 (s, 1H, H-10), 8.18 (d, 2H, H-4,5, J = 8.8 Hz), 7.96 (d, 2H, H-3',5', J = 4.8 Hz), 7.68 (m, 2H, H-2,7), 7.61 (m, 2H, H-3,6); 13C NMR δ: 161.5 (C-14), 150.4 (C-2',6'), 148.3 (C-11), 140.4 (C-4'), 130.8 (C-4a,10a), 129.9 (C-10), 129.6 (C-8a,9a), 129.0 (C-4,5), 127.3 (C-2,7), 125.5 (C-3,6), 124.7 (C-1,8,9), 121.5 (C-3',5'); Anal. Calcd For C21H15N3O (325.37): C, 77.52; H, 4.65; N, 12.91. Found: C, 77.27; H, 4.79; N, 13.05%.
N'-(Anthracen-9-ylmethylene)-9-oxo-9,10-dihydroacridine-4-carbohydrazide (7e). Yield 36%; mp 324−327 °C; IR (KBr) ν: 3209 (NH), 3046 (CHarom), 1663 (C=Oacridone), 1622 (C=Oamide), 1587 (C=N) cm−1; 1H NMR δ: 12.47 (s, 1H, NH), 12.02 (s, 1H, H-10'), 9.76 (s, 1H, H-11), 8.87 (d, 2H, H-1,8, J = 8.8 Hz), 8.75 (s, 1H, H-10), 8.54 (d, 1H, H-1', J = 8.0 Hz), 8.47 (d, 1H, H-3', J = 6.8 Hz), 8.26 (d, 1H, H-8', J = 8.0 Hz), 8.17 (d, 2H, H-4,5, J = 8.4 Hz), 7.89 (d, 1H, H-5', J = 8.4 Hz), 7.76 (m, 1H, H-6'), 7.69 (m, 2H, H-2,7), 7.62 (m, 2H, H-3,6), 7.46 (m, 1H, H-2'), 7.33 (m, 1H, H-7'); 13C NMR δ: 176,5 (C-9'), 163.9 (C-14), 148.3 (C-11), 140.2 (C-4a',10a'), 134.0 (C-6'), 133.5 (C-3'), 130.9 (C-4a,10a), 130.6 (C-1'), 130.1 (C-10), 129.8 (C-8a,9a), 129.1 (C-4,5), 127.4 (C-2,7), 125.8 (C-8'), 125.6 (C-3,6), 124.8 (C-1,8), 124.5 (C-9), 122.1 (C-7'), 121.7 and 118.4 (C-4´ and C-9a'), 120.5 (C-8a'), 120.0 (C-2'), 118.7 (C-5'),; Anal. Calcd For C29H19N3O2 (441.49): C, 78.90; H, 4.34; N, 9.52. Found: C, 78.55; H, 4.13; N, 9.32%.
General procedure for the preparation of hydrazones 13a−g
To a solution of the appropriate aldehyde or ketone (1.147 mmol) in ethanol (10 mL), (acridin-9-yl) hydrazine (12, 200 mg, 0.956 mmol) and several drops of glacial acetic acid were added. The reaction mixture was then refluxed for 3 h following which it was cooled and poured onto chilled water. The resulting precipitate was filtered off, dried and the product recrystallized from methanol.
9-(Benzylidenehydrazono)-9,10-dihydroacridine (13a). Yield: 84%; mp 68−70 °C; IR (KBr) ν: 3271 (NH), 3101, 1626, 1597 (C=N) cm−1; 1H NMR δ: 10.83 (s, 1H, H-10), 8.99 (dd, 1H, H-1, J = 8.8, 1.6 Hz), 8.55 (s, 1H, HC=N), 8.40 (dd, 1H, H-8, J = 8.0, 1.2 Hz), 7.89 (dd, 2H, H-2',6', J = 8.8, 1.6 Hz), 7.55−7.48 (m, 5H, H-3,6,3'−5'), 7.30 (dd, 1H, H-5, J = 8.4, 1.2 Hz), 7.28 (dd, 1H, H-4, J = 8.4, 0.8 Hz), 7.14−7.05 (m, 2H, H-2,7); 13C NMR δ: 154.4 (C-13), 149.8 (C-9), 140.6 (C-4a), 138.6 (C-10a), 135.3 (C-1'), 132.3 (C-1), 131.4 (C-3), 130.9 (C-6), 130.0 (C-4'), 129.0 (C-3',5'), 128.6 (C-2',6'), 125.5 (C-8), 120.9 (C-7), 119.7 (C-2), 119.3 (C-8a), 116.2 (C-5), 115.6 (C-9a), 115.6 (C-4) ; Anal. Calcd For C20H15N3 (297.36): C, 80.78; H, 5.08; N, 14.13. Found: C, 80.54; H, 5.01; N, 13.85%.
