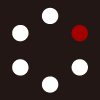
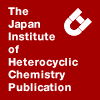
HETEROCYCLES
An International Journal for Reviews and Communications in Heterocyclic ChemistryWeb Edition ISSN: 1881-0942
Published online by The Japan Institute of Heterocyclic Chemistry
e-Journal
Full Text HTML
Received, 25th August, 2009, Accepted, 13th October, 2009, Published online, 14th October, 2009.
DOI: 10.3987/REV-09-657
■ Development of Epoxysilane Rearrangement and Its Application to Chirality Transfer
Michiko Sasaki, Hidaka Ikemoto, and Kei Takeda*
Divison of Medicinal Chemisry, Graduate School of Biomedical Sciences, Hiroshima University, 1-2-3 Kasumi, Minami-ku, Hiroshima 734-8551, Japan
Abstract
Development and synthetic application of epoxysilane rearrangement, a novel synthetic use of α,β-epoxysilanes, in which an anion-induced ring-opening of epoxide and Brook rearrangement in the resulting α-silyl alkoxide occur in a tandem fashion to provide a β-siloxy allyl anion, are described. Chirality transfer from epoxides to the position next to a nitrile group by taking advantage of the concerted process of the rearrangement is also reported.CONTENTS
1. Introduction
2. Background
3. Tandem base-promoted ring-opening/brook rearrangement/allylic alkylation of O-silyl cyanohydrins of β-silyl-α,β-epoxyaldehyde
4. Synthetic application of the epoxysilane rearrangement
5. Chirality transfer using epoxysilane rearrangement
1. INTRODUCTION
Although preparation of α,β-epoxysilanes was reported more than fifty years ago,1 they had attracted little attention for use in synthetic applications until Stork and co-workers showed that α,β-epoxysilanes can be converted to aldehydes and ketones via a ring-opening followed by desilylation (Scheme 1).2 Since then, they have been extensively used as versatile building blocks for organic synthesis.3 Among those reported, one of the most synthetically useful reactions is a ring-opening process, in which attack of a nucleophile occurs in a regioselective manner to produce diastereomerically pure β-hydroxysilanes (Scheme 2, 1 → 2).4 The process with α-ring opening provides a highly stereospecific olefin synthesis coupled with the stereospecific syn- and anti-elimination depending on the reaction conditions (2 → (E)- and (Z)-3).
On the other hand, when a nucleophile is a heteroatom with a leaving ability and the silyl group is bulky enough to suppress the α-attack, β-ring opening in α,β-epoxysilane 4 occurs to provide an enol silyl ether 5 stereospecifically via a Brook rearrangemen5 in the resulting α-oxidosilane followed by anti-elimination.6
A similar type of reaction via Brook rearrangement in α,β-epoxysilanes was first observed by Jung and coworkers.7 They found that treatment of epoxysilane 6 with t-BuLi produced aldehyde 7 via a tandem process that involves a base-induced β-ring opening followed by [1,2]- and [1,6]-silyl migration (Brook and [1,6]-retro-Brook rearrangement, respectively). This result was contrary to their expectation that oxetane derivative 8 would be obtained by 4-exo-epoxy mode cyclization.
Enantiomerically pure epoxides are now among the most important intermediates for organic synthesis because of their ready availability and high versatility.8 Their chiralities have been extensively utilized for the establishment of one or more than two chiral centers via ring-opening reactions. However, when we started studies on α,β-epoxysilanes, there was no literature precedent for use of epoxides as a source for chiral carbanions.
In this review, we focus on a novel synthetic use of α,β-epoxysilanes, in which an anion-induced ring-opening of epoxide and Brook rearrangement in the resulting α-silyl alkoxide occur in a tandem fashion to provide a β-siloxy allyl anion, which we call epoxysilane rearrangement,9 and its application to chirality transfer reactions.
2. BACKGROUND
We conceived the idea of using α,β-epoxysilanes as a versatile building block, when trying to extend Brook rearrangement-mediated [3 + 4] annulation,10 which uses a combination of acryloylsilanes 9 and methyl ketone enolates 10, for the construction of eight-membered rings. Thus, we considered that the eight-membered ring 15 can be obtained by an anionic oxy-Cope rearrangement of divinylcyclobutanolate derivative 14, which could be derived from 13 via Brook rearrangement followed by cyclization, which in turn was prepared by the reaction of epoxysilane 12 with alkenyl methyl ketone enolate 10. We have tried a variety of combinations of an epoxysilane and a nucleophile, but almost all resulted in decomposition, recovery of the starting materials, or ring-opening on the undesired side.11
Although we could not establish the viability of the tandem sequence that involves β-ring opening followed by a Brook rearrangment in the reaction of α,β-epoxysilanes with a nucleophile, the idea has been realized in a different form. During the course of extension of Brook rearrangement-mediated [3 + 2] annulation12 to asymmetric versions, we had occasion to examine the reaction of β-silyl-α,β-epoxyacylsilanes 16 with enolate of alkyl methyl ketone 17. Although we expected the formation of cyclopentenol derivatives 22 via a two-fold Brook rearrangement followed by an intramolecular nucleophilic addition ((E)-19 → 22, dashed arrow), the major products were uncyclized enol silyl ether 20 and cyclopropanediol derivative 21.13 The former is formed by intramolecular proton transfer before a second Brook rearrangement in (E)-19, while the latter is formed as a result of an intramolecular allylic attack on the carbonyl group by a siloxy carbanion generated by the second Brook rearrangement in (Z)-19 (Scheme 6).
