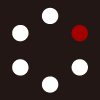
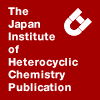
HETEROCYCLES
An International Journal for Reviews and Communications in Heterocyclic ChemistryWeb Edition ISSN: 1881-0942
Published online by The Japan Institute of Heterocyclic Chemistry
e-Journal
Full Text HTML
Received, 2nd February, 2010, Accepted, 12th April, 2010, Published online, 14th April, 2010.
DOI: 10.3987/REV-10-667
■ Bridged Nucleic Acids: Development, Synthesis and Properties
Satoshi Obika,* S. M. Abdur Rahman, Aki Fujisaka, Yuji Kawada, Takeshi Baba, and Takeshi Imanishi*
Graduate School of Pharmaceutical Science, Osaka University, 1-6 Yamadaoka, Suita, Osaka 560-0871, Japan
Abstract
Restricting the sugar moiety of a nucleic acid to a single conformation can be accomplished by forming a bridge in the sugar. A large number of bridged nucleic acids with variable bridged structures and conformations have been developed. The synthesis and properties of these artificial nucleic acid analogues are discussed. Such conformational restriction of the sugar moiety significantly improved the properties of the nucleic acid.CONTENTS:
1. INTRODUCTION
2. BEFORE BRIDGED NUCLEIC ACIDS —OUR ACYCLIC NUCLEOSIDES—
3. SEVERAL BRIDGED NUCLEIC ACIDS —BEFORE 2’,4’-BNA/LNA—
4. 2’,4’-BNA/LNA AND ITS DERIVATIVES —BRIDGED NUCLEIC ACIDS WITH N-TYPE SUGAR CONFORMATION—
5. 2’,4’-BNA/LNA DERIVATIVES WITH EXPANDED RING SIZE
6. BRIDGED NUCLEIC ACIDS WITH S-Type SUGAR CONFORMATION
7. PROPERTIES OF BRIDGED NUCLEIC ACIDS
8. ACKNOWLEDGEMENTS
9. REFERENCES
1. INTRODUCTION
Recently, much attention has been focused on the development of nucleic acid based technologies and therapies. Research interest in nucleic acid based technologies such as antisense,1 antigenes,2 ribozymes3 and RNA interference4 increases daily. Chemically modified oligonucleotides are highly applicable to these technologies. Oligonucleotides are chemically modified in order to improve target binding affinity, nuclease resistance, target specificity, cellular uptake and biodistribution. Several nucleic acids, modified either in the sugar, the base or the backbone have been developed during the past few decades:5-7 Some of these modified nucleic acids exhibited increased hybridizing ability while other characteristics remained unchanged or diminished. Indeed, most early attempts at modifying nucleic acids resulted in the improvement of only a single property, rather then the simultaneous improvement of all these properties mentioned above. For example, phosphorthioate modification improved the nuclease resistance of oligonucleotides, at the expense of target binding affinity.8 Despite a slight decrease in hybridizing ability, the first antisense drug containing a phosphorthioate oligonucleotide was approved by the FDA, and appeared on the market in 1998 for the treatment of cytomegalovirus-induced retinitis.9 Since then, tremendous effort has been made to develop novel nucleic acid derivatives with improved properties.
Our strategy for developing novel modified nucleic acid analogues was based on restricting the conformation of the sugar in the nucleotides. The sugar moiety of nucleic acids possesses high conformational flexibility and is in equilibrium between several conformations, such as N-type (North type) and S-type (South type) (Figure 1).
When nucleic acids hybridize with their complementary strand, this conformational freedom is drastically restricted. The entropy loss associated with conformational restriction during the hybridization process is an important consideration when developing a nucleic acid analogue with high binding affinity to its complementary strand. It is well known that the sugar conformations in A-form RNA predominantly exist in the N-type conformation, and that B-form DNA possesses an S-type sugar pucker. Therefore, modified oligonucleotides in which the sugar conformation is preorganized into the N-type conformation are expected to form stable duplexes with complementary single-stranded RNA (ssRNA), and that preorganized S-type oligonucleotides will form stable duplexes with single-stranded DNA (ssDNA). Preorganization of nucleic acids in a suitable form, for example by adding an extra bridge in the sugar moiety, would be a promising strategy for preventing loss of entropy.