9-(2,4,6-Trimethoxybenzylidenehydrazono)-9,10-dihydroacridine (13b). Yield 83%; mp 239−240 °C; IR (KBr) ν: 3267 (NH), 3107, 1620, 1599 (C=N) cm−1; 1H NMR δ: 10.60 (s, 1H, H-10), 9.87 (d, 1H, H-1, J = 8.4 Hz), 8.80 (s, 1H, HC=N), 8.41 (d, 1H, H-8, J = 8.0 Hz), 7.47 and 7.45 (m, m, 2H, H-3,6), 7.22 (d, 1H, J = 8.4 Hz) and 7.18 (d, 1H, J = 8.4 Hz, H-4,5), 7.05 and 7.04 (m, m, 2H, H-2,7), 6.35 (s, 2H, H-3',5'), 3.94 (s, 6H, C-2',6'-OCH3), 3.87 (s, 3H, C-4'-OCH3); 13C NMR δ: 162.6 (C-4'), 160.4 (C-2',6'), 150.9 (C-13), 148.8 (C-9), 140.2 (C-4a), 138.6 (C-10a), 133.2 (C-1), 131.0 (C-3), 130.4 (C-6), 125.5 (C-8), 120.5 (C-7), 119.6 (C-2), 119.2 (C-8a), 116.4 (C-5), 115.7 (C-9a), 115.3 (C-4), 104.8 (C-1'), 91.0 (C-3',5'), 56.0 (C-2',6'-OCH3), 55.4 (C-4'-OCH3); Anal. Calcd For C23H21N3O3 (387.44): C, 71.30; H, 5.46; N, 10.85. Found: C, 71.48; H, 5.53; N, 10.77%.
9-(4-Bromobenzylidenehydrazono)-9,10-dihydroacridine (13c). Yield 93%; mp 99−101 °C; IR (KBr) ν: 1630, 1597 (C=N) cm−1; 1H NMR δ: 10.89 (s, 1H, H-10), 8.91 (d, 1H, H-1, J = 8.0 Hz), 8.53 (s, 1H, HC=N), 8.39 (d, 1H, H-8, J = 7.6 Hz), 7.82 (m, 2H, H-2',6'), 7.72 (m, 2H, H-3',5'), 7.51 (m, 2H, H-3,6), 7.31 (d, 1H, J = 8.4 Hz) and 7.27 (d, 1H, J = 8.4 Hz, H-4,5), 7.12 and 7.07 (m, m, 2H, H-2,7); 13C NMR δ: 153.1 (C-13), 149.9 (C-9), 140.5 (C-4a), 138.5 (C-10a), 134.5 (C-1'), 132.2 (C-1), 131.9 (C-3',5'), 131.4 (C-6), 130.9 (C-3), 129.3 (C-2',6'), 125.4 (C-8), 123.2 (C-4'), 120.8 (C-7), 119.8 (C-2), 119.1 (C-8a), 116.1 (C-5), 115.6 (C-4), 115.4 (C-9a); Anal. Calcd For C20H14BrN3 (376.26): C, 63.85; H, 3.75; N, 11.17. Found: C, 63.58; H, 3.53; N, 10.84%.
