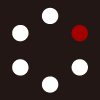
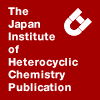
HETEROCYCLES
An International Journal for Reviews and Communications in Heterocyclic ChemistryWeb Edition ISSN: 1881-0942
Published online by The Japan Institute of Heterocyclic Chemistry
e-Journal
Full Text HTML
Received, 18th January, 2010, Accepted, 28th January, 2010, Published online, 29th January, 2010.
DOI: 10.3987/COM-10-11911
■ Photoinduced Electron Transfer-Initiated Selective Cyclization Reactions of (Z)-N-Benzoyl-α-dehydro(1-naphthyl)alaninamides into 4,5-Dihydrooxazole Derivatives
Yuhki Sato, Atsuhiko Yoshida, Tetsutaro Igarashi, and Tadamitsu Sakurai*
Material and Life Chemistry, Faculty of Engineering, Kanagawa University, 3-27-1 Rokkakubashi, Kanagawa-ku, Yokohama 221-8686, Japan
Abstract
An investigation was undertaken to gain understanding of substituent and solvent effects on the photoreactivity of the title 1-naphthylalanine N’,N’-disubstituted amides [(Z)-1] and the (Z)-1-derived product composition. Results indicated that the photoinduced electron transfer reaction of (Z)-1 in methanol containing triethylamine (TEA) proceeds less efficiently than that of the corresponding 1-naphthylalanine alkyl esters to afford cis-4,5-dihydrooxazoles in preference to the trans-isomers and also the former isomers undergo negligible isomerization to the latter isomers in the presence of TEA. Analysis of solvent effects substantiated that both the polarity of solvent and the ability of solvent to donate proton or to accept electron are major factors for controlling the photoreactivity and product composition ratio.Excited-state chemistry for organic molecules has continued to contribute to the development of convenient methods for synthesizing pharmacologically active heterocyclic compounds.1 Particularly, photoinduced electron transfer (PET) reactions have attracted much recent attention owing to the potential that these reactions are able to construct many types of heteroatom-containing ring systems with high efficiencies.1a,c,d In the course of a systematic study on the PET reaction of N-acyl-α-dehydronaphthylalaninamides, we found that these naphthylalaninamides undergo highly selective cyclization reactions to give 3,4-dihydrobenzo[f]quinolinone derivatives.2 On the other hand, N-substituted benzoyl-α-dehydronaphthylalanine alkyl esters activated by PET from triethylamine (TEA) were found to cyclize efficiently to 3,4-dihydrooxazole derivatives without forming any dihydrobenzo[f]quinolinones.3 Because both of these two types of photoproducts have asymmetric carbon atoms in their heterocyclic rings, the PET-initiated reactions of these α-dehydroamino acids were extended to asymmetric photocyclization by introducing various chiral auxiliaries into the starting N-acyl-α-dehydronaphthylalanines.4,5 However, naphthylalanine alkyl ester-derived cis-dihydrooxazoles underwent TEA-catalyzed isomerizations readily to the thermodynamically more stable trans-isomers.3a This fact has imposed some restrictions on tertiary amines, solvents, and N-benzoyl-α-dehydronaphthylalanine alkyl esters employed in the PET-initiated asymmetric cyclization reactions. A mechanistic investigation on the formation of the dihydroquinolinone ring from N-acyl-α-dehydronaphthylalaninamides confirmed that the N’-amide hydrogen in these naphthylalaninamide derivatives migrated to 3-position on the quinolinone ring.2a,b Thus, replacement of this labile amide hydrogen by an alkyl group closes pathway to the 3,4-dihydrobenzoquinolinone heterocycles to eventually force the starting N’,N’-disubstituted naphthylalaninamides to choose the other pathway to the 4,5-dihydrooxazole heterocycles. Since the N,N-disubstituted amino carbonyl group is considered to have weaker electron-withdrawing ability than the methoxycarbonyl, we predict that the latter heterocycles of the cis-configuration are not converted into the corresponding trans-isomers in the presence of TEA. To pave the way for an extension of our research into the asymmetric photocyclization of N-acyl-α-dehydroamino acid derivatives under various conditions, N,N-disubstituted (Z)-2-benzoylamino-3-(1-naphthyl)-2-propenamides [(Z)-1a–c] were synthesized followed by an examination of substituent and solvent effects on their photoreactivity and photoproduct composition in the presence of TEA (Chart 1).
