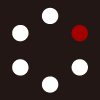
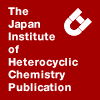
HETEROCYCLES
An International Journal for Reviews and Communications in Heterocyclic ChemistryWeb Edition ISSN: 1881-0942
Published online by The Japan Institute of Heterocyclic Chemistry
e-Journal
Full Text HTML
Received, 10th December, 2009, Accepted, 28th January, 2010, Published online, 29th January, 2010.
DOI: 10.3987/REV-09-664
■ Development of Novel Asymmetric Reactions and Their Application to the Synthesis of Natural Products
Manabu Node,* Tetsuya Kajimoto, and Minoru Ozeki
Kyoto Pharmaceutical University, Misasagi, Yamashina-ku, Kyoto 607-8412, Japan
Abstract
Asymmetric Michael addition of zinc enolate to a chiral nitroolefin was improved and the method was applied to the synthesis of (–)-Δ9(12)-Capnellene [(–)-6], (–)-aphanorphine [(–)-7], and (–)-eptazocine [(–)-8]. Whisky lactone (28) was also prepared by taking advantage of tandem Michael addition-MPV-reduction, which was capable of constructing three contiguous chiral centers of 1,3-mercaptoalcohols. In addition, (+)-negamycin [(+)-35] was synthesized by using the Michael addition of a chiral amine [(–)-31] as a key step with the best overall yield and with shortest steps to date. Efficient synthesis of galanthamine [(-)-51] was attained by a novel strategy of remote asymmetric control of intramolecular Michael addition of phenolic hydroxyl group to the dienone moiety. Moreover, epibatidine [(–)-42] was synthesized by using the Diels-Alder reaction, of which the dienophile, chiral allene dicarboxylate, was prepared by asymmetric crystallization. Finally, the first synthesis of naturally occurring form of dichroanal B (71), dichroanone (72), and taiwaniaquinone H (73) were achieved by using intramolecular asymmetric Heck reaction.1. INTRODUCTION
A number of asymmetric reactions have been developed to date and applied to the total synthesis of chiral forms of naturally occurring products having biological activity. The first author of the present article initially became interested in using Michael addition to develop a novel asymmetric reaction in 1990, when he was appointed as a professor of Kyoto Pharmaceutical University (KPU). His research at KPU started with the improvement of the chiral nitroenamine 1, the Michael acceptor of the asymmetric nitroolefination developed by Fuji et al.1
2. IMPROVEMENT OF ASYMMETRIC NITROOLEFINATION USING A CHIRAL NITROENAMINE
The nitroolefination initiated with Michael addition of an enolate prepared from the six-membered lactone 2 and the nitroolefine 1a,1b proceeded in good yield with excellent ee.; however, reactions with the enolates of five-membered lactones afforded disappointing results. In the transition-state of the Michael addition, three molecules of the zinc enolate were supposed to coordinate to one molecule of 1a, 1b (Figure 1). The preference of the Re-face attack of the enolate (Figure 1, path A) afforded the products 4a and 4b, the ee values of which were decreased when the Si-face attack of the enolate (Figure 1, path B) was not effectively suppressed due to the relatively small steric hinderance between the ligand of the zinc ion and the methoxyl group in 1a and 1b. Thus, the methoxyl groups of 1a and 1b were substituted with a more bulky tert-butyldimethylsilyloxy group to overcome the defects of the Michael addition.
As a result, the reaction of the zinc enolate of 2-methyl-γ-lactone (5) with 3a produced 4a in 92% yield with 88%ee, while that with 1a provided 4a in 56%ee (Table 1, entries 1 and 2). Moreover, the reaction of the enolate of 2-methyl-δ-lactone (2) with 3b increased the chemical yield and ee, compared to the reaction with 1b (Table 1, entries 3 and 4).2,3
Taking advantage of the reaction, which used 3a and 3b as the chiral Michael acceptor to afford 4b and 4c in excellent chemical yield and ee (Table 1, entries 4 and 5), (–)-Δ9(12)-capnellene [(–)-6], (–)-aphanorphine [(–)-7], and (–)-eptazocine [(–)-8] were efficiently synthesized in short steps as shown in Scheme 1.4,5
3. TANDEM ASYMMETRIC MICHAEL ADDITION – MPV-REDUCTION
Asymmetric Michael addition using a chiral mercaptoalcohol such as (–)-(1R,3R,4R)-3-hydroxy-8-mercapto-p-menthane [(–)-9] as a nucleophile was first attempted, employing many kinds of α,β-unsaturated carbonyl compounds as the Micheal acceptors. The Michael addition of methyl vinyl ketone (10a) with (–)-9 in the presence of dimethylaluminum chloride afforded the MPV-reductant 11 as well as the Michael adduct 12 in 30% and 36% yield, respectively (Scheme 2, eq. 1). Since the equilibrium between acyclic ketone / cyclopentanol and secondary alcohol / cyclopentanone would incline to the right more than that of acyclic ketone / cyclohexanone and secondary alcohol / cyclohexanol, the Michael addition of 10a was performed with the mercaptoalcohol (+)-13 having cyclopetanol as a partial structure to afford the corresponding MPV-reductant 14a. As expected, the MPV-reductant 14a was obtained as a sole product in good yield (69%) (Scheme 2, eq. 2).6
The stereochemistry of the Michael addition and MPV-reduction was investigated using several α,β-unsaturated ketones, 10b-f, as the Michael acceptor. As shown in Table 2, the Michael addition-MPV-reduction of 10b afforded 14b as a single diastereomer, the absolute structure of which was determined by X-ray diffraction analysis after deriving to the sulfone.
A plausible mechanism leading to the high diastereoselectivity is shown in Scheme 3.7 Namely, the β-substituted α,β-unsaturated ketone 10b reacted reversibly with the chiral aluminum complex 15 derived from (+)-13 and Me2AlCl to give two diastereomeric chelated adducts, 16a and 16b. The R-diastereomer 16a was subsequently subjected to a reversible intramolecular MPV reduction. Since the distance between the migrating hydrogen and the carbonyl carbon in the chelated R-isomer 16a was estimated about 3.1–3.4 Å from PM3 calculations, the R-isomer of the 10-membered ring could easily proceed to the rigid bicyclic transition-state. Meanwhile, the phenyl ring in the S-diastereomer 16b lay close to the hydrogen at C-10 on the β-face of the 10-membered chelated ring, which greatly retarded the MPV-reduction. Thus, the production of a single isomer of 14b was attributed to dynamic kinetic resolution8 via reversible Michael addition and the kinetically controlled intramolecular MPV-reduction of one of the two Michael adducts (Scheme 3).