Based on these concepts, we developed a novel class of nucleic acid analogues with fixed conformations by bridging the sugar moiety of the nucleotide; these derivative are called bridged nucleic acids (BNAs).10 Of the various BNA analogues synthesized to date, 2’-O,4’-C-methylene bridged nucleic acid (2’,4’-BNA),11,12 with N-type sugar conformation has found wide application in nucleic acid base technologies. This nucleic acid analogue is locked in the N-type sugar conformation. Nucleic acid derivatives with the N-type sugar pucker have been found to be very useful in antisense and antigene technologies.2,7 The enhanced affinity of the N-type sugar pucker for complementary ssRNA or dsDNA is attributed to improved stacking to the nearest neighbours and the quenching of local backbone motion, thereby reducing the entropic penalty in the free energy of stabilization of duplex and triplex structures.13 Hence, the development of N-type sugar puckered nucleic acids is essential for nucleic acid base technologies. 2’,4’-BNA, the most promising N-type nucleic acid analogue, was first synthesized in our laboratory in 1997.11 Soon after this publication, Wengel et al. independently synthesized the same nucleic acid analogue in 1998 and named it locked nucleic acid, or LNA.14,15 This nucleic acid analogue 2’,4’-BNA or LNA showed unprecedented target binding affinity and has emerged as the most promising nucleic acid analogue used in molecular biology.16-18 Because of its interesting properties, 2’,4’-BNA is commercially available and 2’,4’-BNA-modified antisense oligonucleotides have entered human clinical trials.19 The outstanding properties of 2’,4’-BNA have encouraged continued research in this field.
Apart from the development of 2’,4’-BNA/LNA, a number of other BNAs with N-type sugar conformation have been developed by us and others. Wengel et al. reported 2’,4’-BNA/LNA analogues such as 2’-amino-LNA20,21 and several derivatives22 and 2’-thio-LNA20 in which the 2’-oxygen atom of 2’,4’-BNA/LNA was replaced by 2’-nitrogen and 2’-sulfur atom, respectively. We have synthesized the amino congeners of 2’,4’-BNA analogues, namely, 3’-amino-2’,4’-BNA23,24 and 5’-amino-2’,4’-BNA,25 by dual modifications, i.e, modifications of both the sugar moiety and the backbone. 2’,4’-BNA with unnatural bases was also developed by us26-31 and other groups.32-34 Carbocyclic five-membered bridged BNA analogues A, B ware recently reported.35,36 BNA analogue C with a six-membered bridge structure with a 2’-C,4’-C architecture was first developed by Wang et al.37,38 Ethylene bridged nucleic acid (ENA) with a six-membered bridge structure bearing a 2’-O architecture was introduced by Koizumi et al.39,40 Chattopadhyaya et al. reported a 2’-N-containing ENA, i.e., Aza-ENA41 and carbocyclic ENA anlogues.35 Several carbocyclic six-membered 2’,4’-linked BNAs D, E were developed by Nielsen et al.42 We have developed several derivatives of a highly potent BNA analogue, 2’-aminomethylene BNA (2’,4’-BNANC),
bearing a six-membered bridge structure with a functionalizable nitrogen atom on the bridge.43-46
BNA analogues with a seven-membered bridged moiety, such as propylene bridged nucleic acid (PrNA),40 was developed by Koizumi et al., and 2’-O,4’-C-methylene oxymethylene BNA (2’,4’-BNACOC)47,48 was developed by us. A light-responsive 2’,4’-BNACOC derivative was synthesized by us very recently.49
A number of BNA analogues with a fixed S-type sugar pucker were also synthesized by us. Oligonucleotides consisting of an S-type sugar puckered nucleoside are expected to form stable B-form duplexes with complementary ssDNA. Therefore, this type of oligonucleotides might find applications in DNA microarrays50 or decoy strategies.51 The various S-type BNAs developed in our laboratory include 3’,4’-BNA,52-55 3’-amino-3’,4’-BNA,56 5’-amino-3’,5’-BNA,57,58 and various trans-3’,4’-BNA analogues,59-65 2’,5’-BNAON.66 Wengel synthesized a 3’,1’-aminomethylene linked DNA analogue67 G, and an α-configured arabino-type BNA, α-L-LNA68 and 2’-amino-α-L-LΝΑ69 which possessed the S-type sugar conformation.
This review will give an account of the development and synthesis of these various BNA analogues and their properties.
2. BEFORE BRIDGED NUCLEIC ACIDS –OUR ACYCLIC NUCLEOSIDES–
In 1995, we reported the synthesis of novel nucleoside dimer analogues 1-3 which contain an acyclic nucleoside and a carbamate linkage (Fig. 4).70 These formed the foundation of our development of novel artificial nucleic acids. The carbamate-linked oligonucleotides had been synthesized before; however, their ability to hybridize with DNA or RNA complements varied depending on the sequence of the oligonucleotides.71 The carbamate linkage is a little shorter than the phosphodiester linkage in natural nucleic acids. Therefore, we employed an acyclic nucleoside in combination with a rigid carbamate linkage in order to relax the strain caused in the hybridization step.