The starting α-dehydronaphthylalaninamides of the (Z)-configuration were prepared in satisfactory yields by the Knoevenagel-type condensation between 1-naphthaldehyde and N-benzoylglycine in acetic anhydride containing sodium acetate, followed by the ring-opening reactions of the resulting (Z)-4-(1-naphthylmethylene)-2-phenyl-5(4H)-oxazolone with the corresponding 20% molar excess secondary amines, N,N-dimethylamine [(Z)-1a], N,N-dibenzylamine [(Z)-1b], and piperidine [(Z)-1c], in chloroform. After a nitrogen-saturated methanol solution of (Z)-1a (4.0x10–3 mol dm–3, 500 mL) containing TEA (0.10 mol dm–3) was irradiated at wavelengths longer than 280 nm from a 400 W high-pressure Hg lamp for 5 h at room temperature (conversion, 38%), the reaction mixture obtained was subjected to preparative thin layer chromatography over silica gel. This usual workup allowed us to isolate cis- and trans-4-dimethylaminocarbonyl-5-(1-naphthyl)-2-phenyl-4,5-dihydrooxazoles (cis-2a, 32%; trans-2a, 4% yield) having the vicinal coupling constants (J4,5) of 10.3 and 6.8 Hz in DMSO-d6, respectively, along with the (E)-isomer of 1a (44%) (Scheme 1). Since methyl (Z)-2-benzoylamino-3-(1-naphthyl)-2-propenoate was completely converted into the corresponding cis- and trans-dihydrooxazoles under the same irradiation conditions,3 the amidation of the methoxycarbony moiety in this α-dehydroamino acid
methyl ester is considered to greatly lower the photoreactivity in methanol.
The fact that conversion into the dihydrooxazole derivative 2, the photostability of which is high under the irradiation conditions tested, is not accompanied by any side reactions enabled us to monitor the photocyclization reaction by means of 1H NMR spectroscopy and to accurately estimate the composition of each compound after irradiation (Table 1). The data collected in Table 1 demonstrate that the thermodynamically less stable cis-dihydrooxazol isomer is formed in preference to the trans-isomer and the composition ratio of these two isomers remains unchanged (cis-2a/trans-2a = 7.4) irrespective of the conversion of 1a. Because negligible formation of 2a occurred via (E)-1a without TEA, the former observation confirms that PET-initiated cyclization to 2a is a kinetically-controlled process. In addition, no occurrence of the TEA-catalyzed isomerization of cis-2a to the thermodynamically more stable trans-isomer is substantiated by the latter observation, suggesting that replacement of the methoxycarbonyl group attached to the oxazole ring in 2 by the dimethylaminocarbonyl considerably reduces the acidity of a proton at 4-position of this ring.