Interestingly, in the reaction using the chiral mercaptoalcohol 17 as a nucleophile, different facial selectivity from the reaction with (+)-13 was observed in the Michael addition step (Table 3).9
The inverted stereoselectivity of the products 18 in the tandem Michael addition-MPV reduction could be attributed to the plausible transition-state shown in Figure 2.
Moreover, when the α-substituted α,β-unsaturated ester 19 was employed as the Michael acceptor, the enolate intermediate generated by the Michael addition was protonated by the proton on the sulfur atom to afford 20 with high diastereoselectivity (Scheme 4).10
To expand the method for controlling three contiguous chiral centers, the reaction of α,β-disubstituted α,β-unsaturated ketone 21 with the chiral mercaptoalcohol (+)-13 was scrutinized further.11
The results are summarized in Table 4, and show that the substrates having aromatic rings for both R1 and R3 were converted to the β-mercaptoalcohol in good yield with excellent dr (Table 4, entries 1-4). In addition, as the alkyl group at the α-position R2 became more bulky, the yield became the less satisfactory; however, the dr increased (Table 4, entries 2-4). Unfortunately, the chemical yield and dr were both unsatisfactory when the substrates having an alkyl group for R3 were used as the Michael acceptor (Table 4, entries 5-7).
Thus, several additives were tested to increase the yield and dr of the reaction using the substrate 21f as a model Michael acceptor. A relationship between the pKa values of additives and chemical yield was clearly observed and adding pentafluorobenzoic acid (PFBA) (pKa = 1.7) was found to give the best yield (49%, 22Af:22f = 93:2) (Figure 3).11
Next, the most suitable amount of PFBA was scrutinized in the reaction using 21f as the acceptor substrate. The highest chemical yield of the product 22Af was obtained in the case that 1.5 eq. of PFBA was added against the substrate (Figure 4). Moreover, the additive increased the ratio of 22A : 22B, compared to the reaction without PFBA (Table 4 and 5).
To improve the usefulness of the tandem Michael addition-MPV reduction in organic synthesis, desulfurization of the chiral sulfide moiety from 14 to provide chiral secondary alcohols 24a and 24b was tried using Raney nickel, the most common reagent for the reduction of carbon-sulfur bonds. Unexpectedly, racemization of the secondary alcohol was observed especially in the case of benzylic alcohols due to the occurrence of a set of oxidation-reduction reactions. Thus, sodium hypophosphite was used as a hydrogen source in the reduction with Raney Ni (W-2) in ethanolic acetate buffer to suppress the oxidation. In addition, elimination of the bornyl sulfide moiety, followed by the oxidation of sulfide to sulfoxide, was also carried out to give chiral allylic alcohols 25 (Scheme 5). As an application of the desulfurization, rove beetle pheromone 26 was synthesized in short steps (Scheme 6).
Finally, removal of the chiral auxiliary used in the tandem Michael addition-MPV reduction was performed. Needless to mention, treating 18a-c with DBU facilely gave the corresponding syn-mercaptoalcohol in good yield; however, some effort had to be made to cleave the 10-camphonyl group from the products 22A. Herein, the Wagner-Meerwein rearrangement, which occurs in the treatment of bornyl-type monoterpenes with Lewis acids, was chosen to cleave the chiral group from the products. Namely, the carbonyl group of 22Ae was first reduced with lithium aluminum hydride to alcohol, which was then treated with BF3.Et2O followed by a thiol exchange reaction in the presence of BF3.Et2O and dodecanethiol to afford the desired 1,3-mercaptoalcohol 27a in good yield (Table 6).10-12
As an application of the tandem Michael addition-MPV reduction, whisky lactone (28a) and cognac lactone (28b) were synthesized.13 The carbon-sulfur bond in the products was reductively cleaved by Raney nickel after the protection of the hydroxyl group by conventional acetylation.14 The resulting acetate 29 was oxidized with ruthenium tetroxide, saponified, and treated with acid to afford 28a and 28b (Scheme 7).
4. ASYMMETRIC MICHAEL ADDITION OF A CHIRAL AMINE.
Asymmetric Michael additions of amines to α,β-unsaturated esters are very promising reactions to afford units of β-amino acids, which are a part of a number of naturally occurring bioactive compounds. Many methods of stereoselective Michael addition, with amines or amides utilized as nucleophiles, have been developed using either catalytic or stoichiometric approaches. For example, Davies employed a chiral phenethylamide as a nucleophile to attack α,β-unsaturated esters to afford derivatives of β-amino acids,15 while Tomioka achieved the Michael addition of an achiral N-silylated lithium amide in the presence of a chiral ligand.16 Enders developed a recyclable N-silylated amide carrying (S)-2-methoxymethylpyrrolidine as a nucleophile.17 On the basis of these findings, we next embarked on the development of a novel asymmetric Michael addition to α,β-unsaturated esters 30 using the chiral bornylamine (–)-31 as a nucleophile, which could be prepared from commercially available ketopinic acid,18 for establishing an alternative way of providing both (R)- and (S)-β-amino acids.19
Initially, the Michael additions were scrutinized by fixing the reaction conditions, i.e., adding the amide prepared from (–)-31 (1.5 eq) and n-butyllithium (1.5 eq) to a solution of tert-butyl cinnamate 30a in an appropriate organic solvent and stirring the mixture at -78 ºC for 2 hours (Table 7). While the reaction conducted in a non-polar solvent, such as toluene or hexane, did not proceed well in terms of chemical yield and diastereoselectivity (Table 7, entries 5 and 6), that in diethyl ether (Table 7, entry 1) and in tetrahydrofuran (Table 7, entry 4) proceeded with satisfactory yields and an excellent contrast of product ratios. Interestingly, the diastereoselectivity was completely inverted by changing the solvent from diethyl ether to tetrahydrofuran (Table 7, entries 1 and 4).
The reaction solution of tetrahydrofuran maintained a transparent red color during the reaction while that of diethyl ether remained transparent yellow. On the basis of this difference in color, the inversion of the diastereoslectivity could be attributed to differences in the aggregated forms of the lithium amide in the solutions, i.e., forms A and B would be dissociated by adding tert-butyl cinnamate 30a to construct the transition states TS-A and B (Scheme 8).