The synthesis of nucleoside dimers 1-3 was accomplished as shown in Scheme 1. (S)-Glycidol (compound 4) was treated with aqueous ammonia, and the amino and primary hydroxyl groups of the obtained compound were protected with Boc and TBDPS groups, respectively, to afford 5. Alcohol 5 was converted into methylthiomethyl ether 6, which was then treated with nucleobases in the presence of NBS to give the acyclic nucleoside analogues 7a-7c (B1 = thymin-1-yl (T), N6-benzoyladenin-9-yl (ABz), or N4-benzoylcytosin-9-yl (CBz)). After deprotection of the Boc group, 8 was coupled with natural 2’-deoxyribonucleoside 9 activated by N,N’-carbonyldiimidazole (CDI) to afford 1 via 10. The other isomer, 2, was readily synthesized in a similar manner using (S)-8a derived from (R)-glycidol. In addition, (S)-8a was also coupled with 12 to give the corresponding dimer 13, which was transformed into 3.
Nucleoside dimers 1 and 3 were converted into their phosphoramidite derivatives and incorporated into oligonucleotides using an automated DNA synthesizer. Unfortunately, UV melting experiments showed that the oligonucleotides containing these nucleoside dimers did not exhibit increased hybridization with their complementary strands, although these oligonucleotide derivatives were highly resistant to enzymatic degradation.72
3. SEVERAL BRIDGED NUCLEIC ACIDS –BEFORE 2’,4’-BNA/LNA–
The above results showed that conformationally flexible acyclic nucleosides decreased hybridization when incorporated into oligonucleotides. This finding inspired us to produce novel nucleic acid analogues bearing a conformationally restricted or locked sugar moiety. The advantages of a conformationally restricted sugar moiety were described above. To our knowledge, the first example of this new class of nucleic acids, bicyclonucleosides with an additional 3’,5’-ethylene bridge, was reported by Leumann et al. in 1993.73 This is a pioneering work had considerable impact on subsequent related research. The synthesis of the bicyclonucleosides is outlined in Scheme 2. The key intermediate 15 was prepared from starting material 14 in a 5-step sequence.73 The primary alcohol of 15 was oxidized to give aldehyde 16, which was converted into bicyclic compound 17. Coupling reaction of 17 with nucleobases was carried out under Vorbrüggen conditions74,75 to give anomeric mixtures of the bicyclo-DNA monomer 18. After deprotection and separation of each anomer, the bicyclo-DNA monomer was converted into
phosphoramidite derivative 19, which was successfully incorporated into oligonucleotides. Introduction of bicyclo-DNA into oligonucleotides stabilized the duplexes and triplexes formed with RNA and DNA.76
Later, the same laboratory reported several derivatives of bicyclo-DNA, including tricyclo-DNA, a tricyclic congener of bicyclo-DNA, which has a cyclopropane ring to further restrict the flexibility of the nucleic acid backbone.77 As shown in Scheme 3, tricyclo-DNA was prepared from diol 15, which was used in the synthesis of bicyclo-DNA. Compound 15 was converted to olefin 22 via a series of reactions including Dess-Martin periodinate oxidation, deketalization/acetylation, deprotonation and silylation. The cyclopropane ring was introduced into olefin derivative 22 by the Simmons-Smith reaction to give 23, and a subsequent coupling reaction with nucleobases and desilylation gave the tricyclo-DNA monomer 24.77
Another example is the methano carbocyclic nucleosides reported by Altman et al. in 1994.78 They prepared 4’,6’- and 1’,6’-methano carbocyclic thymidines 30 and 35 as shown in Schemes 4 and 5, respectively. Cyclopentenol 25 was stereoselectively converted to 26 by Simmons-Smith cyclopropanation, tosylation, azidation and reduction. Thymine nucleobase was constructed by reaction with the acylisocyanate derivative and subsequent acid-mediated cyclization. Debenzylation of 27 by Pd-catalyzed hydrogenolysis, thioacylation of 2’-OH, silyl protection of the 3’- and 5’-OH groups, and benzyloxymethyl (BOM) protection of the thymine nucleobase produced 28 which was subjected to radical reduction to give 29. Deprotection of the silyl and BOM groups afforded the desired 4’,6’-methano carbocyclic thymidine 30.
