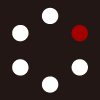
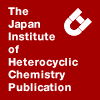
HETEROCYCLES
An International Journal for Reviews and Communications in Heterocyclic ChemistryWeb Edition ISSN: 1881-0942
Published online by The Japan Institute of Heterocyclic Chemistry
e-Journal
Full Text HTML
Received, 28th December, 2009, Accepted, 19th February, 2010, Published online, 23rd February, 2010.
DOI: 10.3987/REV-09-665
■ Recent Progress of New Catalytic Synthetic Methods for Nitrogen Heterocycles Based on Hydrogen Transfer Reactions
Ryohei Yamaguchi,* Ken-ichi Fujita, and Mingwen Zhu
Graduate School of Human and Environmental Studies, Kyoto University, Kyoto 606-8501, Japan
Abstract
This review summarizes a variety of synthetic methods for nitrogen heterocycles based on hydrogen transfer reactions catalyzed by transition metals in a past decade. Most of them employ iridium and ruthenium complexes as the catalysts and provide a versatile and environmentally benign synthetic methodology of various nitrogen heterocyclic compounds. Intermolecular cyclization reactions of amines with alcohols afford 5-7 membered alicyclic amines, quinolines, piperazines, indoles, quinoxalines, pyrroles, benzimidazoles, and benzoxazoles. Intramolecular cyclization reactions of amino alcohols give indoles, 1,2,3,4-tetrahydroqunolines, 1,2,3,4-tetrahydroquinoxalines, 3,4-dihydro-2(1H)-quinolinones, and oxindoles. Friedländer-type cyclization reactions provide a convenient route to various quinolines. Finally, cyclization reactions of amines with amines afford quinolines and phenylpyrrolidines.CONTENTS
1. INTRODUCTION
2. INTERMOLECULAR CYCLIZATION OF AMINES WITH ALCOHOLS
2.1 Ir-Catalyzed Reactions
2.2 Ru-Catalyzed Reactions
2.3 Other Reactions
3. INTRAMOLECULAR CYCLIZATION OF AMINO ALCOHOLS
4. FRIEDLÄNDER-TYPE CYCLIZATION FORMING QUINOLINES
4.1 Ru-Catalyzed Reactions
4.2 Other Reactions
5. CYCLIZATIONS OF AMINES WITH AMINES
6. REFERENCES
1. INTRODUCTION
Organic transformations based on catalytic hydrogen transfer reactions have attracted considerable attention in recent years from viewpoint of development of environmentally benign synthetic methodology, one of the most important subjects in current organic synthesis.1 Ru-catalyzed C-N bond formation reactions of amines with alcohols were initially investigated in 1980's, though most of them required high reaction temperature (>150 oC) and applicable substrates were rather limited.2 We found the high catalytic performance of Cp*Ir (Cp*: η5-pentamethylcyclopentadienyl) complexes in Oppenauer-type oxidation of alcohols in 2002.3 Since then, we have developed several environmentally benign C-N bond formation reactions using a Cp*Ir complex, [Cp*IrCl2]2 (1), as a hydrogen transfer catalyst.4-7 In these reactions, use of harmful organic halides as substrates can be avoided, and harmless and readily available alcohols are utilized as substrates. Moreover, only water is generated as the coproduct without producing stoichiometric amounts of wastes. A couple of examples are shown below.
(a) N-Alkylation of Amines with Alcohols4
Other than our works, there have been many reports on organic transformations based on catalytic hydrogen transfer reactions and several reviews have been appeared so far.1,2c,8 In this review, we would like to summarize our and other works on new catalytic synthetic methods for nitrogen heterocyclic compounds based on hydrogen transfer reactions over the last decade.
2. INTERMOLECULAR CYCLIZATION OF AMINES WITH ALCOHOLS
Intermolecular cyclization of amines with alcohols have proven to be attractive synthetic methods for nitrogen heterocycles. We have recently found versatile and efficient Cp*Ir-catalyzed cyclization systems and, thereafter, several Ir-catalyzed reactions have been reported.
2.1. Ir-Catalyzed Reactions
We reported a new method for the N-heterocyclization of primary amines with diols catalyzed by [Cp*IrCl2]2 (1) / NaHCO3 system.5a,b A variety of five-, six-, and seven-membered cyclic amines could be synthesized in good to excellent yields in environmentally benign and atom economical manner with only water as a coproduct. Table 2-1 summarizes the representative results of the reaction of primary amines with diols. In the presence of a catalytic amount of 1 (1.0 mol% Ir) and NaHCO3 (1.0 mol%), the reaction of benzylamine with 1,5-pentanediol (1.5 : 1.0 ratio) in toluene for 17 hours gave N-benzylpiperidine in an isolated yield of 91% (entry 1). The reactions of benzylamine with 1,4-butanediol and 1,6-hexanediol gave N-benzylpyrrolidine and N-benzylazepane in good yields, respectively (entries 2 and 3). The reactions of several substituted diols on the methylene chain also proceeded smoothly to give substituted cyclic amines (entries 4-8). In the reaction of benzylamine with 2,5-hexanediol, a diastereomeric mixture of N-benzyl-2,5-dimethylpyrrolidine (cis : trans = 73 : 27) was obtained in 94% yield (entry 4). Benzo-fused cyclic amines such as N-benzylisoindoline and N-benzyl-1,2,3,4-tetrahydroisoquinoline were also synthesized by the reactions of benzylamine with 1,2-benzenedimethanol and 2-(2-hydroxyethyl)benzyl alcohol, respectively (entries 9 and 10). The product having a morpholine skeleton could be synthesized in good yield (76%) by the employment of diethylene glycol as a diol substrate (entry 11). N-(3-Pyridylmethyl)pyrrolidine, which is known as a nicotinic agonist, could be smoothly synthesized using easily available 3-pyridylmethylamine as a starting material (entry 12). In the reactions of aniline as the starting primary amine, higher catalyst loading (5.0 mol% Ir) and a higher reaction temperature (130 °C) were required to obtain good yields (entries 14 and 15). The reaction of aniline with 1,4-butanediol gave N-phenylpyrrolidine in 70% yield (entry 14). Introduction of electron-donating substituents at the phenyl ring of aniline considerably improved the yield (entry 15). The reactions of other primary amines, such as 1-naphthylmethylamine, phenethylamine and octylamine, also afforded the cyclic amines in good yields (entries 13, 16, and 17).
The synthesis of N-benzylpiperidine in 100 mmol scale has been successfully accomplished by using a smaller amount of the iridium catalyst 1 (0.50 mol% Ir) as shown in Scheme 2-1.5c
A possible mechanism for the Cp*Ir complex-catalyzed N-heterocyclization of primary amines with diols is shown in Scheme 2-2,5a,b which is based on our previous studies on Cp*Ir-catalyzed N-alkylation of amines with alcohols.4,6 There should be two catalytic cycles 1 and 2. In the former, an intermolecular N-Alkylation of the primary amine with one of the alcohol moiety of diol would proceed to afford amino alcohol as an intermediate. Then, in the cycle 2, the amino alcohol intermediate would be cyclized intramolecularly to give the product via an iminium intermediate. The formation of iridium alkoxide species could be stimulated in the presence of the base by trapping hydrogen chloride generated at the first step of the reaction.