Moreover, it was found that the addition of 0.5 equivalents of lithium salts, such as lithium trifluoroacetate and lithium trifluoromethanesulfonate, generally increased both chemical yield and diastereoselectivity. Notably, in the reaction with p-chlorocinnamate (30b), the chemical yield increased from 80% to 91% and the diastereoselectivity improved from 91 : 9 to 94 : 6 on adding lithium triflate (Table 8).
The newly generated chiral center was determined to have an S-configuration by X-ray diffraction analysis of 32Ad, which was obtained in the reaction of tert-butyl p-methylcinnamate with (–)-31.
Next, in order to develop the Michael addition as a general method to synthesize β-amino acids or esters, the chiral auxiliary must be removed from the Michael adducts. Although cleavage reactions of the carbon-nitrogen bond are difficult in general, many methods have been developed. For example, the oxidative dealkylation of secondary amines with N-chlorosuccinimide (NCS) can be conducted facilely due to the ease of forming a Schiff base.20 Thus, we tried to cleave the chiral auxiliary by using an excess amount of N-iodosuccinimide (NIS) to oxidize the tertiary amine into iminium salt.21 Namely, the Michael adducts 32A were treated with 4 equivalents of NIS to afford the β-amino esters 33 and 2-methoxybornyl aldehyde 34, which can be reconverted to (–)-31 by reductive amination with benzyl amine, in good yield (Table 9).
The present method was used to synthesize (+)-negamycin [(+)-35],22,23 an antibiotic isolated from Streptomyces purperfuscus. The Michael addition of (–)-31 was applied to the α,β-unsaturated tert-butyl ester 36, which was prepared by the metathesis reaction of oxazolidine 37 and tert-butyl acrylate (38) in the presence of Grubbs catalyst.24 As expected, the amine was introduced in good yield with an excellent ee to afford 39. After the cleavage of the chiral auxiliary with NIS, the resulting amine 40 was converted to (+)-35 in 4 steps. The synthetic route starting from Boc-glycinal 41 provided (+)-35 with an overall yield of 42% in eight steps (Scheme 9).25
5. ASYMMETRIC CRYSTALLIZATION OF ALLENE-1,3-DICARBOXYLATE AND SYNTHESIS OF EPIBATIDINE
(–)-Epibatidine [(–)-42] is an alkaloid isolated from the skin of the poison frog Epipedobates tricolor,26 the collection of which is forbidden by the international treaty for the protection of endangered species enacted in 1984. Although much progress has been made over last decade in establishing practical methods of synthesis,27 optically pure (–)-42 remains an attractive target because it is an excellent candidate for a non-opioidic analgesic agent for clinical use in accordance with the development of biological studies.28 We succeeded in the asymmetric synthesis of (–)-42 by means of the crystallization-induced asymmetric transformation of allene-1,3-dicarboxylates (43-45) (Figure 5).29
A mixture of (S)- and (R)-isomers of di-(–)-menthyl allene-1,3-dicarboxylate (diastereo ratio = 5:4) (43) prepared from di-(–)-menthyl 1,3-acetonedicarboxylate by a reaction with 2-chloro-1,3- dimethylimidazolinium chloride (DMC) and triethylamine was crystallized in the presence of triethylamine (0.01 eq.) at low temperature to afford di-(–)-menthyl (R)-allene-1,3-dicarboxylate [(R)-43] as a single crystal in pentane. Repeating the same procedure three times gave (R)-43 in 90% total yield (Scheme 10).
Later, we found that (–)-bornyl and (–)-isobornyl allene-1,3-dicarboxylates (44, 45) were more suitable substrates for the asymmetric crystallization because the procedure was attainable in hexane at room temperature (44, 89%; 45, 89%). The absolute axis configuration of 44 and 45 obtained by the asymmetric crystallization was identical to that of (R)-43.30
Being encouraged by obtaining the chiral allene-1,3-dicarboxylates (R)-43, we adopted it as a dienophile of the Diels-Alder reaction with N-Boc-pyrrole 46. Fortunately, the Diels-Alder reaction conducted in the presence of AlCl3 at –78 ºC in CH2Cl2 afforded the endo-adduct 47 as the sole product in good yield.31 After hydrogenation of the isolated double bond of the endo adduct (–)-47, the diester 48 was ozonolyzed to the β-ketoester 49, which was converted to a known synthetic intermediate (–)-5028c of (–)-epibatidine by dealkoxycarbonylation and successive reprotection of the secondary amino group with (Boc)2O (Scheme 11).
6. SYNTHESIS OF GALLANTHAMINE
(–)-Galanthamine [(–)-51], an alkaloid isolated from Galanthus woronowii, referred to as Caucasian snow drop,32 and Lycoris radiate33 was developed as a medicine for Altzheimer’s disease based on its biological actions as an allosteric modulator of the nicotinic receptor to secrete acetylcholine as well as a competitive inhibitor of acetylcholine esterase.34
The synthesis of (±)-51, where intramolecular oxidative phenol coupling of norbelladine (52) was adopted to afford narwedine (53), was first achieved by Barton and Kirby.35 Thereafter, much effort was made to improve their method36 since it was accompanied by the successful preparation of an enantiomerically pure form of (–)-51 using the asymmetric crystallization of (–)-narwedine [(–)-53](Figure 6).36 However, the yield of the intramolecular phenol coupling reaction in each route still remains less than 50%, which hinders the overall yield from reaching a satisfactory level.37
While some excellent approaches to the synthesis of (±)-51 or (–)-51 using the intramolecular Heck reaction as a key step have been published,38 such routes require multiple steps and their overall yields remain unsatisfactory. Based on this background, we succeeded in the asymmetric synthesis of (–)-5139,40 by improving the biomimetic approach via the intramolecular phenol coupling reaction.
In advance of the asymmetric synthesis of (–)-51, a facile synthetic route for (±)-51 was established using intramolecular phenol coupling.40 The intramolecular phenol coupling of 52 was reported to yield a p–p’ coupling product rather than the desired p–o’ coupling product due to steric repulsion.35 To overcome these disadvantages in the synthesis of (±)-51, we referred to Koga’s procedure where the catechol moiety of 52 was replaced with a pyrogallol group because the symmetrical characteristic of the pyrogallol moiety in the precursor (54) made the p–o’ coupling afford the only possible coupling product.41 Improving the yield of the oxidative phenol coupling of 55 by employing phenyliodine(III) bis(trifluoroacetate) (PIFA) as the oxidative reagent, our scheme increased the overall yield of the total synthesis of (±)-galanthamine [(±)-51] (60% overall yield from the norbelladine derivative 55, 6 steps) in comparison with yields reported to date (Scheme 12).