This result indicates the possibility of the reaction of an epoxysilane 23 bearing an anion-stabilizing electron-withdrawing group at the α-position with an amide base in the presence of an electrophile. If a tandem process that involves a base-promoted isomerization of the epoxide (24 → 25), Brook rearrangement (25 → 26), and a reaction of the resulting allylic anion with an electrophile (26 → 27 → 28) proceeds well, the epoxysilane 23 would function as a homoenolate equivalent14 with synthetically useful functionality (Scheme 7).
3. TANDEM BASE-PROMOTED RING-OPENING/BROOK REARRANGEMENT/ALLYLIC ALKYLATION OF O-SILYL CYANOHYDRINS OF β-SILYL-α,β-EPOXYALDEHYDE
We first focused on O-silyl cyanohydrins of β-silyl-α,β-epoxyaldehydes 29a,b as a precursor for the species of 27, in which a nitrile group is an electron-withdrawing group, because of its ease of preparation. The preparation of 29a and 29b was shown in Scheme 8.
When 29a and 29b were treated with LDA (1.1 equiv) in THF at –80 °C in the presence of MeI (1.2 equiv) for 5 min, α-methylated cyanohydrins 30, products formed via a tandem sequence (24 → 28), were obtained in 82% and 84% yields, respectively (Table 1).9
It is particularly noteworthy that (1) the reactions were completed within 5 min at –80 °C and alkylation products of possible intermediates other than 30 were not detected and (2) E/Z ratios of 30 obtained from the two diastereomers were markedly different. These facts prompted us to conduct stereochemical and mechanistic studies on the reaction. Summarizing the results obtained from the studies, (1) the mode of ring opening of the epoxide is anti,15 which is based on competitive experiments using the diastereomeric cyanohydrins 29a and 29b, (2) experiments using epoxysilanes with a different silyl group showed that silicon-oxygen bond formation is involved in the rate-determining transition state 31, and (3) there is no equilibrium between (E)- and (Z)-siloxyallyl anions 32, which are candidates for immediate precursors for the alkylation.
Based on these and other results, we have proposed a reaction pathway that involves silicate intermediates (Z)-33 and (E)-33, which are formed from 29a and 29b, respectively, by an anti ring-opening followed by the formation of an oxygen-silicon bond (Scheme 9). The silicates are converted into rotamers (Z)-33a,b and (E)-33a,b, respectively, after rotation around the C3-C4 bond in such a way that the C4-silicon bond can adopt a parallel arrangement with the π orbitals of the double bond. They are transformed into the products (E)- and (Z)-34 directly via concerted alkylation and/or via allyl anion intermediates (Z)-32 and (E)-32. Among silicates (Z)-33a, 33b and (E)-33a, 33b, only (Z)-33a can form an internally chelated structure with a metal cation. This pathway can explain the fact that (Z)-isomer is obtained in higher ratio from diastereomer 29a.
4. SYNTHETIC APPLICATION OF THE EPOXYSILANE REARRANGEMENT
Since we have published a review on mechanistic studies of epoxysilane rearrangements and their application as synthetic reactions,16,17 we will touch upon the title subject in this section only briefly. For the generation of an anion at the γ-position of α,β-epoxysilanes that triggers the tandem process, we devised three methods, (1) deprotonation, (2) reaction of acylsilanes with a nucleophile followed by Brook rearrangement, and (3) a conjugate addition of a nucleophile to an enoate system bearing an epoxysilane moiety at the α-position.