We found previously that an increase in steric bulkiness of the alkoxycarbonyl moiety in N-benzoyl-α-dehydro(1-naphthyl)alanine alkyl esters enhances the selectivity for the corresponding cis-dihydrooxazole isomer.3 To ascertain whether a similar tendency is observed in the ET-initiated photocyclization of the corresponding N’,N’-disubstituted alaninamide derivatives, a nitrogen-saturated methanol solution of (Z)-1b or (Z)-1c (4.0´10–3 mol dm–3) was irradiated under the same conditions as those for (Z)-1a. Results obtained for (Z)-1c show that on introducing the piperidinocarbonyl group in 1, which is a somewhat bulkier than the dimethylaminocarbonyl group in 1a, the composition ratio of cis-2 to trans-2 is increased by 7% (7.4→7.9) with a decrease in the photochemical conversion of 1 (38%→29% at 5 h irradiation) (Table 1). It is thus assumed that ET-initiated photochemical transformation into the dihydrooxazole isomers proceeds through the same mechanism as that proposed in a previous study, as shown in Scheme 2. The preferential formation of the cis-isomer has been proposed to be due to much less steric hindrance for hydrogen shift from the biradical intermediate II having the cis-configuration than that from the trans-II. Comparison of the composition ratio of cis-2 to trans-2 between the naphthylalaninamide and naphthylalanine alkyl ester derivatives reveals that steric effect of the N’,N’-disubstituted aminocarbonyl group on the process of hydrogen shift in II is comparable in its magnitude to that of the isopropoxycarbonyl group in the latter derivative.3 On the other hand, irradiation of a nitrogen-saturated methanol solution of (Z)-1b was conducted in the presence of TEA under the same conditions but resulted in an appearance of a complicated product mixture. As only the isomerization into (E)-1b was observed without TEA, fragmentation of the 1b-derived anion radical and TEA cation radical pair initially formed by ET is considered to generate benzyl radical and debenzylated anion intermediates eventually affording a mixture of complicated products.
A previous study on the PET-initiated cyclization of N-acyl-α-dehydronaphthylalaninamide derivatives demonstrated that back electron transfer occurs efficiently within the alaninamide-derived anion radical/TEA cation radical pair intermediate to deactivate the reaction.2d This finding led us to propose that stability of the anion radical/cation radical pair is a major factor influencing the photoreactivity of these derivatives. Taking into account that both the polarity of solvent and the ability of solvent to form a hydrogen bond may affect the stability and conformation of the ion radical pair (I) and biradical (II) intermediates, effects of protic and aprotic solvents on the photochemical conversion (photoreactivity) of 1a and on the composition ratio of cis-2a to trans-2a were explored. In Table 2 are collected the data obtained by irradiating (Z)-1a (4.0x10–3 mol dm–3, 10 mL) in each solvent containing TEA (0.10 mol dm–3) in parallel for 5 h on a merry-go-round apparatus using the same filter and light source. An analysis of aprotic solvent effect using acetonitrile and 1,2-dichloroethane on the conversion of 1a clearly shows that an increase in the solvent polarity markedly reduces the conversion (58%→8.8%). Since the polar solvent, acetonitrile, stabilizes the ion radical pair intermediate I and also accelerates a dissociation into the corresponding anion and cation radicals to a greater extent, as compared to the less polar solvent, 1,2-dichloroethane, this result suggests the involvement of I as a precursor of the biradical intermediate II and additionally the much lower reactivity of dissociated ion radicals (Scheme 2). The large difference in photochemical conversion between acetonitrile and 1,2-dichloroethane makes it highly probable that the solvent polarity contributes mainly to promoting the dissociation into ion radicals and in the latter solvent a charge transfer interaction between the solvent molecule and the 1a-derived anion radical enhances the stability of I to result in an increase of the reactivity toward cyclization to II. On the other hand, a comparison of the photochemical conversions of 1 in acetonitrile and methanol of comparable polarity to each other supports the major role of a hydrogen-bonding interaction between the polar protic solvent and I in the stabilization of this intermediate.6 Much lower conversions in 2-propanol (9.3%) and 2-methyl-2-propanol (5.0%) than that in methanol reflect a considerably weaker hydrogen-bonding interaction between 2-propanol or 2-methyl-2-propanol and the anion radical in I. In this less polar protic solvent back electron transfer is very likely to occur efficiently causing a decrease in the conversion.