9-(4-Methoxybenzylidenehydrazono)-9,10-dihydroacridine (13d). Yield 78%; mp 68−70 °C; IR (KBr) ν: 3269 (NH), 3130, 1624, 1597 (C=N) cm−1; 1H NMR δ: 10.73 (s, 1H, H-10), 9.05 (dd, 1H, H-1, J = 8.4, 1.2 Hz), 8.48 (s, 1H, HC=N), 8.37 (dd, 1H, H-8, J = 8.4, 1.2 Hz), 7.83 (m, 2H, H-2',6'), 7.51−7.46 (m, 2H, H-3,6), 7.27 (dd, 1H, J = 8.4, 1.2 Hz) and 7.23 (dd, 1H, J = 8.4, 0.8 Hz, H-4,5), 7.11−7.03 (m, 4H, H-2,7,3',5'), 3.84 (s, 3H, OCH3); 13C NMR δ: 160.8 (C-4'), 154.3 (C-13), 149.2 (C-9), 140.5 (C-4a), 138.5 (C-10a), 132.3 (C-1), 131.2 (C-6), 130.7 (C-3), 129.2 (C-2',6'), 127.9 (C-1'), 125.3 (C-8), 120.7 (C-7), 119.5 (C-2), 119.3 (C-8a), 116.0 (C-9a), 115.7 (C-5), 115.4 (C-4), 114.4 (C-3',5'), 55.3 (OCH3); Anal. Calcd For C21H17N3O (327.39): C, 77.04; H, 5.23; N, 12.83. Found: C, 76.38; H, 5.53; N, 12.64%.
9-(2,4,6-Trimethylbenzylidenehydrazono)-9,10-dihydroacridine (13e). Yield 41%; mp 79−81 °C; IR (KBr) ν: 3259 (NH), 3103, 1628, 1595 (C=N) cm−1; 1H NMR δ: 10.78 (s, 1H, H-10), 9.06 (dd, 1H, H-1, J = 8.0, 1.2 Hz), 8.87 (s, 1H, HC=N), 8.42 (dd, 1H, H-8, J = 8.0, 1.6 Hz), 7.49 and 7.47 (m, m, 2H, H-3,6), 7.27 (dd, 1H, J = 8.0, 0.4 Hz) and 7.25 (dd, 1H, J = 7.6, 0.8 Hz, H-4,5), 7.10 (ddd, 1H, J = 8.0, 7.2, 1.2 Hz H-7), 6.96 (m, 3H, H-2,3',5'), 2.51 (s, 6H, C-2',6'-CH3), 2.28 (s, 3H, C-4'-CH3); 13C NMR δ: 155.6 (C-13); 149.6 (C-9); 140.3 (C-4a); 138.6 (C-10a); 138.4 (C-4'), 137.5 (C-2',6'), 131.8 (C-1), 131.3 (C-3), 130.7 (C-6), 129.5 (C-3',5'), 128.9 (C-1'), 125.5 (C-8); 120.7 (C-7); 119.2 (C-8a); 119.2 (C-2); 116.0 (C-5); 115.6 (C-9a); 115.5 (C-4); 21.3 (C-2',6'-CH3), 20.7 (C-4'-CH3); Anal. Calcd For C23H21N3 (339.44): C, 81.38; H, 6.24; N, 12.38. Found: C, 80.98; H, 5.94; N, 12.06%.
9-(Propan-2-ylidenehydrazono)-9,10-dihydroacridine (13f). Yield 38%; mp 76−78 °C; IR (KBr) ν: 3277 (NH), 3132, 2995, 2939 (CH), 1622, 1595 (C=N) cm−1; 1H NMR δ: 10.43 (s, 1H, H-10), 8.74 (d, 1H, H-1, J = 7.6 Hz), 8.24 (d, 1H, H-8, J = 7.6 Hz), 7.40 (m, 2H, H-3,6), 7.18 and 7.15 (m, m, 2H, H-4,5), 7.02 (m, 1H, H-7), 6.94 (m, 1H, H-2), 2.12 (s, 3H, CH3), 1.99 (s, 3H, CH3); 13C NMR δ: 158.0 (C-13), 144.2 (C-9), 140.5 (C-4a), 138.4 (C-10a), 131.7 (C-1), 130.7 (C-3), 130.1 (C-6), 125.2 (C-8), 120.5 (C-7), 119.9 (C-8a), 119.0 (C-2), 115.9 (C-9a), 115.6 (C-4), 115.2 (C-5), 24.6 (CH3), 17.9 (CH3); Anal. Calcd For C16H15N3 (249.32): C, 77.08; H, 6.06; N, 16.85. Found: C, 76.88; H, 5.94; N, 17.06%.