A β-siloxy carbanion generated in the nitrile derivative 35 can participate in a Michael-Initiated-Ring-Closure type reaction with bisenoate 36 to give 37a,b (Scheme 10).18 The use of phosphonio group instead of a nitrile group as the electron-withdrawing group resulted in the formation of siloxydiene derivatives 39 via Wittig reaction (38 → 39).19 When the group next to the epoxysilane moiety is an anion-stabilizing leaving group such as tosyl, the epoxysilane 40 can function as an acrolein β-anion equivalent to give β-substituted acrolein derivatives 41 and 42.20
We previously reported that reactions of acylsilanes with KCN under phase-transfer catalytic conditions proceeded smoothly via Brook rearrangement to produce O-silylated cyanohydrin derivatives in excellent yields.21 These results prompted us to focus our attention on the reaction of acylsilanes with cyanide ion as a method for the generation of a carbanion at the γ-position to the epoxysilane. Reaction of δ-silyl-γ,δ-epoxy-α,β-unsaturated acylsilanes 43 with KCN in the presence of chloro- or cyanoformates and 18-crown-6 gave highly functionalized dienol silyl ether derivatives 44 via a two-fold Brook rearrangement-induced tandem sequence (Scheme 11).22
A carbanion generated by Michael addition of a lithium enolate of α-chloroacetamide to enoate 45 bearing an epoxysilane moiety at the α-postion can also participate via the same type tandem sequence to give 46 with stereoselectivity that can be explained by the formation of chelation structures (Scheme 12).23
5. CHIRALITY TRANSFER USING EPOXYSILANE REARRANGEMENT
As mentioned above, epoxysilane rearrangement was discovered during extension of the Brook rearrangement-mediated [3 + 2] annulation to its enantioselective version. The key of the idea is to use the epoxide chirality as a precursor for chiral carbanions via a concerted process involving Brook rearrangement (47 → 48 → 49), which would allow enantioselective C-C bond formation in the reaction with an electrophile (Scheme 13).
We recognized the possibility of its application to chirality transfer from the fact that the diastereomeric epoxysilanes 29a and 29b give 34 in markedly different E/Z ratios in the reaction with a base in the presence of an electrophile. Preliminary experiments using a combination of homochiral epoxysilane 29a, LDA, and benzyl bromide resulted in complete racemization. We attributed this failure to the slower reaction of a generated chiral carbanion with an electrophile because the process involves a configurationally extremely unstable α-nitrile carbanion and the process is an intermolecular one.
5.1. INTRAMOLECULAR TRAPPING OF CHIRAL CARBANIONS GENERATED VIA EPOXYSILANE REARRANGEMENT BY [2,3]-WITTIG REARRANGEMENT
We next changed the reaction with an electrophile from an intermolecular process to an intramolecular one with the expectation of acceleration of the trapping reaction of the carbanion and then focused on [2,3]-Wittig rearrangement.24 After extensive examination of various substrates, we chose the 2-naphthyl (Nap) derivatives 50a and 50b, which were obtained from the corresponding epoxy aldehyde and provided the best results in terms of separability of diastereomers. When enantiomerically enriched 50a was treated with n-BuLi (2.0 equiv) in THF, the most common solvent for [2,3]-Wittig rearrangement, for 5 min at –80 ° to –75 °C, the tandem rearrangement products (E)- and (Z)-51 were obtained in 46% yield and in racemic form (Table 2, entry 1). Although the asymmetric induction remained poor in the solvent at higher temperatures (entry 3), change in the solvent to other ethereal solvents resulted in dramatic improvement in asymmetric induction (entries 5-8). The use of 1,4-dioxane provided excellent enantiomeric purity (entries 7 and 8).25
Reaction of diastereomer 50b proceeded in a similar manner to give (R,E)- and (S,Z)-51, which are enantiomeric to those obtained from 50a, respectively (Table 3).
Particularly noteworthy is that chirality of a carbanion derived from the epoxide, conjugated with both a naphthyl group and a double bond, can be retained even above room temperature. The observed stereospecificity suggests (1) the formation of a carbanion precursor in a concerted manner followed by [2,3]-Wittig rearrangement before racemization (Scheme 14, 52a → 53 → 54 → 55) or (2) the direct formation of [2,3]-Wittig rearrangement products from a silicate derivative, a possible intermediate in the Brook rearrangement, without involvement of a carbanion intermediate (52a → 53 → 55)(Scheme 14).
To obtain information about the configurational stability of a carbanion between an aryl group and a double bond, we examined [2,3]-Wittig rearrangement of enantiomerically enriched (S)-56, in which racemization may be more facile. Although no asymmetric induction was observed in THF, reactions in 1,4-dioxane were found to show the best enantioselectivity again to give (R)-57 (Table 4, entries 1 and 4). It is notable that less enantioselectivity was observed at a lower temperature in Et2O (entries 2 and 3), suggesting that the loss of chirality is at least partly attributed to the racemization due to decreased rate of the [2,3]-Wittig rearrangement at a lower temperature. These results are consistent with the former possibility, although the latter possibility of tandem epoxysilane rearrangement/[2,3]-Wittig rearrangement cannot be ruled out.
The origin of the excellent enantioselectivity observed in 1,4-dioxane is unclear at present, but it may be due to the decreased rate of racemization by chelation of the lithium cation with the two oxygens. This is supported by the fact that the addition of HMPA in 1,4-dioxane (entry 5) and the use of 1,3-dioxane (entry 6) resulted in complete racemization.