As already described, the composition ratio of cis-2 to trans-2 is determined approximately by the magnitude of steric hindrance to hydrogen shift in the biradical intermediate II, imposed by the bulky 1-naphthyl group. Because TEA is able to form a hydrogen bond to the imino hydrogen of this intermediate, there is a potential that TEA-assisted hydrogen shift proceeds on the opposite side of the naphthalene ring to avoid a steric repulsion between this aromatic ring and TEA (Figure 1). If so, it is expected that on enhancing the polarity of solvent or the ability of solvent to donate proton, the above hydrogen bond is weakened to result in a decrease in the relative composition of cis-2a owing to the diminished steric hindrance to the hydrogen shift process affording trans-2a. The data given in Table 2 reveal that this relative composition has a strong tendency to decrease with increasing the polarity of solvent (ClCH2CH2Cl< t-BuOH < i-PrOH < MeOH< MeCN) as well as the ability of solvent to form a hydrogen bond (MeCN< MeOH). This tendency is consistent with our expectation and, hence, provides a piece of evidence for the involvement of TEA-assisted hydrogen shift giving the cis-dihydrooxazole isomer preferentially. Much larger relative composition of cis-2a than expected in 1,2-dichloroethane implies a contribution of the charge transfer interaction between this solvent molecule and the ring nitrogen atom in the intermediate II that imposes an additional steric hindrance on hydrogen shift from II to trans-2a.
EXPERIMENTAL
General methods
1H and 13C NMR and IR spectra were taken with a JEOL JNM-A500 spectrometer and a Shimadzu Prestige-21 infrared spectrophotometer, respectively. Chemical shifts were determined using tetramethylsilane as an internal standard. Elemental analyses were performed on a Perkin-Elmer PE2400 series II CHNS/O analyzer. TEA was fractionally distilled from sodium hydroxide. Methanol and acetonitrile were purified according to the standard procedure and freshly distilled prior to use.6 All other solvents and reagents used were obtained from commercial sources and were of the highest grade available.
General procedure for the synthesis of (Z)-4-(1-naphthylmethylene)-2-phenyl-5(4H)-oxazolone
1-Naphthaldehyde (90 mmol) was slowly added to an acetic anhydride solution (110 mL) of N-benzoylglycine (84 mmol) and sodium acetate (61 mmol) at rt and the resulting mixture was heated at 60–65 °C for 1 h with stirring. The reaction mixture was cooled overnight in an ice bath. The solid separated out was collected by filtration with suction and was washed with water, a small amount of cold EtOH, and then dry hexane. After the crude product was air-dried at rt, it was recrystallized from hexane-CHCl3 to give yellow crystals in a 60% yield.
(Z)-4-(1-Naphthylmethylene)-2-phenyl-5(4H)-oxazolone: mp 166.0–167.0 °C. IR (KBr): 1795, 1645 cm–1. 1H NMR (500 MHz, CDCl3): δ 7.52–7.63 (6H, m), 7.88 (1H, d, J = 8.6 Hz), 7.95 (1H, d, J = 8.6 Hz), 8.10 (1H, s), 8.18 (2H, d, J = 7.6 Hz), 8.29 (1H, d, J = 8.6 Hz), 9.02 (1H, d, J = 6.7 Hz). Anal. Calcd for C20H13NO: C, 80.25; H, 4.38; N, 4.68. Found: C, 80.31; H, 4.40; N, 4.83. These physical and spectroscopic data were in good agreement with those of (Z)-4-(1-naphthylmethylene)-2-phenyl-5(4H)- oxazolone prepared in a previous study.3b
General procedure for the synthesis of (Z)-N-benzoyl-α-dehydro(1-naphthyl)alaninamide derivatives [(Z)-1a–c]
(Z)-4-(1-Naphthylmethylene)-2-phenyl-5(4H)-oxazolone (3.3 mmol) was added to dry CHCl3 (50 mL) containing dimethylamine (50 wt% aqueous solution, 4.0 mmol), dibenzylamine (4.0 mmol), or piperidine (4.0 mmol) and the resulting solution was allowed to stand for 1 h with stirring at rt. The reaction mixture was concentrated to dryness and the resulting residue was dissolved in EtOH and then treated with activated charcoal powder. After removal of the solvent under reduced pressure, the crystalline solid obtained was recrystallized from ethyl EtOAc affording colorless crystals in a 70–95% yield.