We also demonstrated an efficient two-step asymmetric synthesis of (S)-2-phenylpiperidine as an extension of the N-heterocyclization of primary amines with diols.5a,b The results are illustrated in Scheme 2-3. First, the reaction of enantiomerically pure (R)-1-phenylethylamine and 1-phenyl-1,5-pentanediol was conducted to produce a diastereomeric mixture of the corresponding N-(1-phenylethyl)-2-phenylpiperidines 2a and 2b with 92% de. Then hydrogenation of this diastereomeric mixture using Pd/C catalyst gave (S)-2-phenylpiperidine in 96% yield with 78% ee.
Trudell et al. reported a facile method for the efficient and enantioselective construction of 2-(pyridin-3-yl)piperidine alkaloids based on N-heterocyclization of enantiomerically pure primary amines with diols catalyzed by 1.9 As shown in Scheme 2-4, N-heterocyclization of (R)-1-phenylethylamine with diol 3 catalyzed by 1 gave a diastereomeric mixture of 2-substituted piperidines 4a and 4b in 72% yield with 90% de. Similarly, the reaction starting with (S)-1-phenylethylamine gave 5a and 5b in 69% yield with 90% de. Then, 4a and 5a were converted to 6 and 7, respectively, via hydrogenolysis of N-1-phenylethyl group and concomitant treatment with POCl3 (Scheme 2-5). Pd-catalyzed Suzuki-Miyaura coupling of 6 with 3-pyridineboronic acid gave (S)-noranabasamine in 84% with ee greater than 86%. (R)-noranabasamine were also prepared from 7 in 76% yield with ee greater than 80%.
We reported the facile synthesis of five- and six-membered cyclic secondary amines by N-heterocyclization of an ammonium tetrafluoroborate with diols, as a development of our studies on Cp*Ir-catalyzed N-alkylation of ammonium salts with alcohols.7a When the reaction of ammonium tetrafluoroborate with 1-phenyl-1,5-pentanediol was performed at 140 °C for 17 h in the presence of 1 (5.0 mol% Ir) and NaHCO3 (30 mol%), 2-phenylpiperidine was obtained in 85% yield (Scheme 2-6). Similarly, 2-phenylpyrrolidine was synthesized in 62% yield by the reaction of ammonium tetrafluoroborate with 1-phenyl-1,4-butanediol. By this method, synthetically important secondary N-heterocycles could be obtained by using cheap ammonium salt and diols as starting materials under solvent-free conditions.
Williams et al. reported the N-heterocyclization of tryptamine with diols catalyzed by [IrCl(cod)]2 / dppf [1,1'-bis(diphenylphosphino)ferrocene].10 The reaction of tryptamine with the appropriate diols (1,4-butanediol, 1,5-pentanediol, and 1,6-heptanediol) resulted in good conversion to the corresponding pyrrolidine, piperidine, and in reasonable isolated yield into azepane (Scheme 2-7).
Ishii et al. reported a unique system for N-heterocyclization of 1-naphthylamines with 1,2- and 1,3-diols catalyzed by IrCl3·3H2O / BINAP [2,2'-bis(diphenylphosphino)-1,1'-binaphthyl].11 Table 2-2 summarizes the representative results. When the reaction of 1-naphthylamine with 1,3-propanediol was performed in mesitylene under air at refluxing temperature for 15 h in the presence of IrCl3·3H2O (5.0 mol% Ir), BINAP (7.5 mol%), and Na2CO3 (8.0 mol%), 7,8-benzoquinoline was obtained in 96% yield (entry 1). A variety of benzoquinoline and benzoindole derivatives were synthesized by this system starting with naphthylamines and 1,2- and 1,3-diols (entries 2-8).
Madsen et al. reported the synthesis of piperazines by the reactions of 1,2-diamines with 1,2-diols catalyzed by 1 / NaHCO3 in aqueous media.12 The results are summarized in Table 2-3. For example, when the reaction of trans-1,2-diaminocyclohexane with ethylene glycol was performed in water at 100 °C overnight in the presence of 1 (1.0 mol% Ir) and NaHCO3 (5.0 mol%), decahydroquinoxaline was obtained in an isolated yield of 96% (entry 1). Various piperazine derivatives were also synthesized by this catalytic system (entries 2-5).
We also reported the synthesis of piperazines by the N-alkylative homo- and cross-coupling of ethanolamines catalyzed by 1 / NaHCO3.13 The representative results are summarized in Table 2-4 and Scheme 2-8. When the reaction of N-benzylethanolamine was performed in toluene at 110 °C for 17 h in the presence of 1 (5.0 mol% Ir) and NaHCO3 (15 mol%), N,N’-dibenzylpiperazine was obtained in 66% yield (entry 1). Methyl, methoxy, chloro, bromo, and trifluoromethyl substituents were tolerant in this catalytic system to give the corresponding piperazine derivatives in moderate to good yields (entries 2-7). Furthermore, cross-coupling reactions of Boc-protected diethanolamines with benzylamine were also carried out as shown in Scheme 2-8. These reactions gave N-benzyl-N'-Boc-piperazine derivatives in moderate to high yields.
A possible mechanism for the N-alkylative homocoupling of ethanolamines is described in Scheme 2-9. The first stage of the reaction would involve an intermolecular carbon-nitrogen bond formation through hydrogen transfer process and condensation to afford a diaminoethanol intermediate A. Subsequent intramolecular carbon-nitrogen bond formation would give the piperazine product.
2.2. Ru-Catalyzed Reactions14
Osakada and Yamamoto et al. reported that the reactions of 1,1’-ferrocenedimethanol with arylamine and with alkylamine in the presence of RuCl2(PPh3)3 catalyst afforded N-alkyl-(or N-aryl)-2-aza[3]ferrocenophanes (Table 2-5).15 Thus, the reaction of 1,1’-ferrocenedimethanol and 4-butylaniline was carried out in the presence of RuCl2(PPh3)3 catalyst (3.5 mol%) in NMP solution at 180 °C for 24h to give N-(4-butylphenyl)-2-aza[3]ferrocenophane in 63% yield (entry 1). Similar reactions with 4-t-butylaniline, 4-hydroxyaniline, hexylamine, and benzylamine afforded the corresponding N-substituted 2-aza[3]ferrocenophanes in moderate to good yields (entries 2-5).