5.2. CHIRALITY TRANSFER IN THE CONSTRUCTION OF SEVEN-MEMBERED CARBOCYCLES USING A COMBINATION OF BROOK REARRANGEMENT-MEDIATED [3 + 4] ANNULATION AND EPOXYSILANE26
The fact that the chirality of the epoxides can be trapped intramolecularly without complete racemization even through allyl rearrangement prompted us to examine the construction of seven-membered ring systems using a combination of Brook rearrangement-mediated [3 + 4] annulation and epoxysilane rearrangement. Thus, [3 + 4] annulation using 58 instead of acryloylsilane 9 (Scheme 5) as a three-carbon unit would afford highly functionalized cycloheptenone derivatives 62 via a tandem process that involves a two-fold Brook rearrangement (59 → 60 and 60 → 61) as shown in Scheme 15. The strategy relies on our findings that once the divinylcyclopropanediolate is formed, the next anionic oxy-Cope rearrangement proceeds very rapidly and that vinylcyclopropanol 21 is formed in the reaction of 16 with methyl ketone enolate 17. In the annulation, we expected remote chirality transfer from the epoxide to the seven-membered ring by taking advantage of the concerted nature of the processes.
When lithium enolate 10a-e was added to a THF solution of racemic 58 at –80 °C and the mixture was allowed to warm to 0 °C, the expected [3 + 4] annulation product 62a-e was isolated in 31-60% yields as a single diastereomer (Table 5).
We first conducted [3 + 4] annulation using enantiomerically enriched (-)-58 (90% ee) and 10a under the same conditions as those employed for the racemic one to give 62a with 19% ee. This result suggests that the epoxide chirality can be transferred to remote positions without complete racemization via an intramolecular process. One of the origins of the low enantioselectivity was thought to be partial racemization in 61 via ring opening-closure of the cyclopropane, because we have observed that a ring-opening and ring-closing process is quite facile and competes with anionic oxy-Cope rearrangement.10 Therefore, we verified this by the reaction of (-)-58 with lithium enolate of isopropyl methyl ketone, in which anionic oxy-Cope rearrangement cannot occur. The reaction resulted in complete racemization to give vinylcylopropandiolate 63 in 42% yield together with uncyclized enol silyl ethers 64 (Scheme 16). This result suggests that the acceleration of anionic oxy-Cope rearrangement could suppress the racemization.
Our previous observation that the use of enolate of cycloalkenone in [3 + 4] annulation accelerates the anionic oxy-Cope rearrangement because of its fixed conformation, which is suitable for the rearrangement,27 led us to examine [3 + 4] annulation using an enolate of cycloalkenone. When (-)-58 was treated with potassium enolate 65 of 2-cycloheptenone, [3 + 4] annulation proceeded to give 66 in 35% yield with 62% ee (Scheme 17). As expected, the enantiomeric excess was greatly improved. Transformation of 66 into a functionalized eight-membered carbocycle 68 could be achieved in a manner similar to that reported previously.27
We next became interested in a new variation of the [3 + 4] annulation using an opposite combination of the three and the four carbon units (69 + 9), in which an epoxysilane moiety is incorporated in four-carbon unit 69, because the epoxysilane can be prepared more easily than 58 (Scheme 18). The crucial step of this strategy should be 1,4-O-to-O silyl migration in 70, which allows the formation of the intermediate 61, which is exactly the same as that obtained from the normal version of [3 + 4] annulation (58 + 10), via epoxysilane rearrangement (71 → 72). We have also observed a similar 1,4-O-to-O migration of silyl group.28 This annulation, in principle, generates four types of divinylcyclopropanolates, 70, 71, 61 and 73, depending on the timing of the epoxysilane rearrangement and the 1,4-O-to-O silyl migration, which should provide seven-membered carbocycles 74, 75, 62, 76, respectively, via an anionic oxy-Cope rearrangement.
When lithium enolate of 77 was treated with 78a at –80 °C and allowed to warm to 0 °C, 79 (a 1:1 diastereomeric mixture), an oxy-Cope rearrangement product of 70 before 1,4-O-to-O silyl migration, was obtained as a sole identifiable product in 50% yield (Table 6, entry 1). On the other hand, the use of sodium enolate prepared with NaHMDS resulted in the formation of desired cycloheptenone 80a in 43% yield in addition to 79 (16%) (Table 6, entry 2). Since this result can be interpreted as the consequence of acceleration of the 1,4-O-to-O silyl migration by an increase in the ionic character of a counter cation, we examined the reaction using 78b (R3Si = PhMe2Si) with the expectation of further acceleration of the 1,4-O-to-O silyl migration by introduction of a phenyl group to the silyl group. Treatment of sodium enolate of 77 with 78b under the same conditions afforded 80b in an improved yield, 65% (Table 6, entry 3). Although formation of 79 could not be detected, instead 81, a two-fold 1,4-O-to-O silyl migration product, was formed in 11% yield. These results indicate that the product distributions are a subtle balance among the relative rates of epoxysilane rearrangement, anionic oxy-Cope rearrangement and 1,4-O-to-O silyl migration in 70 and 61.