Being encouraged by the success in the racemic synthesis of (±)-51, we next planned to establish a novel route for the asymmetric synthesis of (–)-51 that could circumvent narwedine (53) in view of the severe allergic responses caused. In order to improve racemic synthesis for asymmetric synthesis, the phenolic hydroxyl group should preferentially attack one of the two β-olefinic carbons of the dienone in the intramolecular Michael addition since the symmetrical dienones (56) were converted to racemic mixtures (57) by a non-selective intramolecular reaction (Scheme 12).
Herein, remote axis asymmetric induction was used, restricting the conformation of the seven-membered ring of 56 by introducing chiral centers similar to Koga who put an alkoxycarbonyl group onto the seven-membered ring for chiral synthesis of (+)-galanthamine [(+)-51].41 Our strategy was designed so as to introduce another C–N bond of the benzylic position of the gallyl amino moiety of 56 to take advantage of the easily cleavable properties of the benzylic C–N bond and the aza-acetal bond. We decided to link an optically pure α-amino acid on the benzylic C–N bond of 56 to afford a chiral imidazolidinone [A]. Specifically, the conformation of the seven-membered ring of the coupling product [B] would be restricted by the fused-imidazolidinone composed of an amino acid to afford [C] as a single Michael adduct (Scheme 13). Originally, synthesis of chiral 1,3-imidazolidin-5-one and its application to chiral induction was reported by Seebach, who proposed the concept as Self Regeneration of Stereocenters (SRS).46 Among naturally abundant amino acids, phenylalanine and valine were chosen as the chiral auxiliary to form the fused imidazolidinone because the bulky isopropyl and benzyl groups on amino acid residues would provide promising stereoselectivities. In the beginning, we simply expected the spontaneous Michael addition after deprotection of R1 in [B] to progress diastereoselectively to afford cyclic ether [C] with the desired configuration.
As expected, PM3 calculations revealed that the most stable conformer of B (R1 = H, R2 = Bn, R3 = CF3CO), where the chiral imidazolodinone moiety was composed of D-phenylalanine, had a shorter distance between the phenolic oxygen atom and Cβ1 (2.61 Å) than that between the oxygen and Cβ2 (3.15 Å) (Figure 7).
Moreover, the calculation showed that the Michael adduct from the former transition state was more stable than that from the latter transition state by 6.7 kcal/mol (Figure 8). These results supported our expectation that the Michael addition of phenolic hydroxyl groups in B bearing a D-amino acid as a chiral auxiliary, predominantly affords a diastereomer that could lead to the desired enantiomers of synthetic intermediates.
This approach began with the reaction of tyramine and N-Boc-D-phenylalanine (58) in the presence of EDC.HCl and HOBt to afford amides 59. Removal of the Boc-group of 59 with methanesulfonic acid to afford amines 60, the formation of a Schiff base with 3,5-dibenzyloxy-4-methoxybenzaldehyde, and successive treatment with acid yielded imidazolidinone (61). The diastereoselectivity in the reaction providing 61 (>98% de) was satisfactorily high.46 The amino group of 61 was next protected with a trifluoroacetyl group to prepare 62, a substrate of phenol coupling. The intramolecular oxidative coupling of 62 with PIFA in trifluoroethanol at –40 ºC afforded the highest yield of the corresponding spirodienone 63 (Scheme 14).
Thus, deprotection of the benzyl group of 63 with boron trichloride at –78 ˚C afforded the desired 64 as a single diastereomer in excellent yield (95%), as expected.43 In the transformation of 64 into (–)-51, we deoxygenated the phenolic hydroxyl group of 64 via the triflate 65 prior to the reduction of α,β-unsaturated ketone, i.e., the cyclic ether 64 was converted to 65 which was reduced with a palladium catalyst in the presence of formate to afford the narwedine derivative 6644 and then, the conjugated ketone moiety of 66 was reduced with L-Selectride45 resulting in the allyl alcohol 67. The hydrolysis of 67 in an aqueous medium did not yield any imine 68 because of the low solubility of the deoxygenated intermediate 67. Therefore, more drastic conditions were chosen to achieve hydrolysis and the hydrolysis with potassium hydroxide in aqueous ethanol under refluxed conditions in the presence of a phase transfer, tetrabutylammonium bromide (TBAB), resulted in the imine 68. Optically pure (–)-galanthamine [(–)-51] (100% ee) was finally obtained by the reduction of 68 with sodium borohydride, followed by N-formylation using ethyl formate and the subsequent reduction of the formamide 69 with lithium aluminum hydride39 (Scheme 15).
7. ASYMMETRIC HECK REACTION AND SYNTHESIS OF ABEO-ABIETANE-TYPE DITERPENOIDS
Recently, diterpenes having an abeo-abietane (4a-methyltetrahydrofluorene) skeleton were identified as a new type of naturally occurring product. Among them, standishinal (70) isolated from Thuja standishii by Tanaka has been evaluated as a potential anti-tumor agent for treating breast cancer post-menopause because of its appreciable inhibitory activity against aromatase.47 Thus, other diterpenes possessing the same skeleton are expected to have promising activities for the treatment of female hormone-dependent cancers (Figure 9). In spite of the success in the total synthesis of racemic forms of dichroanal B (71), dichroanone (72), taiwaniaquinol B, and taiwaniaquinone D and H (73),48 no asymmetric synthesis has been reported to date, except for that by Stoltz and his colleagues.49 However, it should be noted that (+)-72 synthesized therein was the antipode of the naturally occurring compound. Against this background, we embarked on a synthetic study of natural form of abeo-abietane-type diterpenes using an intramolecular Heck reaction.
We started with the synthesis of (±)-72 in advance of the asymmetric synthesis of the abeo-abietane-type diterpenoids.50 The o-dienylphenyl triflate 74 was designed as the substrate of the intramolecular Heck reaction, and was prepared from β-cyclocitral (75) and 2’,3’-dihydroxy-4’- methoxyacetophenone (76) (Scheme 16). The palladium-catalyzed reaction proceeded smoothly in DMF at 100 °C to afford the isomers 77a and 77b, the isolated double bond of which was selectively reduced with Wilkinson’s catalyst providing the desired intermediate 78. Subsequent dealkylation and formylation yielded (±)-72 in short steps (Scheme 16).