9-((1-Phenylethylidene)hydrazono)-9,10-dihydroacridine (13g). Yield 42%; mp 86−89 °C; IR (KBr) ν: 2923, 1638, 1613 (C=N) cm−1; 1H NMR δ: 10.68 (s, 1H, H-10), 8.58 (d, 1H, H-1, J = 7.6 Hz), 8.38 (d, 1H, H-8, J = 7.6 Hz), 7.97 (m, 2H, H-2',6'), 7.52−7.45 (m, 5H, H-3,6,3'−5'), 7.24 (m, 2H, H-4,5), 7.11 (m, 1H, H-7), 6.95 (m, 1H, H-2), 2.46 (s, 3H, CH3); 13C NMR δ: 155.8 (C-13), 145.5 (C-9), 140.6 (C-4a), 138.4 (C-10a), 138.3 (C-1´), 131.5 (C-1), 131.1 (C-3), 130.5 (C-6), 129.1 (C-4'), 128.5 (C-3',5'), 126.0 (C-2',6'), 125.4 (C-8), 120.7 (C-7), 119.8 (C-8a), 119.4 (C-2), 115.7 (C-5), 115.4 (C-4), 115.4 (C-9a), 14.3 (CH3); Anal. Calcd For C21H17N3 (311.39): C, 81.00; H, 5.50; N, 13.49. Found: C, 79.83; H, 5.34; N, 13.46%.
ACKNOWLEDGEMENTS
Financial support for this work by the Slovak Grant Agency VEGA (Grants No. 1/0476/08, 1/0053/08), Slovak State NMR Program (Grant No. 2003SP200280203) and Turun Yliopistosäätiö is gratefully acknowledged. We also thank the Center for Scientific Computing (CSC) for computational support and services.
References
1. a) D. Sabolová, M. Kožurková, P. Kristian, I. Danihel, D. Podhradský, and J. Imrich, Int. J. Biol. Macromol., 2006, 38, 94; CrossRef b) Z. Fröhlichová, J. Tomaščiková, J. Imrich, P. Kristian, I. Danihel, S. Böhm, D. Sabolová, M. Kožurková, and K. D. Klika, Heterocycles, 2009, 77, 1019; CrossRef c) M. Kožurková, D. Sabolová, H. Paulíková, L. Janovec, P. Kristian, M. Bajdichová, J. Buša, D. Podhradský, and J. Imrich, Int. J. Biol. Macromol., 2007, 41, 415; CrossRef d) M. Kožurková, D. Sabolová, L. Janovec, J. Mikeš, J. Kovaľ, J. Ungvarský, M. Štefanišinová, P. Fedoročko, P. Kristian, and J. Imrich, Bioorg. Med. Chem., 2008, 16, 3976; CrossRef e) Z. Gažová, A. Bellová, Z. Daxnerová, J. Imrich, P. Kristian, J. Tomaščiková, J. Bágeľová, D. Fedunová, and M. Antalík, Eur. Biophys. J., 2008, 37, 1261; CrossRef f) S. Hamuľaková, P. Kristian, D. Jun, K. Kuča, J. Imrich, I. Danihel, S. Böhm, and K. D. Klika, Heterocycles, 2008, 76, 1219; CrossRef g) J. Tomaščiková, J. Imrich, I. Danihel, S. Böhm, P. Kristian, J. Pisarčíková, M. Sabol, and K. D. Klika, Molecules, 2008, 13, 501; CrossRef h) J. Tomaščiková, I. Danihel, S. Böhm, J. Imrich, P. Kristian, I. Potočňák, J. Čejka, and K. D. Klika, J. Mol. Struct., 2008, 875, 419; CrossRef i) K. D. Klika, E. Balentová, J. Bernát, J. Imrich, M. Vavrušová, E. Kleinpeter, K. Pihlaja, and A. Koch, J. Heterocycl. Chem., 2006, 43, 633; CrossRef j) K. D. Klika, E. Balentová, J. Bernát, J. Imrich, M. Vavrušová, K. Pihlaja, A. Koch, E. Kleinpeter, A. Kelling, and U. Schilde, ARKIVOC, 