A relay of stereochemistry in the second version of the [3 + 4] annulation provided better ee than those obtained with the normal version of [3 + 4] annulation in the case of TBS derivative 78a, the origin of which is not clear at present (Table 7).
5.3. CHIRALITY TRANSFER FROM EPOXIDE TO α-NITRILE CARBANION
Based on the above findings, we next proceeded to examine intermolecular trapping of chiral α-nitrile carbanions generated via Brook rearrangement using O-carbamoyl cyanohydrins 82. We attributed the unsuccessful result in the chirality transfer using enantioenriched 29a to the intervention of a pathway via an α-nitrile-stabilized carbanion intermediate. Chiral carbanions that are conjugated with carbon-oxygen or carbon-nitrogen multiple bonds have been considered to be extremely difficult to generate due to their high proclivity toward racemization. To the best of our knowledge, reports by Walborsky and co-workers, who found that a chiral cyclopropyl nitrile derivative could be enantiospecifically deuterated in basic CH3OD, are the only example.29 They also found that the corresponding chiral aliphatic nitrile derivative suffered extensive racemization under the same conditions. We envisioned that the carbamoyloxy group (Cb)30 would decelerate the rate of racemization of the α-nitrile allylic carbanion by its strong chelating ability.
On the basis of exploratory experiments to find conditions in which the best result in terms of chemical and optical yields is obtained with LDA in the presence of benzyl bromide in Et2O or toluene at -80 °C, we examined the reactions of 82a and 82b with LDA in the presence of BnBr in Et2O and toluene with reaction times of 1 min and 60 min (Table 8).31 The latter reaction time was selected considering the possibility of a relatively slow reaction rate of the silicate intermediate and/or the chiral carbanion with benzyl bromide. The ees of (R,Z)-83 and (R,E)-83 in the reactions in Et2O were improved by quenching for 1 min, but at the expense of the chemical yield, while in toluene the chemical and optical yields were not greatly affected by the reaction time. This result can be interpreted in terms of a relatively slow reaction rate of the silicate intermediate and/or the chiral carbanion with benzyl bromide in Et2O. The optical yields in the reaction of 82b were lower than those in the reaction of 82a.
To examine the possibility of the intermediacy of a discrete chiral α-nitrile carbanion in the reaction of 82a,b, we performed benzylation reaction of O-carbamoyl cyanohydrin 85. When LDA was added to a solution of 85 and benzyl bromide in Et2O at –80 °C and then quenched for 1 min, benzylated product 86 was obtained in 35% chemical yield and 5% ee (Table 9). While prolongation of the reaction time to 60 min gave almost the same ee, the use of toluene as a solvent gave somewhat higher ee (11%). These results suggest the intermediacy of a discrete chiral α-nitrile carbanion that can be trapped intermolecularly with an electrophile without complete racemization, although the ees were low. Consequently, the partial chirality transfer observed in the above epoxysilane rearrangement should be based on both a concerted alkylation process from the silicate intermediate and the presence of the carbamoyl group, although the former process should be mainly responsible for the chirality transfer.
To gain further insight into the stereochemical course of this reaction, particularly alkylation of silicates 33 (33 → 34, Scheme 9), we trapped the α-silyl alkoxide intermediates corresponding to 25 (Scheme 7).32 We envisioned that clarification of the correlation between the double bond geometry of 87 and the double bond geometry and absolute stereochemistry at the C-2 position of 83 is essential for understanding the chirality transfer observed. After extensive experimentation, we were able to isolate (E,Z)-87 by performing the reaction in Et2O at a lower temperature. We have sometimes observed that the rate of Brook rearrangement in Et2O is much slower than that in THF. Treatment of 82a with LDA at –98 °C in Et2O afforded (E)-87 in 59% yield. A similar result was obtained with 82b to give (Z)-87. The observed stereospecificity was consistent with a syn-mode ring opening of the epoxide, although we have suggested an anti-mode ring opening for similar reactions using the corresponding OTBS derivatives in THF.
When LDA (1.1 equiv) was added to an Et2O solution of (E)-87 at –80 °C in the presence of BnBr and the reaction was quenched after 1 h, (Z)-83 and (E)-83 were obtained in 85% and 8% yields, respectively, together with protonated product (Z)-84 (Scheme 19). In contrast to the results obtained in the reaction of 82a (30-40% ee), the ees of all products were low. Reaction of (Z)-87 provided (E)- and (Z)-83 with low ees in a reversed ratio.