Cho and Shim et al. reported Ru-catalyzed heteroannulation of anilines with alkanolammonium chlorides affording indoles.16 After several experiments to obtain the optimized reaction conditions, they found that the reactions of anilines with alkanolammonium chlorides in the presence of a catalytic amount of a ruthenium catalyst (5-10 mol%) together with SnCl2·2H2O (1 equiv.) in an aqueous medium (H2O–dioxane) at 180 oC for 20 h afforded the corresponding indoles in moderate to good yields. The representative results are summarized in Table 2-6. The employment of RuH2(PPh3)4 catalytic system was revealed to be more active towards indole formation than that of RuCl3·nH2O/ 3PPh3 catalytic system. The yield was considerably affected by the electronic nature and the position of the substituent on aniline. With chloroaniline having electron-withdrawing Cl substituent (entry 6), the yield was generally lower than those with anilines having electron-donating substituents (entries 2-5, 7 and 8). The yields of the reactions with ortho- and meta-substituted anilines were higher than those when para-substituted anilines were used (entries 2-8). In the reaction of m-toluidine, the corresponding indoles were obtained as a regioisomeric mixture, favoring 6-methyl isomer, which was formed via less sterically hindered position (entry 3).
In the cases of two-methyl substituted anilines (entry 9-11), the reactions proceeded smoothly even by the use of 5 mol% of ruthenium catalyst (RuCl3·nH2O/3PPh3) as compared to those with mono-substituted anilines. The formation of >100% yields of indoles indicates that at least two alkanol groups out of three in triethanolammonium chloride are available for the C2-fragment counterpart.
Although the present reaction mechanism including the role of SnCl2·2H2O is not yet fully understood, a plausible pathways are shown in Schemes 2-10 and 2-11. The initial formation of 2-anilinoethanols (A) by an alkanol group transfer from alkanolammonium chlorides to anilines seems to be a key step (Scheme 2-10). Subsequent steps seems to proceed via N-alkylation and ruthenium-mediated heteroannulation shown in Cycle A and Cycle B (Scheme 2-11).
Cho and Oh reported a new ruthenium-catalyzed approach for quinoxalines from o-phenylenediamines and vicinal-diols.17 The representative results are summarized in Table 2-7.
The addition of benzalacetone as a hydrogen acceptor at the present reaction resulted in a slightly increased yield, whereas that of 1-dodecene showed no significant change (entries 1-3). The presence of KOH was essential for the effective formation of quinoxalines, because the reactions in the absence of KOH resulted in only 13% and 18% yields (entries 4 and 5), consistent with the well-known fact that strong bases are used as promoters in transition metal-catalyzed transfer hydrogenation from alcohols. Various vicinal-diols were subjected to react with o-phenylenediamines in order to investigate the reaction scope (entries 6-20). The product yield was not significantly affected by the position of the substituent on the aromatic ring, whereas the electronic nature of that had some relevance to the product yield.
Williams et al. reported that the combination of a bidentate phosphine, such as dppf, with [Ru(p-cymene)Cl2]2 was particularly effective for N-alkylation of amines with alcohols.18 Cyclization of 1,5-pentanediol with aniline by using this catalytic system afforded N-phenylpiperidine in moderated yield (Scheme 2-12). However, a greater catalyst loading was needed to avoid formation of the lactone and other side products than the mono-alkylation.
Williams et al. also reported ruthenium-catalyzed conversion of 1,4-alkynediols into pyrroles.19 Xantphos as ligand gave the best reactivity and selectivity in furan formation and used this ligand for pyrrole-forming reactions. Thus, various 1,2,5-substituted pyrroles have been synthesized from 1,4-alkynediols using a ruthenium catalyzed isomerization to give the corresponding 1,4-dicarbonyl compounds, which undergo in situ cyclization to pyrroles in the presence of amine. The representative results are summarized in Table 2-8.
The reactions with unhindered substrates were highly selective for pyrrole formation, leading to the formation of 2,5-dimethylpyrroles (entries 1-4). More hindered substrates still afforded pyrroles selectively, although in some cases, the corresponding furans were formed as significant by-products (entries 5-7, 10, and 12). However, many functional groups were tolerated, including halide, nitrile, ester and furyl (entries 10-14). Aniline could be used as the amine (entries 15-17), although some diketone remained uncyclized under these conditions. 2-Aminopyridine gave an unsatisfactory conversion into pyrrole (entry 18). Formation of pyrroles possessing a 2-(p-chloro)-substituent was generally less selective towards pyrrole formation, although reasonable conversions were obtained in favorable cases, where unbranched aliphatic primary amines were used (entries 20 and 21).
Very recently, Williams et al. reported Ru-catalyzed N-heterocyclization of amines with diols.20 Given the success of amine alkylation with alcohols using the catalytic system consisted of [Ru(p-cymene)Cl2]2 with diphosphines, they disclosed that the use of 2.5 mol % [Ru(p-cymene)Cl2]2 with DPEphos was an effective catalyst. The use of triethylamine (10 mol %) was found to provide consistent results, and this was used as an additive in all cyclization reactions. The representative results are summarized in Table 2-9.
Aniline and other substituted anilines were reacted with 1,4-butanediol to give N-phenylpyrrolidines (entry 1-7), although the more electron poor anilines gave lower conversions under these conditions (entries 6 and 7). Aliphatic primary amines were also effective (entries 8-11), including the branched primary amine. The use of 1,5-pentanediol and 1,6-hexanediol also led to cyclization to the corresponding N-heterocycle (entries 12 and 13).
2.3. Other Reactions
Blacker, Marsden, and Williams et al. have recently reported Ru-catalyzed synthesis of benzimidazoles from alcohols and o-aminoaniline.21a The reactions were carried out using Ru(PPh3)3(CO)H2 (2.5 mol %) as a catalyst and Xantphos (2.5 mol %) as a ligand in the presence of crotononitrile (2.2 equiv.) as a hydrogen acceptor as well as piperidinium acetate (15 mol %) in toluene at reflux. The representative results are summarized in Table 2-10.
A range of alcohols was converted into the corresponding benzimidazoles. Benzylic alcohols having electron-rich (entries 3 and 4) and electron-deficient (entry 5) substituents were converted into benzimidazoles in good yield, although the 2-methylsubstituted benzyl alcohol gave a lower yield (entry 6). The 2-thienyl, 2-furyl and nonbenzylic substrates were also effective (entries 7-10). The reaction is not limited to simple o-aminoanilines, as is shown in the heterocyclization of 2,3-diaminophenazine giving the product in high yield (entry 11).
They also reported Ir-catalyzed synthesis of bezoxazoles from aldehydes and 2-aminophenoles without hydrogen acceptor.21a The reactions were conducted using [Cp*IrI2]2 (1 mol %) as a catalyst in toluene at reflux. When the reaction was carried out at the higher concentration of 5 M, higher yield were obtained. The reactions were equally effective in the presence or absence of styrene as a sacrificial hydrogen acceptor. The representative results are summarized in Table 2-11. Benzaldehyde (entry 1) and electron-rich benzaldehydes (entries 2 and 3) gave good yields, although the electron-deficient 4-cyanobenzaldehyde (entry 4) and aliphatic aldehyde (entry 7) only gave moderate yields, consistent with a mechanism involving a hydride abstraction with associated buildup of partial cation character. The reactions of heterocyclic aldehydes (entries 5 and 6) and substituted 2-aminophenols (entries 8-11) were successful in all cases. Enolizable aliphatic aldehydes were not suitable substrates.