2006, (xvi), 93; k) K. D. Klika, J. Bernát, J. Imrich, I. Chomča, R. Sillanpää, and K. Pihlaja, J. Org. Chem., 2001, 66, 4416; CrossRef l) J. Bernát, E. Balentová, P. Kristian, J. Imrich, E. Sedlák, I. Danihel, S. Böhm, N. Prónayová, K. Pihlaja, and K. D. Klika, Coll. Czech. Chem. Commun., 2004, 69, 833; CrossRef m) K. D. Klika, J. Imrich, M. Vilková, J. Bernát, and K. Pihlaja, J. Heterocycl. Chem., 2006, 43, 739; CrossRef n) P. Kristian, E. Balentová, J. Bernát, J. Imrich, E. Sedlák, I. Danihel, S. Böhm, N. Prónayová, K. D. Klika, K. Pihlaja, and J. Baranová, Chem. Pap., 2004, 58, 268; o) S. Böhm, J. Tomaščiková, J. Imrich, I. Danihel, P. Kristian, A. Koch, E. Kleinpeter, and K. D. Klika, submitted to Theochem.-J. Mol. Struct., 2009; p) J. Imrich, J. Tomaščiková, I. Danihel, P. Kristian, S. Böhm, I. Potočňák, J. Kožíšek, and K. D. Klika, Heterocycles, 2010, 80, 489. CrossRef
2. Z. Fröhlichová, J. Imrich, I. Danihel, P. Kristian, S. Böhm, D. Sabolová, M. Kožurková, O. Hritzová, B. Horváth, T. Bušová, and K. D. Klika, Spectrochim. Acta, Part A, 2009, 73, 238. CrossRef
3. E. Balentová, J. Imrich, J. Bernát, L. Suchá, M. Vilková, N. Prónayová, P. Kristian, K. Pihlaja, and K. D. Klika, J. Heterocycl. Chem., 2006, 43, 645. CrossRef
4. J. E. Sinsheimer, V. Jagodic, J. Polak, D. D. Hong, and J. A. Burckhalter, J. Pharm. Sci., 1975, 64, 925. CrossRef
5. M. Demeunynck, F. Charmantray, and A. Martelli, Curr. Pharm. Des., 2001, 7, 1703. CrossRef
6. a) K. D. Klika, L. Janovec, J. Imrich, G. Suchár, P. Kristian, R. Sillanpää, and K. Pihlaja, Eur. J. Org. Chem., 2002, 1248; CrossRef b) K. D. Klika, P. Valtamo, L. Janovec, G. Suchár, P. Kristian, J. Imrich, H. Kivelä, J. Alföldi, and K. Pihlaja, Rapid Commun. Mass Spectrom., 2004, 18, 87; CrossRef c) L. Janovec, S. Böhm, I. Danihel, J. Imrich, P. Kristian, and K. D. Klika, Collect. Czech. Chem. Commun., 2007, 72, 1435. CrossRef
7. a) R. M. Issa, A. A. Hassanein, I. M. El-Mehasseb, and R. I. A. El-Wadoud, Spectrochim. Acta, Part A, 2006, 65, 206; CrossRef b) V. V. Syakaev, S. N. Podyachev, B. I. Buzykin, S. K. Latypov, W. D. Habicher, and A. I. Konovalov, J. Mol. Struct., 2006, 788, 55. CrossRef
8. a) T. Baohe, H. Meizi, T. Shixing, H. Indira, T. Zhiwu, L. Jiebo, J. Yinxue, and Y. Ming, Bioorg. Med. Chem. Lett., 2009, 19, 2162; CrossRef b) N. C. Romeiro, G. Aguirre, P. Hernández, M. González, H. Cerecetto, I. Aldana, S. Pérez-Silanes, A. Monge, E. J. Barreiro, and L. M. Lima, Bioorg. Med. Chem., 2009, 17, 641; CrossRef c) N. K. Singh, N. Agrawal, and R. C. Aggarwal, Indian J. Chem. Sect. A, 1984, 23, 1011.