(Z)-2-Benzoylamino-N,N-dimethyl-3-(1-naphthyl)-2-propenamide [(Z)-1a]: mp 158.0–158.5 °C; IR (KBr) ν/cm–1 = 1659, 1612; 1H NMR (500 MHz, DMSO-d6) δ = 2.96 (3H, s), 3.24 (3H, s), 6.88 (1H, s), 7.43 (2H, dd, J = 7.4, 7.4 Hz), 7.50 (1H, dd, J = 7.4, 7.4 Hz), 7.51–7.54 (3H, m), 7.69 (1H, d, J = 7.4 Hz), 7.83 (2H, d, J = 7.4 Hz), 7.87 (1H, d, J = 8.0 Hz), 7.94 (1H, d, J = 7.4 Hz), 8.00 (1H, dd, J = 8.0 Hz), 10.02 (1H, s); 13C NMR (125 MHz, DMSO-d6) δ = 35.0, 39.1, 119.7, 124.4, 125.6, 126.0, 126.2, 126.5, 127.8 (2C), 128.0, 128.3 (2C), 128.4, 131.0, 131.4, 131.8, 132.3, 133.1, 133.3, 165.6, 167.9. Anal. Calcd for C22H20N2O2: C, 76.72; H, 5.85; N, 8.13. Found: C, 76.48; H, 5.86; N, 8.16.
(Z)-2-Benzoylamino-N,N-dibenzyl-3-(1-naphthyl)-2-propenamide [(Z)-1b]: mp 189.0–189.5 °C; IR (KBr) ν/cm–1 = 1667, 1618; 1H NMR (500 MHz, DMSO-d6) δ = 4.64 (2H, s), 4.87 (2H, s), 7.01 (1H, s), 7.24 (1H, d, J = 7.6, 7.6 Hz), 7,26 (1H, dd, J = 7.6, 7.6 Hz), 7.33 (2H, dd, J = 7.6, 7.6 Hz), 7.34 (2H, dd, J = 7.6, 7.6 Hz), 7.36 (2H, d, J = 7.6 Hz), 7.39 (2H, d, J= 7.6 Hz), 7.44 (2H, dd, J= 7.6, 7.6 Hz), 7.45 (1H, dd, J= 7.6, 7.6 Hz), 7.47 (1H, dd, J= 7.6, 8.2 Hz), 7.50 (1H, dd, J = 6.9, 7.6 Hz), 7.54 (1H, dd, J = 7.6, 7.6 Hz), 7.73 (1H, d, J= 6.9 Hz), 7.74 (1H, d, J = 7.6 Hz), 7.85 (1H, d, J= 7.6 Hz), 7.86 (2H, d, J= 7.6 Hz), 7.91 (1H, d, J= 8.2 Hz), 10.18 (1H, s); 13C NMR (125 MHz, DMSO-d6) δ = 47.3, 51.3, 119.7, 124.1, 125.5, 126.0, 126.3, 126.7, 127.1 (2C), 127.7 (2C), 127.8 (4C), 128.3 (4C), 128.4, 128.5, 128.7 (2C), 130.8, 131.0, 131.8, 132.1, 133.1, 133.3, 136.9 (2C), 166.1, 169.0. Anal. Calcd for C34H28N2O2: C, 82.23; H, 5.68; N, 5.64. Found: C, 81.97; H, 5.87; N, 5.72.