Finally, a synthesis of benzothiazole was also successfully performed by the similar reaction conditions as above. Thus, the cyclization reaction of p-tolualdehyde and 2-aminothiophenol gave benzothiazole in a moderate yield (Scheme 2-13).
Very recently, Blacker, Marsden, and Williams et al. reported a new system for oxidative condensation of amines with 2-aminophenols catalyzed by [{η5-Ph4C4CO)}2HRu2(CO)4(µ-H)], so-called Shvo catalyst.21b
Various benzoxazole derivatives can be synthesized by this catalytic system using 2,6-dimethoxy-1,4-benzoquinone as the hydrogen-accepting terminal oxidant. The representative results are summarized in Table 2-12. The reaction is effective for a range of benzylic amines. Higher yields were obtained for substrates bearing electron-donating substituents and lower yields were obtained for the electron-poor derivative (entries 2-4). The presence of an electron-withdrawing chloro substituent in aminophenol gives lower yield than the corresponding electron-rich variants (entries 5 and 6). Additionally, the reaction also proceeded with non-benzylic amine to give the corresponding benzoxazole in moderate yield (entry 8).
3. INTRAMOLECULAR CYCLIZATION OF AMINO ALCOHOLS
The intramolecular cyclization of amino alcohols should be an attractive method for the synthesis of N-heterocyclic compounds, because they can be obtained in a single step reaction and without the generation of wasteful coproducts.22 Some publications describing the new catalytic system for N-heterocyclization of amino alcohols based on hydrogen transfer reactions have appeared recently.
We reported the cyclization of amino alcohols to benzo-fused N-heterocycles catalyzed by 1 / K2CO3 system.6 The representative results are summarized in Table 3-1. The reaction of 2-aminophenethyl alcohol under toluene reflux for 17 hours in the presence of 1 (5.0 mol% Ir) and K2CO3 (10 mol%) gave indole in 80% yield (entry 1). 2-Aminophenethyl alcohol derivatives bearing a substituent on the aromatic ring were converted into the corresponding indoles in moderate to high yields (entries 2-4). Indoles bearing a substituent on the N-heterocyclic ring could be also synthesized in good to excellent yields by the reaction of 2-aminophenethyl alcohols with a substituent on the methylene chain (entries 5 and 6).
Although the mechanism for this reaction is not completely clear as of yet, a possible mechanism is shown in Scheme 3-1. The first step of the reaction would involve catalytic oxidation of an alcohol to an aldehyde to give an intermediate A and a hydrido iridium species. The intermediate A would readily cyclize to afford indoles via intramolecular nucleophilic attack of amino group to carbonyl carbon followed by dehydration, which would be a noncatalytic process. Release of hydrogen in the reaction of the hydrido iridium with 2-aminophenethyl alcohol could regenerate the catalytic active alkoxo iridium species.23
We also reported the intramolecular cyclization of amino alcohols affording 1,2,3,4-tetrahydroquinoline derivatives.6 The results are summarized in Table 3-2. 3-(2-Aminophenyl)propanols bearing a substituent at the aromatic ring (entries 2-4) or the methylene chain (entries 5 and 6) were converted into the corresponding 1,2,3,4-tetrahydroqunolines in moderate to high yields. It should be noted that this catalytic system was applicable to the synthesis of 2,3,4,5-tetrahydro-1-benzazepine using 4-(2-aminophenyl)butanol as a starting material (entry 7).
A possible mechanism for the catalytic synthesis of 1,2,3,4-tetrahydroquinolines and 2,3,4,5-tetrahydro-1-benzazepine is shown in Scheme 3-2. The first step of the reaction affording an intermediate B would be similar to that for the synthesis of indoles shown above. The intermediate B would transform into C via intramolecular nucleophilic addition and dehydration. Then addition of the hydrido iridium to an iminic C=N bond of C would occur to give an amido iridium intermediate D. The intermediate D would react with an amino alcohol to give a product and regenerate the catalytic active alkoxo iridium species.
Eary et al. reported the cyclization of anilino alcohols to give 1,2,3,4-tetrahydroquinoxalines catalyzed by 1 / K2CO3 system. The results are summarized in Scheme 3-3.24 The reaction of 2-(2-aminophenylamino)ethanol at 110 °C for 17 hours in the presence of 1 (25 mol% Ir) and K2CO3 (25 mol%) gave N-methyl-1,2,3,4-tetrahydroquinoxaline in 80% yield. Similar reactions of 2-(2-aminophenylamino)ethanol derivatives gave corresponding 1,2,3,4-tetrahydroquinoxaline products in moderate to high yields, although longer reaction time and higher catalyst loading were required. 1-Methyl-2,3,4,5-tetrahydro-1H-benzo[b][1,4]diazepine were also obtained in 68% yield after the reaction for seven days.
We reported the oxidative cyclization of amino alcohols leading to lactams catalyzed by [Cp*RhCl2]2 / K2CO3 system using acetone as a hydrogen acceptor.25 The results for the synthesis of six- or seven-membered benzo-fused lactams are summarized in Table 3-3. When the reaction of 3-(2-aminophenyl)propanol was performed at 100 °C for 20 hours in the presence of [Cp*RhCl2]2 (5.0 mol% Rh) and K2CO3 (10 mol%) in acetone, 3,4-dihydro-2(1H)-quinolinone was formed in 80% yield (entry 1). 3-(2-Aminophenyl)propanols bearing a substituent on the aromatic ring were converted into the corresponding 3,4-dihydro-2(1H)-quinolinones in moderate to excellent yields, respectively (entries 2-7). It should be noted that the present catalytic system was applicable to the synthesis of 1,3,4,5-tetrahydro-2H-1-benzazepin-2-one in good yield (86%) using 4-(2-aminophenyl)-1-butanol as a starting material (entry 8).
The results for the synthesis of five-membered benzo-fused lactams (oxindoles) are summarized in Table 3-4. 2-Aminophenethyl alcohols bearing a substituent on the aromatic ring, the methylene chain, and the nitrogen atom were converted into the corresponding oxindoles in moderate to high yields, respectively (entries 1-4). In contrast to the synthesis of dihydroquinolinones, the synthesis of oxindoles could be carried out at a lower temperature (acetone reflux).