The observed lower asymmetric induction in comparison with the cases in 82a,b can be rationalized by assuming competitive C-protonation at the C-2 position by 87 with benzylation. This hypothesis led us to examine proton transfer reactions using substoichiometric amounts of LDA. The results are shown in Table 10.
As expected, the enantiomeric excess increased with decreasing amount of LDA in the reactions of both (E)-and (Z)-87; thus, use of 0.1 equiv and 1.0 equiv of LDA gave the best and poorest results in terms of ee, respectively, and the best result with regard to chemical yield was obtained when 0.3 equiv of the base was used. The lower chemical yields when using stoichiometric amounts of the base may stem from base-induced decomposition in 84.
The above results prompted us to examine the reaction using much weaker bases than LDA in protic solvents, which would suppress base-induced decomposition and allow a rapid capture of the resulting anions. The best result was obtained by treating (Z)-87 with t-BuOLi in t-BuOH at room temperature, giving (E,S)-84 in 86% yield and 75% ee (Table 11, entry 8).
In order to understand the stereochemical outcome in the reactions of (E)- and (Z)-87, the origin of the stereospecificity depending on the E/Z-geometry of 87 should be rationalized. The E/Z-geometry in 84 would be solely controlled by the conformation of silicate intermediates 88a,b, which are generated via deprotonation by a base followed by attack of the oxyanion on the silicon atom, assuming that no E/Z isomerization occurs in the products 84 under the conditions and that C-Si bond cleavage in the silicates occurs in a concerted fashion while keeping a parallel alignment between the C-Si bond and the π-orbital (Scheme 20). On the other hand, the stereochemistry at the C-2 position would be regulated by both the double bond geometry and a syn/anti mode protonation to the C-Si bond in 88. For example, (E)-88a would afford (Z,R)-84 and (Z,S)-84 in syn- and anti-protonation, respectively.
The findings that (Z,S)-84 and (E,S)-84 were obtained from (E)-87 and (Z)-87 as a major product and as a sole product, respectively, can be explained on the basis of the results of ab initio calculations at the RI-MP2/6-31+G* level of theory (Scheme 21). Thus, an internally chelated conformation (E)-88a was found to be more stable than (E)-88b, while in the case of (Z)-derivatives, a local minimum identified was only (Z)-88a in which the lithium ion was chelated with the carbonyl oxygen. On the basis of the absolute configuration of the products 84, which were determined by the modified Mosher method, (E)-87 affords (Z,S)-84 and (E,R)-84 via anti-mode protonation of silicate intermediates (E)-89a and (E)-89b derived from (E)-88a and (E)-88b, respectively, according to the least motion principle, while (Z)-87 gives only (E,S)-84 via (Z)-89a derived from (Z)-88a. The origin for the observed anti-mode protonation might be related to steric effects of the bulky silyl group, to steroelectronic effects in the concerted process involving Brook rearrangement and protonation, and to electronic effects of the carbamoyloxy group.
The above results obtained with (E)- and (Z)-87 seem to provide us with a clear view of the mechanism of the second half of the tandem process in the reaction of 82a,b. However, the fact that (E)-83 and (Z)-83 that were obtained from (E)-82a have the same (R)-configuration at C-2 cannot be explained on the basis of the exclusive formation of (E)- or (Z)-88 from 87 via syn-ring opening of the epoxide. Assuming that the anti-mode protonation observed in the reactions of 87 is also applicable to the benzylation of 82a,b, both syn- and anti-mode ring-opening should occur in 82a,b. Since we have found that the mode of ring-opening depends on the solvent and temperature, a more definitive elucidation of the overall stereochemical process of the chirality transfer via the epoxysilane rearrangement must await more detailed mechanistic study, particularly for the first half of the process.
6. CONCLUSION
We have shown that epoxysilanes are useful and versatile building blocks in organic synthesis in combination with Brook rearrangement. Furthermore, the newly found rearrangement will provide a conceptually novel approach that allows construction of stereogenic centers at the position next to conjugative electron-withdrawing groups such as nitrile via a chiral carbanion.
ACKNOWLEDGEMENTS
This research was partially supported by a Grant-in-Aid for Scientific Research (B) (15390006 and 19390006), a Grant-in-Aid for Scientific Research on Priority Areas (17035054 and 18032049), an Exploratory Research (14657563), and a Grant-in-Aid for Young Scientists (B) (20790011) from the Ministry of Education, Culture, Sports, Science and Technology (MEXT). Acknowledgment is also made to the Uehara Memorial Foundation, the Naito Foundation, and the Sasakawa Scientific Research Grant from the Japan Science Society for their partial support. We thank the Research Center for Molecular Medicine, Faculty of Medicine, Hiroshima University and N-BARD, Hiroshima University for the use of their facilities.