(Z)-2-Benzoylamino-3-(1-naphthyl)-N,N-(pentan-1,5-diyl)-2-propenamide [(Z)-1c]: mp 170.0–171.0 °C; IR (KBr) ν/cm–1 = 1658, 1605; 1H NMR (500 MHz, DMSO-d6) δ = 1.50–1.66 (6H, m), 3.53 (2H, br s), 3.74 (2H, br s), 6.83 (1H, s), 7.42 (2H, dd, J = 7.4, 7.4 Hz), 7.48–7.54 (4H, m), 7.69 (1H, d, J = 6.8 Hz), 7.82 (2H, d, J = 7.4 Hz), 7.87 (1H, d, J = 7.9 Hz), 7.95 (1H, dd, J = 6.8 Hz), 8.00 (1H, d, J = 6.8 Hz), 10.01 (1H, s); 13C NMR (125 MHz, DMSO-d6) δ = 24.3 (2C), 25.2, 42.6, 48.2, 118.8, 124.4, 125.6, 125.9, 126.2, 126.5, 127.7 (2C), 128.0, 128.2 (2C), 128.4, 131.0, 131.3, 131.7, 132.4, 133.2, 133.3, 165.5, 166.6. Anal. Calcd for C25H24N2O2: C, 78.10; H, 6.29; N, 7.29. Found: C, 78.33; H, 6.27; N, 7.08.
General procedure for the irradiation of (Z)-1a–c To examine the distribution and composition of the (Z)-1-derived photoproducts, a nitrogen-saturated MeOH solution of (Z)-1 (4.0x10–3 mol dm–3, 500 mL) containing TEA (0.10 mol dm–3), placed in a Pyrex vessel, was irradiated at wavelengths longer than 280 nm from a 400 W high-pressure Hg lamp at rt (internal irradiation, Pyrex glass filter). At suitable time intervals, an aliquot (5 mL) of the solution was pipetted off and concentrated to dryness in vacuo. The resulting residue was dissolved in DMSO-d6 and subjected to 1H NMR spectral analysis. The composition was estimated from the area ratio of a given 1H NMR signal for each compound. After 5 h irradiation, the remaining solution was concentrated to dryness in vacuo and the resulting residue was subjected to preparative thin layer chromatography over silica gel for isolating the photoproducts. The mixed solvent of CHCl3 and EtOAc was used as a developing solvent. Physical and spectroscopic data of the photoproducts [cis-2a,c, trans-2a,c, and (E)-1a] isolated are as follows. Attempt to isolate (E)-1c was not made.
cis-4-Dimethylaminocarbonyl-5-(1-naphthyl)-2-phenyl-4,5-dihydrooxazole (cis-2a): mp 149.0–149.5 ºC (EtOAc-hexane); IR (KBr) ν/cm–1 = 1655, 1640; 1H NMR (500 MHz, DMSO-d6) δ = 2.07 (3H, s), 2.61 (3H, s), 6.00 (1H, d, J = 10.3 Hz), 6.73 (1H, d, J = 10.3 Hz), 7.47 (1H, dd, J = 7.4, 7.4 Hz), 7.52–7.60 (5H, m), 7.62 (1H, dd, J = 7.4, 7.4 Hz), 7.86 (1H, d, J = 7.4 Hz), 7.95 (1H, d, J = 7.4 Hz), 8.02 (2H, d, J = 7.6 Hz), 8.17 (1H, d, J = 8.0 Hz); 13C NMR (125 MHz, DMSO-d6) δ = 34.5, 37.0, 68.6, 80.3, 123.1, 124.2, 125.0, 125.6, 126.0, 127.3, 128.0 (2C), 128.1, 128.6, 128.7 (2C), 129.6, 131.9, 132.0, 132.6, 164.3, 170.3. Anal. Calcd for C22H20N2O2: C, 76.72; H, 5.85; N 8.13. Found: C, 76.45; H, 5.52; N, 8.54.