A possible mechanism for the oxidative cyclization of amino alcohols leading to lactams catalyzed by [Cp*RhCl2]2 / K2CO3 system is shown in Scheme 3-4. The first step of the reaction would involve the coordination of amino alcohol to the rhodium center to give an intermediate A. Then, β-hydrogen elimination would occur to give an amino-aldehyde B and a rhodium hydride species C (step a). Insertion of acetone into the rhodium-hydride bond in C would occur to give a rhodium isopropoxide species D (step b), which is subject to the alkoxy exchange reaction with amino alcohol to regenerate A (step c). Concurrently, the intermediate B would undergo condensation to give a cyclic hemiaminal E (step d). Oxidation of E by the rhodium catalyst via β-hydrogen elimination would give the lactam product F (steps e and f). Thus, the Cp*Rh catalyst could play a dual role in both dehydrogenations of the alcohol as well as the hemiaminal.
4. FRIEDLÄNDER-TYPE CYCLIZATION FORMING QUINOLINES
Since quinoline ring is present in a number of natural and synthetic products exhibiting interesting pharmacological activities or physical properties, a variety of synthetic approaches for the preparation of quinolines have been investigated. The Friedländer reaction is one of the simplest and the most efficient methods.26 The Friedländer reaction is a base- or acid-catalyzed condensation of an aromatic 2-amino-substituted carbonyl compound with a carbonyl derivative containing a reactive α-methylene group followed by cyclodehydration (Scheme 4-1).
However, most of the these reactions reported so far suffer from the need for high temperatures or harsh conditions, low yields, and problems associated with the storage and stability of starting 2-amino-substituted carbonyl compounds. Thus, various catalytic systems for Friedländer-type cyclization (modified Friedländer reaction) using transition metal hydrogen transfer catalyst starting with 2-aminobenzyl alcohol leading to quinolines have been investigated since 2-aminobenzyl alcohol is less expensive and more stable than is 2-aminobenzaldehyde.
4.1. Ru-Catalyzed Reactions
Cho and Shim et al. reported the ruthenium-catalyzed oxidative cyclization of 2-aminobenzyl alcohol with ketones affording quinolines.27 When the reaction of 2-aminobenzyl alcohol with acetophenone was carried out at 80 °C for 1 hour in the presence of RuCl2(=CHPh)(PCy3)2 (1.0 mol% Ru) and KOH (100 mol%), 2-phenylquinoline was formed in the yield of 97%. Not only above catalyst (Grubbs' first generation catalyst) but also RuCl2(PPh3)3 and RuH4(PPh3)4, well-known catalysts for hydrogen transfer reactions,2,28 showed high catalytic activity for this reaction.29 The results of oxidative cyclization of 2-aminobenzyl alcohol with a variety of ketones are summarized in Table 4-1.
Alkyl aryl ketones were readily cyclized with 2-aminobenzyl alcohol irrespective of the examined functional groups on the aromatic ring to afford the corresponding 2-arylquinolines in excellent to good yields (entries 1-11). The yield of quinoline was not greatly affected by the position of the substituent on the aromatic ring of ketones, whereas the electronic nature of that had some relevance to the product yield. Lower reaction rate and yield were observed with acetophenones having nitro, hydroxy and cyano functional groups on the aromatic ring (entries 8-10). With alkyl heteroaryl ketones, the corresponding quinolines were also formed in high yields (entries 12-14). In the reaction of dialkylketones, the corresponding quinolines were obtained as a regioisomeric mixture, favoring cyclization at less-hindered position over α-methylene (entries 16 and 17). An array of alkyl, aryl, cyclic and benzo-fused cyclic ketones having only the methylene reaction site also afforded the corresponding products ranging from 66–90% (entries 19-23).
Based on above work, Cho and Shim et al. reported the oxidative cyclization of 2-aminobenzyl alcohol with secondary alcohols instead of ketones leading to quinolines (Scheme 4-2).30 When 2-aminobenzyl alcohol was allowed to react with 1-phenylethanol in the presence of RuCl2(=CHPh)(PCy3)2 (1.0 mol% Ru) and KOH in dioxane at 80 °C for 20 hours, 2-phenylquinoline was produced in only 10% yield. However, the addition of 1-dodecene as hydrogen acceptor increased the yield of 2-phenylquinoline to 52%. RuCl2(PPh3)3 also showed catalytic activity, and optimum result was obtained with catalyst loading of 2.0 mol% Ru.
Various secondary alcohols were subjected to react with 2-aminobenzyl alcohol. Representative results are summarized in Table 4-2. With aryl(methyl) carbinols the oxidative coupling and cyclization products were formed in the range of 71–87% yields (entries 1-6). The product yield was not significantly affected by the position and electronic nature of the substituent on the aromatic ring. The reaction proceeds likewise with heteroaryl(methyl) carbinols to give the corresponding 2-heteroaryl substituted quinolines (entries 7-9). 1-(2-Naphthyl)ethanol was also readily oxidatively coupled and cyclized with 2-aminobenzyl alcohol to afford 2-(2-naphthyl)quinoline in 90% yield (entry 10). From the reactions of alkyl(alkyl) carbinols, the corresponding quinolines were also produced in moderate to good yields (entries 11-14). The reaction of 1-phenyl-1-propanol, which has only methylene reaction site, also proceeded to give the corresponding quinoline in 61% yield (entry 15).
As to the reaction pathway, it seems to proceed via a sequence involving initial oxidations of both substrates to carbonyl compounds, cross aldol reaction under KOH to afford α,β-unsaturated ketone A, and cyclodehydration (Scheme 4-3). The initial oxidations of alcohols to carbonyl compounds, which proceed via oxidative addition of O–H bond to the ruthenium center and subsequent β-hydrogen elimination, are well documented in transition metal-catalyzed transfer hydrogenations. 1-Dodecene seems to act as a sacrificial hydrogen acceptor oxidizing [Ru]H2 generated in the initial oxidation stage to [Ru]. An alternative route for the product involves a sequence such as reduction of A to saturated ketone B, cyclodehyderation to form 3,4-dihydroquinoline C and dehydrogenation.
Kaneda et al. reported the oxidative cyclization of 2-aminobenzyl alcohol with ketones using ruthenium-grafted hydrotalcite (Ru/HT) as a heterogeneous catalyst (Scheme 4-4).31 When the reaction of 2-aminobenzyl alcohol with acetophenone was performed in toluene at 100 °C for 20 hours under O2 atmosphere in the presence of Ru/HT catalyst (3.0 mol% Ru), 2-phenylquinoline was formed in 84% yield. A variety of quinoline derivatives were synthesized by this catalytic system under base-free conditions. This is the first reported one-pot quinoline synthesis using heterogeneous catalysts. It should be also noted that Ru/HT could be reused with retention of a high product yield; a recycling experiment resulted in 81% yield of 2-phenylquinoline.
Ramón and Yus et al. reported oxidative cyclization of 2-aminobenzophenone with secondary alcohols affording multi-substituted quinoline derivatives using RuCl2(dmso)4 as a catalyst under solvent-free conditions.32 Representative results are summarized in Table 4-3. For example, when the reaction of 2-aminobenzophenone with 1-phenylethanol was conducted at 100 °C for 48 hours in the presence of RuCl2(dmso)4 (2.0 mol%), potassium tert-butoxide (100 mol%), and benzophenone (100 mol%) under solvent-free condition, 2,4-diphenylquinoline was isolated in the yield of 99% (entry 1). A variety of multi-substituted quinoline derivatives could be synthesized in good yields by this catalytic system (entries 2-9).