References
1. J. J. Eisch and J. T. Trainor, J. Org. Chem., 1963, 28, 487. CrossRef
2. G. Stork and E. Colvin, J. Am. Chem. Soc., 1971, 93, 2080; CrossRef G. Stork and M. E. Jung, J. Am. Chem. Soc., 1974, 96, 3682. CrossRef
3. G. H. Whitham, Science of Synthesis, 2002, 4, 633.
4. P. F. Hudrlik, D. Peterson, and R. J. Rona, J. Org. Chem., 1975, 40, 2263; CrossRef P. F. Hudrlik, R. H. Schwartz, and A. K. Kulkarni, Tetrahedron Lett., 1979, 24, 2233. CrossRef
5. For reviews on the Brook rearrangement, see: M. A. Brook, ‘Silicon in Organic, Organometallic, and Polymer Chemistry,’ John Wiley & Sons, Inc., New York, 2000; A. G. Brook, A. R. Bassindale, ‘Rearrangements in Ground and Excited States,’ ed. by P. de Mayo, Academic Press, New York, 1980, pp. 149-221; A. G. Brook, Acc. Chem. Res., 1974, 7, 77; CrossRef For the use of the Brook rearrangement in tandem bond formation strategies, see: W. H. Moser, Tetrahedron, 2001, 57, 2065; CrossRef E. Schaumann and A. Kirschning, Synlett, 2007, 177; CrossRef Also, see: A. Ricci, A. Degl'Innocenti, Synthesis, 1989, 647; CrossRef P. C. Bulman Page, S. S. Klair, and S. Rosenthal, Chem. Soc. Rev., 1990, 19, 147; CrossRef H. Qi and D. P. Curran, ‘Comprehensive Organic Functional Group Transformations,’ ed. by A. R. Katritzky, O. Meth-Cohn, C. W. Rees, and C. J. Moody, Pergamon, Oxford, 1995, pp. 409-431; P. F. Cirillo and J. S. Panek, Org. Prep. Proc. Int., 1992, 24, 553; CrossRef A. F. Patrocinio and P. J. S. Moran, J. Braz. Chem. Soc., 2001, 12, 7. CrossRef
6. P. Cuadrado and A. M. González-Nogai, Tetrahedron Lett., 2000, 41, 1111; CrossRef P. Cuadrado and A. M. González-Nogai, Tetrahedron Lett., 1997, 38, 8117. CrossRef
7. M. E. Jung and C. J. Nichols, J. Org. Chem., 1996, 61, 9065. CrossRef
8. R. A. Johnson and K. B. Sharpless, Catalytic ‘Asymmetric Synthesis,’ ed. by I. Ojima, Wiley-VCH, New York, 1993, pp. 101-158; E. N. Jacobsen, ‘Catalytic Asymmetric Synthesis,’ I. Ojima, Wiley-VCH, New York, 1993, pp. 159-202; M. Frohn and Y. Shi, Synthesis, 2000, 1979. CrossRef
9. K. Takeda, E. Kawanishi, M. Sasaki, Y. Takahashi, and K. Yamaguchi, Org. Lett., 2002, 4, 1511; CrossRef M. Sasaki, E. Kawanishi, Y. Nakai, T. Matsumoto, K. Yamaguchi, and K. Takeda, J. Org. Chem., 2003, 68, 9330. CrossRef
10. K. Takeda, M. Takeda, A. Nakajima, and E. Yoshii, J. Am. Chem. Soc., 1995, 117, 6400; CrossRef K. Takeda, A. Nakajima, and E. Yoshii, Synlett, 1996, 753; CrossRef K. Takeda, D. Nakane, and M. Takeda, Org. Lett., 2000, 2, 1903; CrossRef K. Takeda, A. Nakajima, M. Takeda, Y. Okamoto, T. Sato, and E. Yoshii, T. Koizumi, and M. Shiro, J. Am. Chem. Soc., 1998, 120, 4947; CrossRef (e) K. Takeda, A. Nakajima, M. Takeda, E. Yoshii, J. Zhang, and R. K. Boeckman, Jr., Org. Synth., 1999, 76, 199; K. Takeda and Y. Ohtani, Org. Lett., 1999, 1, 677. CrossRef
11. For another approach to eight-membered carbocycles 15 via 13 and 14, see: K. Takeda, H. Haraguchi, and Y. Okamoto, Org. Lett., 2003, 5, 3705. CrossRef
12. K. Takeda, M. Fujisawa, T. Makino, E. Yoshii, and K. Yamaguchi, J. Am. Chem. Soc., 1993, 115, 9351; CrossRef K. Takeda, Y. Ohtani, E. Ando, K. Fujimoto, E. Yoshii, and T. Koizumi, Chem. Lett., 1998, 1157; CrossRef K. Takeda, K. Yamawaki, and N. Hatakeyama, J. Org. Chem., 2002, 67, 1786; CrossRef K. Takeda, K. Kitagawa, I. Nakayama, and E. Yoshii, Synlett, 1997, 251.