cis-5-(1-Naphthyl)-4-(piperidinocarbonyl)-2-phenyl-4,5-dihydrooxazole (cis-2c): oily liquid; IR (neat) ν/cm–1 = 1653, 1639; 1H NMR (500 MHz, DMSO-d6) δ = 0.69–0.71 (1H, m), 0.93–0.96 (1H, m), 1.53–1.57 (3H, m), 1.68–1.69 (1H, m), 3.24–3.28 (2H, m), 3.41–3.44 (1H, m), 3.71–3.75 (1H, m), 5.98 (1H, d, J = 10.3 Hz), 6.71 (1H, d, J = 10.3 Hz), 7.99 (1H, dd, J= 7.4, 7.4 Hz), 8.04–8.10 (5H, m), 8.13 (1H, dd, J = 7.4, 7.4 Hz), 8.39 (1H, d, J = 8.0 Hz), 8.47 (1H, d, J = 8.0 Hz), 8.53 (2H, d, J = 6.9 Hz), 8.72 (1H, d, J = 8.0 Hz); 13C NMR (125 MHz, DMSO-d6) δ = 24.3, 25.4, 25.7, 42.4, 46.6, 68.9, 81.2, 123.8, 125.2, 125.9, 126.4, 127.0, 128.1, 128.8 (2C), 128.9, 129.4, 129.5 (2C), 130.7, 132.5, 132.6, 133.5, 165.2, 166.4. Anal. Calcd for C25H24N2O2: C, 78.10; H, 6.29; N, 7.29. Found: C, 77.93; H, 6.32; N, 7.63.
trans-4-Dimethylaminocarbonyl-5-(1-naphthyl)-2-phenyl-4,5-dihydrooxazole (trans-2a): oily liquid; IR (neat) ν/cm–1 = 1655, 1614; 1H NMR (500 MHz, DMSO-d6) δ = 2.96 (3H, s), 3.16 (3H, s), 5.03 (1H, d, J = 6.8 Hz), 6.97 (1H, d, J = 6.8 Hz), 7.49–7.60 (6H, m), 7.63 (1H, dd, J = 7.4, 7.4 Hz), 7.83 (1H, d, J = 6.9 Hz), 7.93 (1H, d, J = 7.4 Hz), 8.01 (1H, d, J = 8.6 Hz), 8.03 (2H, d, J = 8.0 Hz); 13C NMR (125 MHz, DMSO-d6) δ = 36.3, 37.5, 75.1, 80.3, 122.9, 123.1, 126.1, 126.7, 127.2, 127.5, 128.8 (2C), 139.1, 129.5 (2C), 129.6, 129.9, 132.8, 134.1, 136.2, 163.3, 168.5. Anal. Calcd for C22H20N2O2: C, 76.72; H, 5.85; N 8.13. Found: C, 76.57; H, 5.93; N, 8.12.
trans-5-(1-Naphthyl)-4-(piperidinocarbonyl)-2-phenyl-4,5-dihydrooxazole (trans-2c): oily liquid; IR (neat) ν/cm–1 = 1653, 1639; 1H NMR (500 MHz, DMSO-d6) δ = 1.46–1.62 (6H, m), 3.42–3.50 (2H, m), 3.70–3.79 (2H, m), 5.03 (1H, d, J = 6.8 Hz), 7.00 (1H, d, J = 6.8 Hz), 7.51 (1H, dd, J = 7.4, 7.4 Hz), 7.54–7.61 (5H, m), 7.64 (1H, dd, J = 7.4, 7.4 Hz), 7.81 (1H, d, J = 8.0 Hz), 8.47 (1H, d, J = 7.4 Hz), 8.02 (2H, d, J = 7.4 Hz), 8.07 (1H, d, J = 8.0 Hz); 13C NMR (125 MHz, DMSO-d6) δ = 24.0, 25.5, 26.3, 43.2, 46.5, 74.5, 79.6, 122.1, 122.5, 125.6, 126.1, 126.6, 126.8, 128.1 (2C), 128.5, 128.9 (2C), 129.0, 129.2, 133.5, 135.8(2C), 165.3, 166.2. Anal. Calcd for C25H24N2O2: C, 78.10; H, 6.29; N, 7.29. Found: C, 77.78; H, 6.36; N, 7.26.