4.2. Other Reactions
Ishii et al. reported the iridium-catalyzed Friedländer-type reaction starting with 2-aminobenzyl alcohol and ketones.33 [Ir(cod)Cl]2 and IrCl3 showed high catalytic activity for the oxidative cyclization of these substrates affording quinoline derivatives. Representative results are summarized in Table 4-4. When 2-aminobenzyl alcohol was allowed to react with acetophenone in the presence of iridium catalyst (2.0 mol% Ir) and KOH (20 mol%) under solvent-free conditions, 2-phenylquinoline was obtained in good yield (90% with [IrCl(cod)]2 / PPh3 catalyst, 85% with IrCl3 catalyst). Several quinoline derivatives were synthesized by this catalytic system.
Cho et al. reported the recyclable palladium catalyst for the Friedländer-type reaction affording a variety of quinoline derivatives (Scheme 4-5).34 2-Aminobenzyl alcohol undergoes oxidative cyclization with an array of ketones in the presence of a palladium catalyst combined with PEG-2000 along with KOH to give quinolines in good yields. The palladium / PEG-2000 catalytic system could be easily recovered from reaction mixture and reused five times without any loss of catalytic activity. This protocol is the first recyclable transition metal-catalyzed strategy for Friedländer-type quinoline synthesis.
Cho et al. also reported the recyclable copper catalyst composed of CuCl2 / MS 4A for the Friedländer-type reaction (Scheme 4-6).35 When the oxidative cyclization of 2-aminobenzyl alcohol with acetophenone was carried out in dioxane at 100 °C in the presence of CuCl2 (5.0 mol% Cu) along with KOH (300 mol%) under O2 atmosphere for 20 hours, 2-phenylquinoline was obtained in 90% yield. The copper catalytic system could be easily recovered by simple filtration from the reaction mixture. The recovered catalyst could be reused 10 times without loss of catalytic activity.
5. CYCLIZATION OF AMINES WITH AMINES
Shim et al. have reported a series of synthetic methods of quinolines by Ru-catalyzed hetereoannulation of anilines with aliphatic amines. They reported ruthenium-catalyzed synthesis of 2-ethyl-3- methylquinolines from anilines and triallylamine.36 When a mixture of anilines (10 mmol), triallylamine (1 mmol), RuCl3·H2O (0.05 mmol), SnCl2·H2O (1 mmol) and PPh3 (0.15 mmol) in dioxane (10 mL) was stirred at 180 oC for 20 h under Ar, 2-ethyl-3-methylquinoline was obtained in 51% yield. The present cyclization could also be applied to several substituted anilines. The representative results are summarized in Table 5-1.
The yield was considerably affected by the electronic nature and the position of the substituent on aniline. When anilines having electron-donating substituents were used (entries 2, 3, 5, 6, 9, and 10), the yield was generally higher than those of anilines with electron-withdrawing substituent (entries 7 and 8). In the case of o-toluidine the reaction did not proceed at all toward quinoline formation, N-allyl-o-toluidine being only detectable product (entry 4). In the cases of meta-substituted anilines, the corresponding quinolines were obtained as a regioisomeric mixture in moderate yields, favoring the formation of 7-substituted isomers (entries 3, 5, and 8).
They also reported synthesis of quinolines via ruthenium-catalyzed amine exchange reaction between anilines and trialkylamines.37 When anilines (6 mmol) reacted with an array of trialkylamines (1 mmol) in the presence of a catalytic amount of RuCl3·nH2O (0.08 mmol) and bis(diphenylphosphino)methane (dppm) (0.12 mmol) together with SnCl2·2H2O (1 mmol) and 1-hexene (10 mmol) as hydrogen acceptor in dioxane at 180 °C for 20 h, the corresponding 2,3-disubstituted quinolines were produced in moderate to good yields. The representative results are summarized in Table 5-2. The yield was not decisively affected by the position of the substituent on aniline. With 4-chloroaniline having electron-withdrawing Cl substituent (entry 6), the product yield was low. When anilines having electron-donating character were used, the yields are moderate to good. However, compared with the cases of anilines having electron-donating character, much more N-alkylaniline was produced. This result indicates that the reaction proceeds competitively between heteroannulation and N-alkylation and the electronic nature of the substituent on aniline determines relative rate. In the case of m-toluidine, the quinolines were obtained as a regioisomeric mixture, favoring predominantly the formation of the 7-substituted isomer (entry 3).
The initial formation of imines by amine exchange reaction between anilines and trialkylamines seems to be a crucial step. Subsequent steps seem to be followed by the known Schiff-base dimerization and ruthenium-mediated heteroannulation (Scheme 5-1).
Shim et al. also reported Ru-catalyzed synthesis of quinolines from anilines and allylammonium chlorides in an aqueous medium via amine exchange reaction.38 When anilines (6 mmol) reacted with allylammonium chlorides (1 mmol) in the presence of a catalytic amount of RuCl2(PPh3)3 (0.05 mol) together with SnCl2·2H2O (1 mmol) in an aqueous medium (H2O-dioxane = 1 : 9, 10 mL) at 180 oC for 20 h, the quinolines were obtained in moderate to good yields. The representative results are summarized in Table 5-3. All reactions were also accompanied by the formation of N-propylanilines as a side product. The yield was generally lower in the reactions of ortho-substituted anilines (entries 4 and 9) than those in the reactions of meta- and para-substituted anilines. In the cases of meta-substituted anilines such as m-toluidine and m-anisidine (entries 3 and 6), a regioisomeric mixture of the corresponding quinolines was obtained, favoring 7-substituted isomers which were formed via less sterically hindered position on meta-substituted anilines. The reactions of an array of anilines with tetraallylammonium chloride under the identical reaction conditions gave higher yields than those in the reaction with allylammonium chlorides.
A plausible pathway is shown in Scheme 5-2. The reaction would proceed via an initial formation of imines by ruthenium-catalyzed amine exchange reactions between anilines and allylammonium chlorides. According to the well-known intermolecular alkyl group transfer between alkylamines through iminium ion complex catalyzed by transition metals such as palladium and ruthenium, Cycle A can rationalizes the transfer of allylic moiety from allylammonium chloride to aniline. Quaternary ammonium salt could be converted into tertiary amine by cleavage of the carbon-nitrogen bond in an aqueous medium. The initial coordination of tertiary amine to ruthenium followed by oxidative insertion of ruthenium in the adjacent C-H bond produces an alkylruthenium complex A, which rapidly equilibrates with an iminium ion complex B. Nucleophilic attack of aniline to the complex B gives α,β-unsaturated imine C along with [Ru]H2. The following selective reduction of C by [Ru]H2 affords imine D. Subsequently, the known Schiff-base dimerization producing E followed by ruthenium-mediated heteroannulation shown in Cycle B affords 2-ethyl-3-methylquinoline. Watanabe et al. reported that a ruthenium-catalyzed heterocyclization of the Schiff-base dimer E gave quinolines through oxidative addition of the ortho C-H bond of E to low valent ruthenium via orthometallation, insertion of C=N double bond into Ru-C bond of F, reductive elimination of G, and deamination and dehydrogenation of H.39
Cho and Shim et al. reported that ruthenium-catalyzed reductive cyclization of nitroarenes with trialkylamines affording quinolines.40 The representative results are summarized in Table 5-4.