In order to apply the synthetic scheme to the asymmetric synthesis of abeo-abietanes, the asymmetric intramolecular Heck reaction of 79 (a mixture of E/Z isomers) was conducted as a model experiment under Shibasaki’s condition where (S)-BINAP and potassium carbonate were adopted as a chiral ligand and base, respectively.51 Fortunately, the cyclized products 80 and 81 were attained in 70% yield with 49% ee at 60 ºC (Table 10, entry 1). Changing the solvent from toluene to aprotic polar solvents shortened the reaction period and increased both chemical and asymmetric yields at the same temperature (Table 10). The reaction in DMF gave the most satisfactory result, i.e., 90% yield with 97% ee (Table 10, entry 4). Since the dissociation of palladium and triflate could accelerate the Heck reaction using DMF as a solvent, the high enatioselectivity could be obtained by a route involving a cation intermediate.52
Since the two atropisomers were observed at lower temperature than -70 ℃ in the 1H-NMR spectroscopic analysis of the compound, in which the triflate group of 79 was replaced with N-Boc-L-phenylalanine, the present asymmetric Heck reaction should proceed under dynamic kinetic resolution via the equilibrium of two atropisomers (Scheme 17).53
Finally, we applied the intramolecular Heck reaction to the asymmetric synthesis of (–)-71 and (–)-72 isolated from roots of Salvia dichroantha,54 and (–)-73 from Taiwania cryptomerioides,55 Initially, we designed the substrate 82 bearing a rigid acetonide group in the catechol moiety because the flexible isopropyl group employed in the racemic synthesis of (±)-72 retarded the access of the palladium complex to a bulky chiral ligand. Thus, 82 was prepared by modifying a previously reported method 50 as shown in Scheme 18. Namely, 76 was treated with acetone in the presence of BF3.Et2O to give the acetonide 83, the bromination of which with NBS afforded 84 in excellent yield. After lithiation of the bromide 84 with n-butyllithium, the aryllithium generated was reacted with β-cyclocitral (75) to afford the benzylic alcohol 85. Dehydration of 85 by treatment with methanolic hydrochloric acid gave the diene 86 as a mixture of E and Z isomers (ca 1:4). After the demethylation of the methyl ether in 86 with dodecane thiol (Dod-SH) and sodium hydride in DMF, the phenolic compound 87 was converted to the triflate 82.
The intramolecular asymmetric Heck reaction of the triflate 82 (a mixture of E/Z isomers) was tried using palladium (II) acetate, chiral BINAP, and cesium carbonate in DMF at 100 °C, the hydrogenated product of which showed 76% ee (Table 11, entry 1). A remarkable temperature-dependence to improve the ee was not observed in the Heck reaction with chiral BINAP (Table 11, entries 1-3). Among several other chiral phosphorous ligands having C2-symmetry, Synphos (88) exhibited a further improvement in ee and reaction time (Table 11, entries 4-6). Moreover, temperature dependence was observed in the reaction. Finally, it was found that the Heck reaction of 82 and successive hydrogenation gave 89 in good yield (<86% in 2 steps) with excellent ee (94-98% ee) when (R)-88 was used as a ligand.
The absolute configuration of (+)-89 was confirmed to be S by a single-crystal X-ray diffraction analysis after deriving to 90 with NBS. Because the E-isomer of 82 also afforded the desired product in 46% yield under the Heck conditions, which meant the existence of equilibrium between the E/Z isomers, the E/Z mixture was used without any separation in the reactions.
Finally, the acetonide of (+)-89 (94.2% ee) was subjected to deprotection with HCl-MeOH followed by the Friedel-Crafts type reaction with dichloromethoxymethane in the presence of BCl3 to give (–)-71 in 92% yield. Treatment of (+)-90 (98% ee) with sodium methoxide in the presence of CuI followed by removal of the acetonide and oxidation with DDQ afforded (–)-72, which was further transformed to (–)-73 on treatment with Meerwein reagent (Scheme 19).56
We succeeded in the first asymmetric synthesis of naturally occurring abeo-abietane type diterpenoids, (–)-71, (–)-72 and (–)-73, in 10, 12, and 13 steps with an overall yield of 50, 40, and 39%, respectively. Our routes gave a much higher overall yield and ee with fewer steps than those for racemic and antipodal forms reported to date.
8. CONCLUSION AND ACKNOWLEDGEMENTS
In conclusion, we succeeded in the asymmetric synthesis of physologically and pharmaceutically important compounds by developing novel asymmetric reactions, i.e., tandem Michael addition-MPV reduction, Michael addition of the chiral amine, intramolecular Heck reaction, and asymmetric crystallization of allene 1,3-dicarboxylates. We thank the many coworkers and students involved in these studies. In addition, we acknowledge the financial supports from Grant-in-aid, the Frontier Research Program, and the 21st COE Program from the Ministry of Education, Culture, Sports and Technology, Japan.