9. A. Iwan, B. Kaczmarczyk, H. Janeczek, D. Sek, and S. Ostrowski, Spectrochim. Acta, Part A, 2007, 66, 1030. CrossRef
10. A. Bernthsen, Liebigs Ann. Chem., 1878, 192, 1. CrossRef
11. S. Bedel, G. Ulrich, and C. Picard, Tetrahedron Lett., 2002, 43, 1697. CrossRef
12. B. H. Klanderman, J. Org. Chem., 1966, 31, 2618. CrossRef
13. B. Adcock, 'Aminoacridines' in 'Acridines', Ed. R. M. Acheson, (from 'The Chemistry of Heterocyclic Compounds', ed. by A. Weissberger and E. C. Taylor), J. Wiley: New York, 1973, p. 109.
14. P. Kristian, J. Bernát, J. Imrich, E. Sedlák, J. Alföldi, and M. Čornanič, Heterocycles, 2001, 55, 279. CrossRef
15. A. DeLeenheer, J. E. Sinsheimer, and J. H. Burckhalter, J. Pharm. Sci., 1972, 61, 1659. CrossRef
16. J. Dey, J. L. Haynes III, I. M. Warner, and A. K. Chandra, J. Phys. Chem. A, 1997, 101, 2271 and references cited therein. CrossRef
17. a) J. Černák, P. Kristian, J. Bernát, and J. Lipkowski, Acta Crystallogr., Sect. C, 1995, C51, 2397; CrossRef b) A. Linden, J. Guspanová, J. Bernát, and P. Kristian, Acta Crystallogr., Sect. C, 1997, C53, 732. CrossRef
18. K. R. Shoemaker, R. L. Cysyk, S. Padmanabhan, H. B. Bhat, and L. Malspeis, Drug Metab. Dispos., 1982, 10, 35.
19. P. Virta, A. Koch, M. U. Roslund, P. Mattjus, E. Kleinpeter, L. Kronberg, R. Sjöholm, and K. D. Klika, Org. Biomol. Chem., 2005, 3, 2924. CrossRef
20. J. Mäki, P. Tähtinen, L. Kronberg, and K. D. Klika, J. Phys. Org. Chem., 2005, 18, 240. CrossRef
21. M. J. S. Dewar, E. G. Zoebisch, E. F. Healy, and J. J. P. Stewart, J. Am. Chem. Soc., 1985, 107, 3902. CrossRef
22. A. D. Becke, J. Chem. Phys., 1993, 98, 5648. CrossRef
23. M. J. Frisch, G. W. Trucks, H. B. Schlegel, G. E. Scuseria, M. A. Robb, J. R. Cheeseman, J. A. Montgomery Jr., T. Vreven, K. N. Kudin, J. C. Burant, J. M. Millam, S. S. Iyengar, J. Tomasi, V. Barone, B. Mennucci, M. Cossi, G. Scalmani, N. Rega, A. Petersson, H. Nakatsuji, M. Hada, M. Ehara, K. Toyota, R. Fukuda, J. Hagesawa, M. Ishida, T. Nakajima, Y. Honda, O. Kitao, H. Nakai, M. Klene, X. Li, J. E. Knox, H. P. Hratchian, J. B. Cross, C. Adamo, J. Jaramillo, R. Gomperts, E. Stratmann, O. Yazyev, A. J. Austin, R. Cammi, C. Pomelli, J. W. Ochterski, P. Y. Ayala, K. Morokuma, G. A. Voth, P. Salvador, J. J. Dannenberg, V. G. Zakrzewski, S. Dapprich, A. D. Daniels, M. C. Strain, O. Farkas, D. K. Malick, A. D. Rabuck, K. Raghavachari, J. B. Foresman, J. V. Ortiz, Q. Cui, A. G. Baboul, S. Clifford, J. Cioslowski, B. B. Stefanov, G. Liu, A. Liashenko, P. Piskorz, I. Komaromi, R. L. Martin, D. J. Fox, T. Keith, M. A. Al-Laham, C. Y. Peng, A. Nanayakkara, M. Challacombe, P. M. W. Gill, B. Johnson, W. Chen, M. W. Wong, C. Gonzales, and J. A. Pople, Gaussian 03, Revision B.05, Gaussian, Inc., Pittsburgh PA 2003.
24. L. K. Hanson, J. Fajer, M. A. Thompson, and M. C. Zerner, J. Am. Chem. Soc., 1987, 109, 4728. CrossRef