When nitroarenes (2 mmol) were treated with trialkyamines (1 mmol) in the presence of a catalytic amount of RuCl2(PPh3)3 (0.04 mmol) together with SnCl2·2H2O (1 mmol) in toluene-H2O (9/1, 10 mL) at 180 °C for 20 h, the reductive cyclization products quinolines were produced in moderate to good yields with the concomitant formation of the corresponding N-alkyl anilines. With meta- and para-substituted nitroarenes (entries 3 and 4), the yield was higher than that when ortho-substituted nitroarene was used (entry 2). In the reaction of meta-substituted nitroarene, a regioisomeric mixture of the product quinolines were obtained, favoring 7-methyl isomer (entry 3), which was formed via less sterically hindered position. With nitroarenes having electron-withdrawing substituents such as p-chloro, p-acetyl, and p-benzoyl, the yields were lower (entries 5-7). In the case of two-methyl substituted nitroarene, 3,5-dimethylnitrobenzene (entry 9), the yield increased much more when compared with mono-substituted nitroarenes. From the reactions between 3,5-dimethylnitrobenzene and several trialkylamines, the corresponding quinolines were also produced in good yields (entries 10-12). On statistical calculation, it is necessary for the two butyl group transfer from tributylamine to 3,5-dimethylnitrobenzene to form the quinoline. Thus, the result of 85% yield indicates that at least two butyl groups out of three in tributylamine are available for the transfer.
Beller et al. reported the selective N-alkylation of aryl amines using cyclic alkyl amines in the presence of so-called Shvo catalyst.41 In this novel catalytic transformation three C–N bond cleaving and forming steps take place. When aniline (2 equiv.) and pyrrolidine (1 equiv.) were heated at 150 oC for 24 h in the presence of Shvo catalyst (1 mol%), N-phenylpyrrolidine was obtained in 32% isolated yield. The reaction of various aryl amines and three cyclic alkyl amines were investigated. The representative results are summarized in Table 5-5. Electron-rich aryl amines such as m/p-toluidine and m/p-anisidine gave the N-arylpyrrolidines in 31–67% yield (entries 2–5). The pharmaceutically important 3,4-(methylenedioxy)-aniline gave the corresponding product in 58% yield (entry 6). On the other hand, electron-poor haloanilines such as 4-fluoro-, 4-chloro-, and 4-bromoanilines gave the alkylated anilines in low (25-31%) yields (entries 7–9). Other cyclic amines like piperidine and 2-methylpyrrolidine do also react with electron-rich anilines to afford the cyclized products in 58% and 68% yields (entries 10 and 11).
The supposed reaction mechanism is illustrated in Scheme 5-3. Initially, ruthenium-catalyzed dehydrogenation of pyrrolidine should occur via coordination and β-hydride elimination. Then, nucleophilic attack of aniline on the resulting imine would give the aminal, ring opening of which followed by hydrogenation could yield the corresponding 1,4-diamine A. Here, dehydrogenation of the primary amino group would be fast compared to that of the secondary amine. Subsequent nucleophilic attack on the imine, elimination of ammonia and catalytic hydrogenation could finally lead to N-phenylpyrrolidine.
References
1. (a) K. Fujita and R. Yamaguchi, 'Iridium Complexes in Organic Synthesis,' ed. by L. A. Oro and C. Claver, WILEY-VCH Verlag GmbH & Co. KGaA, Weinheim, 2009, Chapter 5, pp 107-143 and references cited therein; (b) K. Fujita and R. Yamaguchi, Synlett, 2005, 560 and references cited therein. CrossRef
2. (a) Y. Watanabe, Y. Tsuji, and Y. Ohsugi, Tetrahedron Lett., 1981, 22, 2667; CrossRef (b) S.-I. Murahashi, K. Kondo, and T. Hakata, Tetrahedron Lett., 1982, 23, 229; CrossRef (c) T. Naota, H. Takaya, and S.-I. Murahashi, Chem. Rev., 1998, 98, 2599 and references cited therein. CrossRef
3. (a) K. Fujita, S. Furukawa, and R. Yamaguchi, J. Organomet. Chem., 2002, 649, 289; CrossRef (b) F. Hanasaka, K. Fujita, and R. Yamaguchi, Organometallics, 2004, 23, 1490; CrossRef (c) F. Hanasaka, K. Fujita, and R. Yamaguchi, Organometallics, 2005, 24, 3422; CrossRef (d) F. Hanasaka, K. Fujita, and R. Yamaguchi, Organometallics, 2006, 25, 4643. CrossRef
4. (a) K. Fujita, Z. Li, N. Ozeki, and R. Yamaguchi, Tetrahedron Lett., 2003, 44, 2687; CrossRef (b) K. Fujita, Y. Enoki, and R. Yamaguchi, Tetrahedron, 2008, 64, 1943. CrossRef
5. (a) K. Fujita, T. Fujii, and R. Yamaguchi, Org. Lett., 2004, 6, 3525; CrossRef (b) K. Fujita, T. Fujii, A. Komatsubara, Y. Enoki, and R. Yamaguchi, Heterocycles, 2007, 74, 673; CrossRef (c) K. Fujita, Y. Enoki, and R. Yamaguchi, Org. Synth., 2006, 83, 217.
6. K. Fujita, K. Yamamoto, and R. Yamaguchi, Org. Lett., 2002, 4, 2691. CrossRef
7. (a) R. Yamaguchi, S. Kawagoe, C. Asai, and K. Fujita, Org. Lett., 2008, 10, 181; CrossRef (b) R. Yamaguchi, M. Zhu, S. Kawagoe, C. Asai, and K. Fujita, Synthesis, 2009, 1220; CrossRef (c) K. Fujita, A. Komatsubara, and R. Yamaguchi, Tetrahedron, 2009, 65, 3624; CrossRef (d) K. Fujita and R. Yamaguchi, J. Synth. Org. Chem., Jpn., 2003, 61, 715; CrossRef (e) K. Fujita, J. Synth. Org. Chem., Jpn., 2008, 66, 322. CrossRef
8. (a) M. H. S. A. Hamid, P. A. Slatford, and J. M. J. Williams, Adv. Synth. Catal., 2007, 349, 1555; CrossRef (b) G. Guillena, D. J. Ramón, and M. Yus, Angew. Chem. Int. Ed., 2007, 46, 2358; CrossRef (c) G. E. Dobereiner and R. H. Crabtree, Chem. Rev., in press; (d) G. Guillena, D. J. Ramón, and M. Yus, Chem. Rev., in press.