References
1. a) K. Fuji, M. Node, H. Nagasawa, Y. Naniwa, and S. Terada, J. Am. Chem. Soc., 1986, 108, 3855; CrossRef b) K. Fuji, M. Node, H. Nagasawa, Y. Naniwa, T. Taga, K. Machida, and G. Snatzke, J. Am. Chem. Soc., 1989, 111, 7921. CrossRef
2. M. Node, R. Kurosaki, K. Hosomi, T. Inoue, K. Nishide, T. Ohmori, and K. Fuji, Tetrahedron Lett., 1995, 36, 99. CrossRef
3. K. Nishide, R. Kurosaki, K. Hosomi, H. Imazato, T. Inoue, M. Node, T. Ohmori, and K. Fuji, Tetrahedron, 1995, 51, 10857. CrossRef
4. a) W. Rui, K. Fuji, and M. Node, Huaxue Tongbao, 1992, 2, 29; b) M. Node, H. Imazato, R. Kurosaki, K. Kawano, T. Inoue, K. Nishide, and K. Fuji, Heterocycles, 1996, 42, 811. CrossRef
5. M. Node. K. Nishide, H. Imazato, R. Kurosaki, T. Inoue, and T. Ikariya, Chem. Commun., 1996, 22, 2559. CrossRef
6. K. Nishide, Y. Shigeta, K. Obata, and M. Node, J. Am. Chem. Soc., 1996, 118, 13103. CrossRef
7. M. Node, K. Nishide, Y. Shigeta, and H. Shiraki, K. Obata, J. Am. Chem. Soc., 2000, 122, 1927. CrossRef
8. a) R. Noyori, M. Tokunaga, and M. Kitamura, Bull. Chem. Soc. Jpn., 1995, 68, 36; CrossRef b) R. S. Ward, Tetrahedron: Asymmetry, 1995, 6, 1475, and references therein. CrossRef
9. H. Shiraki, K. Nishide, and M. Node, Tetrahedron Lett., 2000, 41, 3437. CrossRef
10. K. Nishide, S. Ohsugi, H. Shiraki, H. Tamakita, and M. Node, Org. Lett., 2001, 3, 3121. CrossRef
11. K. Nishide, M. Ozeki, K. Kunishige, Y. Shigeta, P. K. Patra, Y. Hagimoto, and M. Node, Angew. Chem. Int. Ed., 2003, 42, 4515. CrossRef
12. M. Ozeki, K. Nishide, F. Teraoka, and M. Node, Tetrahedron: Asymmetry, 2004, 15, 895. CrossRef
13. M. Ozeki, D. Hashimoto, K. Nishide, T. Kajimoto, and M. Node, Tetrahedron: Asymmetry, 2005, 16, 1663. CrossRef
14. M. Node, K. Nishide, Y. Shigeta, K. Obata, H. Shiraki, and H. Kunishige, Tetrahedron, 1997, 38, 12883. CrossRef
15. a) S. G. Davis and O. Ichihara, Tetrahedron: Asymmetry, 1991, 2, 183; CrossRef b) S. G. Davis, O. Ichihara, and I. A. S. Walters, Synlett, 1993, 461; CrossRef c) M. E. Bunnage, S. G. Davis, and C. J. Goodwin, Synlett, 1993, 731; CrossRef d) S. G. Davis, N. M. Garrido, O. Ichihara, and I. A. S. Walters, J. Chem. Soc., Chem. Commun., 1993, 1153; CrossRef e) S. G. Davis, O. Ichihara, and I. A. S. Walters, J. Chem. Soc. Perkin Trans.1, 1994, 1141; CrossRef f) S. G. Davis, O. Ichihara, and I. A. S. Walters, Synlett, 1994, 117; CrossRef g) S. G. Davis and D. R. Fenwick, J. Chem. Soc., Chem. Commun., 1995, 1109; CrossRef h) S. G. Davis and O. Ichihara, Tetrahedron: Asymmetry, 1996, 7, 1919. CrossRef
16. a) H. Doi, T. Sakai, M. Iguchi, K. Yamada, and K. Tomioka, J. Am. Chem. Soc., 2003, 125, 2886; CrossRef b) H. Doi, T. Sakai, K. Yamada, and K. Tomioka, J. Chem. Soc., Chem. Commun., 2004, 1850; c) T. Sakai, H. Doi, Y. Kawamoto, K. Yamada, and K. Tomioka, Tetrahedron Lett., 2004, 45, 9261. CrossRef
17. D. Enders, H. Wahl, and W. Bettray, Angew., Chem. Int. Ed. Engl., 1995, 34, 455. CrossRef
18. Org. Synth., Coll., Vol. 5, 689.
19. M. Node, D. Hashimoto, T. Katoh, S. Ochi, T. Watanabe, and T. Kajimoto, Org. Lett., 2008, 10, 2653. CrossRef
20. T. Sakai, H. Doi, and K. Tomioka, Tetrahedron, 2006, 62, 8351. CrossRef
21. T. Katoh, T. Watanabe, M. Nishitani, D. Hashimoto, M. Ozeki, T. Kajimoto, and M. Node, Tetrahedron Lett., 2008, 49, 598. CrossRef
22. S. Mizuno, K. Nitta, and H. Umezawa, J. Antibiot., 1970, 23, 581. CrossRef
23. M. Arakawa, M. Shiozuka, Y. Nakayama, T. Hara, M. Hamada, S. Kondo, D. Ikeda, Y. Takahashi, R. Sawa, Y. Nonomura, K. Sheykholeslami, K. Kondo, K. Kaga, T. Kitamura, Y. Suzuki-Miyagoe, S. Takeda, and R. Matsuda, J. Biochem., 2003, 134, 751. CrossRef
24. a) Handbook of Metathesis; R. H. Grubbs, Ed.; Wiley-VCH: Weinheim, 2003; b) P. Schwab, M. B. France, J. W. Ziller, and R. H. Grubbs, Angew. Chem., Int. Ed. Engl., 1995, 34, 2039. CrossRef
25. a) Y. Hayashi, T. Regnier, S. Nishiguchi, M. O. Sydnes, D. Hashimoto, J. Hasegawa, T. Katoh, T. Kajimoto, M. Shiozuka, R. Matsuda, M. Node, and Y. Kiso, J. Chem. Soc., Chem. Commun., 2008, 2379; b) S. Nishiguchi, M. O. Sydnes, A. Taguchi, T. Regnier, T. Kajimoto, M. Node, Y. Yamasaki, F. Yakushiji, Y. Kiso, and Y. Hayashi, Tetrahedron, 2010, 66, 314. CrossRef
26. T. F. Spande, H. M. Garraffo, M. W. Edwards, H. J. C. Yeh, L. Pannel, and J. W. Daly, J. Am. Chem. Soc., 1992, 114, 3475. CrossRef