On the other hand, the synthesis of 1’,6’-methano carbocyclic thymidine 35 started with bicyclic lactone 31, which was converted to 32 by opening of the lacone and silylation.79 Cyclopropane ring formation under basic conditions and saponification gave bicyclic acid 33. Formation of the carbocyclic acid azide, in situ Curtius rearrangement,80 and quenching by benzyl alcohol and subsequent reduction afforded 34. Introduction of a thymine nucleobase followed by desilylation gave 4’,6’-methano carbocyclic thymidine 35.
Matteucci et al. developed a nucleoside trimer containing a 3’,6’-anhydroglucose derivative and 2’,5’ formacetal internucleoside linkages.81 As shown in Scheme 6, starting material 36 was treated with PhC(OMe)3/TsOH to give the 3,6-anhydroglucose derivative 37 via a glucose ortho ester intermediate. Acetolysis and subsequent introduction of a thymine nucleobase gave the bicyclic nucleoside 38, which was then converted to the methylthiomethyl ether 39. After removal of the 5’-O-benzoyl group and replacement with the phenoxyacetyl (Pac) group, the 2’-O-methylthiomethyl derivative 39 was converted to the methyl diphenylphosphinate 40. Condensation with the cytidine derivative and deprotection at the 5’-position gave dimer 41, which was then coupled with the diphenylphosphinate derivative of thymidine to give 43 via 42.
In 1997, we reported the synthesis of 3’-O,4’-C-methyleneribonucleosides (3’,4’-BNAs), which have a 2,6-dioxabicyclo[3.2.0]heptane ring system.52 The first synthesis of 3’,4’-BNA monomer was accomplished from a known 4’-(hydroxymethyl)uridine derivative 44 (Scheme 7).
Selective tosylation of the diastereotopic hydroxymethyl groups in 44 gave 45. Deprotection of the cyclohexylidene group of 45 and subsequent protection of the primary hydroxyl group afforded 46. Oxetane ring formation from 46 was achieved under basic conditions to give the desired nucleoside monomer 47.
We expected the formation of the 2’,4’-BNA monomer by a ring closure reaction between the 2’-OH group and the 4’-tosyloxymethyl group, since we had evidence that 1-methoxy congener 48 yielded 2’,4’-cyclized product 50 along with 3’,4’-BNA precursor 49 under the same reaction conditions (Scheme 8). However, we observed 47 as the sole isomer, probably due to a difference in the sugar conformation between 46 and 48. The 2’-OH group of 46 might be distant from the 4’-tosyloxymethyl group. We observed that the 3’,4’-BNA monomers bearing pyrimidine nucleobases were effectively synthesized by this route. Next, we attempted to develop an alternate route to access the purine congener of 3’,4’-BNA by introducing nucleobases into a 1-O-acetylribofuranose derivative and then forming an oxetane ring under basic conditions (Scheme 9).54,55 The known ribofuranose derivative 51 was silylated stereoselectively, and the obtained alcohol was tosylated to give 52, which was then converted into diacetate 53. After debenzylation and acetylation at the 3-hydroxy group, all four nucleobases (thymine, N4-benzoylcytidine, N6-benzoyladenine, and N2-isobutyrylguanine) were successfully introduced. Deacetylation, oxetane ring formation, and deprotection at the 5’-position proceeded smoothly to afford the desired 3’,4’-BNA monomer 55 having all four nucleobases. This nucleic acid analogue showed similar or slightly increased RNA affinity and decreased DNA affinity.53,82 The adenine analogue of 3’,4’-BNA was also used as a potent RNase L agonist.83
Wengel et al. reported the synthesis of a nucleoside analogue bearing an extra bridge between the 2’- and 3’-positions.84 As shown in Scheme 10, the known ulose 56 was used as a starting material. Stereoselective Grignard addition of an allyl group, removal of the TBDMS group, and subsequent benzylation gave 57. Thymine nucleobase was introduced after deprotection and acetylation (compound 58), and the configuration at the 2’-position of 59 was inverted via a 2-O,2’-O-anhydro intermediate 60. Oxidative cleavage of the allyl group and concomitant reduction gave the hydroxyethyl derivative 62. After tosylation of the primary hydroxyl group, cyclization was carried out under basic conditions to give a bicyclic nucleoside which was debenzylated to provide 2’,3’-BNA analogue 63.