13. K. Takeda and Y. Ohnishi, unpublished results.
14. For reviews on homoenolate equivalents, see: H. Ahlbrecht and U. Beyer, Synthesis, 1999, 365; CrossRef I, Kuwajima and E. Nakamura, Top. Curr. Chem., 1990, 155, 1; D. Hoppe, Angew. Chem., Int. Ed. Engl., 1984, 23, 932; CrossRef N. H. Werstiuk, Tetrahedron, 1983, 39, 205; CrossRef N. H. Werstiuk, ‘Umpoled Synthons,’ ed. by T. A. Hase, John Wiley & Sons, New York, 1987, 173; Also, see: A. Debal, T. Cuvigny, and M. Larchevêque, Tetrahedron Lett., 1977, 18, 3187. CrossRef
15. While the transition state from 29a is more favorable than that from 29b in the syn-elimination in terms of less repulsive interactions between H-4 and the O-silyl cyanohydrin moiety (A-value for OSiMe3: 0.74; for CN: 0.2), in the case of anti-elimination, the transition state from 29b is more favorable. When a mixture of 29a (1 equiv) and 29b (1equiv) in THF was treated with LDA (1 equiv) in the presence of MeI (1 equiv) at –80 °C for 5 min, a 1.0:0.7 mixture of 29a and 29b was obtained in 40% yield together with 35% of 30 (R = Me), indicating that 29b is more reactive than is 29a. These results are consitent with anti-elimination.
16. M. Sasaki and K. Takeda, J. Synth. Org. Chem. Jpn., 2006, 64, 1148.
17. K. Takeda, Yakugaku Zassi, 2007, 127, 1399. CrossRef
18. T. Matsumoto, H. Masu, K. Yamaguchi, and K. Takeda, Org. Lett., 2004, 6, 4367. CrossRef
19. M. Sasaki, M. Horai, and K. Takeda, Tetrahedron Lett., 2006, 47, 9271. CrossRef
20. M. Sasaki and K. Takeda, Org. Lett., 2004, 6, 4849. CrossRef
21. K. Takeda and Y. Ohnishi, Tetrahedron Lett., 2000, 41, 4169. CrossRef
22. K. Tanaka and K. Takeda, Tetrahedron Lett., 2004, 45, 7859. CrossRef
23. N. Okamoto, M. Sasaki, M. Kawahata, K. Yamaguchi, and K. Takeda, Org. Lett., 2006, 8, 1889. CrossRef
24. For reviews on [2,3]-Wittig rearrangement, see: T. Nakai and K. Mikami, Chem. Rev., 1986, 86, 885; CrossRef J. A. Marshall, ‘Comprehensive Organic Synthesis,’ ed. by B. M. Trost and I. Fleming, Pergamon, Oxford, 1991, Vol. 3, pp. 975–1014; K. Mikami and T. Nakai, Synthesis, 1991, 594; CrossRef T. Nakai and K. Mikami, Org. React., 1994, 46, 105; T. Nakai and K. Tomooka, Pure Appl. Chem., 1997, 696, 595; CrossRef D. M. Hodgson, K. Tomooka, and E. Gras, ‘Organolithiums in Enantioselective Synthesis,’ ed. by D. M. Hodgson, Springer, Berlin, 2003, pp. 217-250. CrossRef
25. The solvent has not been used in [2,3]-Wittig rearrangement, probably because the rate of racemization of the carbanion has been thought to be much faster than that of the reaction with an electrophile at temperatures higher than its freezing point (11 °C)..
26. Y. Nakai, M. Kawahata, K. Yamaguchi, and K. Takeda, J. Org. Chem., 2007, 72, 1379. CrossRef
27. K. Takeda, Y. Sawada, and K. Sumi, Org. Lett., 2002, 4, 1031. CrossRef
28. K. Takeda, J. Nakatani, H. Nakamura, K. Sako, E. Yoshii, and K. Yamaguchi, Synlett, 1993, 841. CrossRef
29. H. M. Walborsky and J. M. Motes, J. Am. Chem. Soc., 1970, 92, 2445; CrossRef H. M. Walborsky, A. A. Youssef, and J. M. Motes, J. Am. Chem. Soc., 1962, 84, 2465; CrossRef H. M. Walborsky and F. M. Hornyak, J. Am. Chem. Soc., 1955, 77, 6026. CrossRef
30. D. Hoppe, Angew. Chem., Int. Ed. Engl., 1984, 23, 932. CrossRef
31. M. Sasaki, E. Kawanishi, Y. Shirakawa, M. Kawahata, H. Masu, K. Yamaguchi, and K. Takeda, Eur. J. Org. Chem., 2008, 3061. CrossRef
32. M. Sasaki, Y. Shirakawa, M. Kawahata, K. Yamaguchi, and K. Takeda, Chem. Eur. J., 2009, 15, 3363. CrossRef