27. Asymmetric synthesis of (–)- and (+)-epibatidine, see, a) E. J. Corey, T.-P. Loh, S. A. Rao, D. C. Daley, and S. Sarshar, J. Org. Chem. Soc., 1993, 58, 5600; CrossRef b) S. R. Fletcher, R. Baker, M. S. Chambers, S. Hobbs, and P. J. Mitchell, J. Chem. Soc., Chem. Commun., 1993, 1216; CrossRef c) S. R. Fletcher, R. Baker, M. S. Chambers, R. H. Herbert, S. C. Hobbs, S. R. Thomas, H. M. Verrier, A. P. Watt, and R. G. Ball, J. Org. Chem., 1994, 3, 1771; CrossRef d) A. Hernández, M. Marcos, and H. Rapopport, J. Org. Chem., 1995, 60, 2683; CrossRef e) B. M. Trost and G. R. Cook, Tetrahedron Lett., 1996, 37, 7485; CrossRef f) C. Szántay, Z. Kardos-Balogh, I. Moldvai, C. Szántay, Jr., E. Temesvári-Major, and G. Blaskó, Tetrahedron, 1996, 52, 11053; CrossRef g) H. Kosugi, M. Abe, R. Hatsuda, H. Uda, and M. Kato, J. Chem. Soc., Chem. Commun., 1997, 1857; h) S. Aoyagi, R. Tanaka, M. Naruse, and C. Kibayashi, Tetrahedron Lett., 1998, 39, 4513; CrossRef i) D. C. Jones, N. S. Simpkins, and G. M. P. Giblin, Tetrahedron Lett., 1998, 39, 1023; CrossRef j) H. Nakashima, K. Hiroya, T. Taniguchi, and K. Ogasawara, Synlett, 1999, 1405; CrossRef k) A. Avenoza, C. Cativiela, M. A. Fernández- Recio, and J. M. Peregrina, Tetrahedron: Asymmetry, 1999, 10, 3999; CrossRef l) I. Cabanal-Duvillard, J.-F. Berrien, and J. Royer, Tetrahedron: Asymmetry, 2000, 11, 2525; CrossRef m) I. Cabanal-Duvillard, J.-F. Berrien, H. P. Husson, and J. Royer, Tetrahedron, 2000, 56, 3763; CrossRef n) A. Hall, P. D. Bailey, D. C. Rees, G. M. Rosair, and R. H. Wightman, J. Chem. Soc., Perkin Trans 1, 2000, 329; CrossRef o) D. A. Evans, K. A. Scheidt, and C. W. Downey, Org. Lett., 2001, 3, 3009; CrossRef p) Z.-L. Wei, C. George, and A. P. Kozikowski, Tetrahedron Lett., 2003, 44, 3847; CrossRef q) J.-C. Dong, X. Wang, R.-T. Li, H.-M. Zhang, T.-M. Cheng, and C.-L. Li, Bioorg. Med. Chem. Lett., 2003, 13, 4327; CrossRef r) Y. Hoashi, T. Yabuta, and Y. Takemoto, Tetrahedron Lett., 2004, 45, 9185; CrossRef s) C.-L. Ken and T.-P. Loh, Org. Lett., 2005, 7, 2965; CrossRef t) V. K. Aggarwal and B. Olofsson, Angew. Chem. Int. Ed., 2005, 44, 5516. CrossRef
28. For example, a) N. E. Heard and J. Turner, J. Org. Chem., 1995, 60, 4302; CrossRef b) J. W. Daly, J. Med. Chem., 2003, 46, 445; CrossRef c) M. I. Damaj, K. R. Creasy, A. D. Grove, J. A. Rosecrans, and B. R. Martin, Brain Res., 1994, 664, 34. CrossRef
29. a) M. Node, K. Nishide, T. Fujiwara, and S. Ichihashi, J. Chem. Soc., Chem. Commun., 1998, 2363; b) H. Kimura, T. Fujisawa, T. Katoh, K. Nishide, T. Kajimoto, and M. Node, Chem. Pharm. Bull., 2006, 54, 399. CrossRef
30. T. Katoh, C. Noguchi, H. Kimura, T. Fujiwara, S. Ichihashi, K. Nishide, T. Kajimoto, and M. Node, Tetrahedron: Asymmetry, 2006, 17, 2943. CrossRef
31. K. Nishide, S. Ichihashi, H. Kimura, T. Katoh, and M. Node, Tetrahedron Lett., 2001, 42, 9237. CrossRef
32. a) S. Uyeo and S. Kobayashi, Pharm. Bull., 1953, 1, 139; CrossRef b) S. Kobayashi, T. Shingu, and S. Uyeo, Chem. Ind., 1956, 177.
33. N. F. Proskurnia and A. P. Yakovleva, Zhur. Obschchei. Khim., 1952, 22, 1899.
34. For review: e.g., J. Marco-Contelles, M. do C. Carreiras, C. Rodriguez, M. Villarroya, and A. G. Garcia, Chem. Rev., 2006, 106, 116. CrossRef
35. D. H. R. Barton and G. W. Kirby, Proc. Chem. Soc., 1960, 392.
36. D. H. R. Barton and G. W. Kirby, J. Chem. Soc., 1962, 806. CrossRef
37. a) T. Kametani, K. Yamaki, H. Yagi, and K. Fukumoto, J. Chem. Soc. (C), 1969, 2602; CrossRef b) T. Kametani, C. Seino, K. Yamaki, S. Shibuya, K. Fukumoto, K. Kigasawa, F. Satoh, M. Hiiragi, and T. Hayasaka, J. Chem. Soc. (C), 1971, 1043; CrossRef c) T. Kametani, K. Shishido, E. Hayashi, C. Seino, T. Kohno, S. Shibuya, and K. Fukumoto, J. Org. Chem., 1971, 36, 1295; CrossRef d) T. Kametani, K. Yamaki, T. Terui, S. Shibuya, and K. Fukumoto, J. Chem. Soc., Perkin Trans 1, 1972, 1513; CrossRef e) T. Kametani, M. S. Premila, and K. Fukumoto, Heterocycles, 1976, 4, 1111. CrossRef
38. a) C. Pilger, B. Westermann, B. Flörke, and G. Fels, Synlett, 2000, 1163; CrossRef b) P. J. Parsons, M. D. Charles, D. M. Harvey, L. R. Sumoreeah, A. Shell, G. Spoors, A. L. Gill, and S. Smith, Tetrahedron Lett., 2001, 42, 2209; CrossRef c) B. M. Trost and F. D. Toste, J. Am. Chem. Soc., 2000, 122, 11262; CrossRef ; CrossRef e) B. M. Trost, W. Tang, and F. D. Toste, J. Am. Chem. Soc., 2005, 127, 14785; CrossRef f) C. Guillou, J.-L. Beunard, E. Gras, and C. Thal, Angew. Chem., Int. Ed., 2001, 40, 4745; CrossRef g) A. Mary, D. Z. Renko, C. Guillou, and C. Thal, Tetrahedron Lett., 1997, 38, 5151; CrossRef h) A. Mary, Z. D. Renko, C. Guilou, and C. Thal, Bioorg. Med. Chem., 1998, 6, 1835; CrossRef i) C. Guiloou, A. Mary, Z. D. Renko, E. Gras, and C. Thal, Bioorg. Med. Chem. Lett., 2000, 10, 637; CrossRef j) D. Herlem, M. Martin, C. Thal, and C. Guillou, Bioorg. Med. Chem. Lett., 2003, 13, 2389; CrossRef k) R. C. Larock and D. E. Stinn, Tetrahedron Lett., 1988, 29, 4687. CrossRef