9. L. Miao, S. C. DiMaggio, H. Shu, and M. L. Trudell, Org. Lett., 2009, 11, 1579. CrossRef
10. G. Cami-Kobeci, P. A. Slatford, M. K. Whittlesey, and J. M. J. Williams, Bioorg. Med. Chem. Lett., 2005, 15, 535. CrossRef
11. H. Aramoto, Y. Obora, and Y. Ishii, J. Org. Chem., 2009, 74, 628. CrossRef
12. L. U. Nordstrøm and R. Madsen, Chem. Commun., 2007, 5034. CrossRef
13. K. Fujita, Y. Kida, and R. Yamaguchi, Heterocycles, 2009, 77, 1371. CrossRef
14. As an earlier publication in 1998, van Koten et al. have reported ruthenium-catalyzed N-heterocyclization of primary amines with diols leading to piperidine and piperazine derivatives. R. A. T. M. Abbenhuis, J. Boersma, and G. van Koten, J. Org. Chem., 1998, 63, 4282. CrossRef
15. I. Yamaguchi, T. Sakano, H. Ishii, K. Osakada, and T. Yamamoto, J. Organomet. Chem., 1999, 584, 213. CrossRef
16. C. S. Cho, J. H. Kim, T.-J. Kim, and S. C. Shim, Tetrahedron, 2001, 57, 3321. CrossRef
17. C. S. Cho and S. G. Oh, Tetrahedron Lett., 2006, 47, 5633. CrossRef
18. M. H. S. A. Hamid and J. M. J. Williams, Chem. Commun., 2007, 725. CrossRef
19. S. J. Pridmore, P. A. Slatford, A. Daniel, M. K. Whittlesey, and J. M. J. Williams, Tetrahedron Lett., 2007, 48, 5115. CrossRef
20. M. H. S. A. Hamid, C. L. Allen, G. W. Lamb, A. C. Maxwell, H. C. Maytum, A. J. A. Watson, and J. M. J. Williams, J. Am. Chem. Soc., 2009, 131, 1766. CrossRef
21. (a) A. J. Blacker, M. M. Farah, M. I. Hall, S. P. Marsden, O. Saidi, and J. M. J. Williams, Org. Lett., 2009, 11, 2039; CrossRef (b) A. J. Blacker, M. M. Farah, S. P. Marsden, O. Saidi, and J. M. J. Williams, Tetrahedron Lett., 2009, 50, 6106. CrossRef
22. Y. Aoyagi, T. Mizusaki, and A. Ohta, Tetrahedron Lett., 1996, 37, 9203; CrossRef (b) Y. Tsuji, S. Kotachi, K.-T. Huh, and Y. Watanabe, J. Org. Chem., 1990, 55, 580. CrossRef
23. We have also reported the dehydrogenative reactions of alcohols and cyclic amines catalyzed by Cp*Ir complexes. (a) K. Fujita, N. Tanino, and R. Yamaguchi, Org. Lett., 2007, 9, 109; CrossRef (b) R. Yamaguchi, C. Ikeda, Y. Takahashi, and K. Fujita, J. Am. Chem. Soc., 2009, 131, 8410. CrossRef
24. C. T. Eary and D. Clausen, Tetrahedron Lett., 2006, 47, 6899. CrossRef
25. K. Fujita, Y. Takahashi, M. Owaki, K. Yamamoto, and R. Yamaguchi, Org. Lett., 2004, 6, 2785. CrossRef
26. (a) J. Marco-Contelles, E. Pérez-Mayoral, A. Samadi, M. C. Carreiras, and E. Soriano, Chem. Rev., 2009, 109, 2652 and references cited therein; CrossRef (b) F. Domínguez-Fernández, J. López-Sanz, E. Pérez-Mayoral, D. Bek, R. M. Martin-Aranda, A. J. López-Peinado, and J. Čejka, ChemCatChem, 2009, 1, 241 and references cited therein. CrossRef
27. C. S. Cho, B. T. Kim, T.-J. Kim, and S. C. Shim, Chem. Commun., 2001, 2576. CrossRef
28. G.-Z. Wang and J.-E. Bäckvall, J. Chem. Soc. Chem. Commun., 1992, 337. CrossRef
29. Friedländer-type reactions of 2-aminobenzyl alcohol with ketones catalyzed by ruthenium complexes bearing N-heterocyclic carbene (NHC) ligands have been reported by Verpoort et al.: (a) H. V. Mierde, N. Ledoux, B. Allaert, P. V. D. Voort, R. Drozdzak, D. D. Vos, and F. Verpoort, New. J. Chem., 2007, 31, 1572; CrossRef (b) H. V. Mierde, P. V. D. Voort, D. D. Vos, and F. Verpoort, Eur. J. Org. Chem., 2008, 1625. CrossRef
30. C. S. Cho, B. T. Kim, H.-J. Choi, T.-J. Kim, and S. C. Shim, Tetrahedron, 2003, 59, 7997. CrossRef
31. K. Motokura, T. Mizugaki, K. Ebitani, and K. Kaneda, Tetrahedron Lett., 2004, 45, 6029. CrossRef
32. R. Martínez, D. J. Ramón, and M. Yus, Eur. J. Org. Chem., 2007, 1599. CrossRef
33. K. Taguchi, S. Sakaguchi, and Y. Ishii, Tetrahedron Lett., 2005, 46, 4539. CrossRef
34. C. S. Cho and W. X. Ren, J. Organomet. Chem., 2007, 692, 4182. CrossRef
35. (a) C. S. Cho, W. X. Ren, and S. C. Shim, Tetrahedron Lett., 2006, 47, 6781; CrossRef (b) C. S. Cho, W. X. Ren, and N. S. Yoon, J. Mol. Catal. A: Chem., 2009, 299, 117. CrossRef
36. C. S. Cho, B. H. Oh, and S. C. Shim, Tetrahedron Lett., 1999, 40, 1499. CrossRef
37. C. S. Cho, B. H. Oh, J. S. Kim, T.-J. Kim, and S. C. Shim, Chem. Commun., 2000, 1885. CrossRef
38. C. S. Cho, J. S. Kim, B. H. Oh, T.-J. Kim, S. C. Shim, and N. S. Yoon, Tetrahedron, 2000, 56, 7747. CrossRef
39. Y. Watanabe, Y. Tsuji, Y. Ohsugi, and J. Shida, Bull. Chem. Soc. Jpn., 1983, 56, 2452. CrossRef
40. C. S. Cho, T. K. Kim, B. T. Kim, T.-J. Kim, and S. C. Shim, J. Organomet. Chem., 2002, 650, 65. CrossRef
41. D. Hollmann, S. Bähn, A. Tillack, R. Parton, R. Altink, and M. Beller, Tetrahedron Lett., 2008, 49, 5742. CrossRef