(E)-2-Benzoylamino-N,N-dimethyl-3-(1-naphthyl)-2-propenamide [(E)-1a]: mp 208.5–209.0 ºC (EtOAc-hexane); IR (KBr) ν/cm–1 = 1661, 1622; 1H NMR (500 MHz, DMSO-d6) δ = 2.52 (3H, s), 2.66 (3H, s), 7.20 (1H, s), 7.37 (1H, d, J = 6.9 Hz), 7.46 (1H, dd, J = 7.4, 7.4 Hz), 7.53–7.60 (4H, m), 7.62 (1H, dd, J = 7.4, 7.4 Hz), 7.86 (1H, d, J = 8.0 Hz), 7.94 (1H, d, J = 7.4 Hz), 7.99 (2H, d, J = 7.4 Hz), 8.08 (1H, d, J = 8.0 Hz), 10.51 (1H, s); 13C NMR (125 MHz, DMSO-d6) δ = 33.8, 37.2, 113.3, 123.7, 125.1, 125.4, 125.9, 126.4, 127.6(2C), 127.7, 128.3(2C), 128.4, 131.0, 131.2, 131.9, 133.0, 133.1, 133.2, 164.5, 165.6. Anal. Calcd for C22H20N2O2: C, 76.72; H, 5.85; N 8.13. Found: C, 76.61; H, 5.72; N, 8.14.
ACKNOWLEDGMENTS
This research was partially supported by a “Scientific Frontier Research Project” from the Ministry of Education, Sports, Culture, Science and Technology, Japan.
References
1. a) ‘Synthetic Organic Photochemistry,’ ed. by W. M. Horspool, Plenum, New York, 1984; b) ‘Handbook of Photochemistry and Photobiology,’ Vol. 2, ed. by H. S. Nalwa, American Scientific Publishers, Stevenson Ranch, 2003; c) ‘Synthetic Organic Photochemistry,’ ed. by A. G. Griesbeck and J. Mattay, Marcel Dekker, New York, 2005; d) A. G. Griesbeck, N. Hoffmann, and K. Warzecha, Acc. Chem. Res., 2007, 40, 128. CrossRef
2. a) K. Maekawa, T. Igarashi, K. Kubo, and T. Sakurai, Tetrahedron, 2001, 57, 5515; CrossRef b) T. Motohashi, K. Maekawa, K. Kubo, T. Igarashi, and T. Sakurai, Heterocycles, 2002, 57, 269; CrossRef c) K. Maekawa, H. Kajiwara, Y. Iseya, T. Igarashi, and T. Sakurai, Heterocycles, 2003, 60, 637; CrossRef d) K. Maekawa, A. Shinozuka, M. Naito, T. Igarashi, and T. Sakurai, Tetrahedron, 2004, 60, 10293; CrossRef e) K. Maekawa, K. Fujita, K. Iizuka, T. Igarashi, and T. Sakurai, Heterocycles, 2005, 65, 117. CrossRef
3. a) K. Maekawa, T. Sasaki, K. Kubo, T. Igarashi, and T. Sakurai, Tetrahedron Lett., 2004, 45, 3663; CrossRef b) K. Maekawa, N. Hishikawa, K. Kubo, T. Igarashi, and T. Sakurai, Tetrahedron, 2007, 63, 11267. CrossRef
4. a) K. Maekawa, K. Kubo, T. Igarashi, and T. Sakurai, Heterocycles, 2002, 57, 1591; CrossRef b) K. Maekawa, K. Kubo, T. Igarashi, and T. Sakurai, Tetrahedron, 2004, 60, 1183; CrossRef c) K. Maekawa, K. Kubo, T. Igarashi, and T. Sakurai, Tetrahedron, 2005, 61, 11211. CrossRef
5. a) Y. Sasaki, K. Maekawa, H. Watanabe, T. Matsumoto, K. Kubo, T. Igarashi, and T. Sakurai, Tetrahedron Lett., 2007, 48, 4765; CrossRef b) H. Watanabe, K. Maekawa, T. Igarashi, and T. Sakurai, Heterocycles, 2007, 74, 149. CrossRef
6. J. A. Riddick, W. B. Bunger, and T. K. Sakano, ‘Organic Solvents,’ 4th ed., Wiley, Chichester, 1986.