39. a) S. Kodama, Y. Hamashima, K. Nishide, and M. Node, Angew. Chem. Int. Ed., 2004, 43, 2659; CrossRef b) M. Node, S. Kodama, Y. Hamashima, T. Katoh, K. Nishide, and T. Kajimoto, Chem. Pharm. Bull., 2006, 54, 1662. CrossRef
40. M. Node, S. Kodama, Y. Hamashima, T. Baba, N. Hamamichi, and K. Nishide, Angew. Chem. Int. Ed., 2001, 40, 3060. CrossRef
41. a) K. Shimizu, K. Tomioka, S. Yamada, and K. Koga, Heterocycles, 1977, 8, 277; CrossRef b) K. Shimizu, K. Tomioka, S. Yamada, and K. Koga, Chem. Pharm. Bull., 1978, 26, 3765. CrossRef
42. a) Y. Kita, H. Tohma, K. Kikuchi, M. Inagaki, and T. Yakura, J. Org. Chem., 1991, 56, 435; CrossRef b) Y. Kita, M. Gyoten, M. Ohtsubo, H. Tohma, and T. Takada, Chem. Commun., 1996, 1481; CrossRef c) Y. Kita, T. Takada, M. Gyoten, H. Tohma, M. H. Zenk, and J. Eichhorn, J. Org. Chem., 1996, 61, 5857; CrossRef d) Y. Kita, M. Arisawa, M. Gyoten, M. Nakajima, R. Hamada, H. Tohma, and T. Takada, J. Org. Chem., 1998, 63, 6625; CrossRef e) Y. Kita, M. Egi, T. Takada, and H. Tohma, Synthesis, 1999, 885; CrossRef f) H. Tohma and Y. Kita, Topics Current Chem., 2003, 224, 209. CrossRef
43. a) S. Allen, T. G. Bonner, E. J. Bourne, and N. M. Saville, Chem. Ind., 1958, 630; b) A. B. Foster, D. Horton, N. Salim, M. Stacey, and J. Webber, J. Chem. Soc., 1960, 2587; CrossRef c) T. G. Bonner, E. J. Bourne, and S. McNally, J. Chem. Soc., 1960, 2929; CrossRef d) P. R. Brooks, M. C. Wirtz, M. G. Vetelino, D. M. Rescek, G. F. Woodworth, B. P. Morgan, and J. W. Coe, J. Org. Chem., 1999, 64, 9719. CrossRef
44. S. Cacchi, P. G. Ciattini, E. Morera, and G. Ortar, Tetrahedron Lett., 1986, 27, 5541. CrossRef
45. a) J. Szewczyk, A. H. Lewin, and F. I. Carroll, J. Heterocyclic Chem., 1988, 25, 1809; CrossRef b) J. Szewczyk, J. W. Wilson, A. H. Lewin, and F. I. Carroll, J. Heterocyclic Chem., 1995, 32, 195. CrossRef
46. a) D. Seebach, J. D. Aebi, R. Naef, and T. Weber, Helv. Chim. Acta, 1985, 68, 144; CrossRef b) R. Naef and D. Seebach, Helv. Chim. Acta, 1985, 68, 135; CrossRef c) D. Seebach, A. R. Sting, and M. Hoffmann, Angew. Chem., Int. Ed. Engl., 1996, 35, 2708. CrossRef
47. a) H. Ohtsu, M. Iwamoto, H. Ohishi, S. Matsunaga, and R. Tanaka, Tetrahedron Lett., 1999, 40, 6419; CrossRef b) T. Minami, M. Iwamoto, H. Ohtsu, H. Ohishi, R. Tanaka, and A. Yoshitake, Planta Med., 2002, 68, 742; CrossRef c) M. Iwamoto, H. Ohtsu, H. Tokuda, H. Nishino, S. Matsunaga, and R. Tanaka, Bioorg. Med. Chem., 2001, 9, 1911; CrossRef d) T. Katoh, T. Akagi, C. Noguchi, T. Kajimoto, M. Node, R. Tanaka, M. Nishizawa, H. Ohtsu, N. Suzuki, and K. Saito, Bioorg. Med. Chem., 2007, 15, 2736. CrossRef
48. a) M. Banerjee, R. Mukhopadhyay, B. Achari, and A. K. Banerjee, Org. Lett., 2003, 5, 3931; CrossRef b) M. Banerjee, R. Mukhopadhyay, B. Achari, and A. K. Banerjee, J. Org. Chem., 2006, 71, 2787; CrossRef c) E. Fillion and D. Fishlock, J. Am. Chem. Soc., 2005, 127, 13144; CrossRef d) G. Liang, Y. Xu, I. B. Speiple, and D. Trauner, J. Am. Chem. Soc., 2006, 128, 11022; CrossRef e) S. Tang, Y. Xu, J. He, Y. He, J. Zheng, X. Pan, and X. She, Org. Lett., 2008, 10, 1855; CrossRef f) L. Planas, M. Mogi, H. Takita, T. Kajimoto, and M. Node, J. Org. Chem., 2006, 71, 2896; CrossRef g) E. Alvarez-Manzaneda, R. Chahboun, E. Cabrera, E. Alvarez, R. Alvarez-Manzaneda, R. Meneses, H. Es-Samti, and A. Fernández, J. Org. Chem., 2009, 74, 3384. CrossRef
49. R. M. McFadden and B. M. Stoltz, J. Am. Chem. Soc., 2006, 128, 7738. CrossRef
50. L. Planas, M. Mogi, H. Takita, T. Kajimoto, and M. Node, J. Org. Chem., 2006, 71, 2896. CrossRef
51. T. Takemoto, M. Sodeoka, H. Sasai, and M. Shibasaki, J. Am. Chem. Soc., 1993, 115, 8477. CrossRef
52. J. M. Brown and K. K. Hii, Angew. Chem., Int. Ed. Engl., 1996, 35, 657. CrossRef
53. A manuscript on the details of the existence of atropisomers is now in preparation for publishing.
54. K. Kawazoe, M. Yamamoto, Y. Takaishi, G. Honda, T. Fujita, E. Sezik, and E. Yesilada, Phytochemistry, 1999, 50, 493. CrossRef
55. C.-I. Chang, J.-Y. Chang, C.-C. Kuo, W. Pan, and Y.-H. Kuo, Planta Med., 2005, 71, 72. CrossRef
56. M. Node, M. Ozeki, L. Planas, M. Nakano, H. Takita, D. Mori, S. Tamatani, and T. Kajimoto, J. Org. Chem., 2010, 75, 190. CrossRef