4. 2’,4’-BNA/LNA AND ITS DERIVATIVES –BRIDGED NUCLEIC ACIDS WITH N-TYPE SUGAR CONFORMATION–
In 1997, we achieved the first synthesis of 2’,4’-BNA monomers bearing uracil and cytosine nucleobases,11 where the sugar puckering was exclusively N-type (C3’-endo conformation) due to the methylene bridge between the O2’ and C4’ atoms. On the other hand, Wengel et al. independently accomplished the synthesis of the same nucleic acid analogue in 199814 and called it Locked Nucleic Acid (LNA). Oligonucleotides containing the 2’,4’-BNA/LNA monomers showed unprecedented hybridization ability to their RNA complements,12 superior triplex-forming ability,85-90 and enzymatic stability.76 These properties of 2’,4’-BNA/LNA make them suitable for many kinds of genome technologies, e.g., oligonucleotide-based therapy and as probes for DNA detection. 2’,4’-BNA/LNA is now commercially available and is widely used in many research fields, as described above. The synthesis of 2’,4’-BNA/LNA has been summarized in previous reviews;10,82,91 therefore, only a brief account is presented here. As shown in Scheme 11, our first synthesis of the 2’,4’-BNA monomers started from compound 64, was an intermediate in the synthesis of 3’,4’-BNA (the precursor of compound 46 in Scheme 7).11,52 Compound 64 was converted to the 2,3-O-benzylidene derivative 65, which was subjected to regioselective reductive cleavage to afford 66 with the 3’-O-benzyl group. Ring closure and subsequent deprotection gave the desired 2’,4’-BNA monomer 67. The uracil nucleobase of 67 was converted to cytosine (compound 69) by a conventional method.
On the other hand, the synthesis of 2’,4’-BNA/LNA by Wengel et al. started from 4’-C-hydroxymethyl pentofuranose derivative 51 (Scheme 12).14,92 Regioselective 5-O-benzylation, acetylation, acetolysis, and a second acetylation gave 70. Incorporation of nucleobases and deacetylation afforded 71. Tosylation followed by ring closure under basic conditions and debenzylation gave the desired 2’,4’-BNA/LNA monomer 72.
Later, Koshkin et al. reported a more efficient route to the 2’,4’-BNA/LNA monomers (Scheme 13).93 Their synthesis also started from pentofuranose derivative 51, which was converted to di-O-mesylated compound 73. Acetolysis and the incorporation of nucleobases afforded 74, which was converted to bicyclic nucleoside 75 by one-pot deacetylation, ring closure, then nucleophilic displacement of the 5’-O-mesyl group by sodium benzoate. Subsequent saponification of the benzoyl group and removal of the 3’-O-benzyl group by hydrogenolysis gave the 2’,4’-BNA/LNA monomer 76.
As simple and interesting derivatives of 2’,4’-BNA/LNA, the oxygen atoms in the 2’,4’-BNA/LNA structure were replaced with other hetero atoms. For example, 2’-amino and 2’-thio congeners were developed by Wengel et al. (Scheme 14).21,22 The 4’-C-hydroxymethylnucleoside derivatives 77a and 77b was converted into di-O-tosylates 78a and 78b, which were treated with benzylamine to give 80 via 2,2’-anhydro intermediate 79. Debenzylation of 80 with ammonium formate and 10% Pd(OH)2/C gave the desired 2’-amino-LNA monomer 81. Alternatively, 2’-thio-LNA monomer 82 was obtained from 78b as shown in Scheme 14. The benzyl groups of 78b were removed and reprotected with TIPDS groups to afford 83. Treatment with potassium thioacetate followed by desilylation gave 82 via 84.
3’-Amino and 5’-amino congeners of 2’,4’-BNA were developed by our group.23-25 As shown in Scheme 15, 3’-amino-2’,4’-BNA monomer was synthesized starting from 3-azidofuranose derivative 85. Saponification, regioselective silylation, tosylation and acetolysis gave 86. Incorporation of thymine nucleobase and subsequent base-mediated deacetylation and ring closure smoothly proceeded to give 88. The desired 3’-amino-2’,4’-BNA monomer 90 was obtained by Pd-mediated reduction of the corresponding 3’-azido congener 89, which is an analogue of AZT with N-type sugar conformation.94
On the other hand, 5’-amino-2’,4’-BNA monomer 9425 and its N-methyl derivative 9595 were prepared from 91 (Scheme 16). Compound 92 was obtained from 91 via sequential nucleosidation, cyclization, desilylation and mesylation. Debenzylation produced 93, the 5’-mesyloxy group of which was replaced by an azido group and finally converted to 5’-amino-2’,4’-BNA monomer 94 by reduction under Staudinger conditions. Alternatively, 93 was treated with methylamine in a sealed tube to afford 5’-methylamino derivative 95.
A variety of unnatural nucleobases were also introduced into the 2’,4’-BNA/LNA skeleton for development of efficient triple helix-forming oligonucleotides or for other purposes.26-31,96,97 The structures of nucleosides with unnatural nucleobases are categorized into two groups: N-nucleoside and C-nucleoside. The former contains a linkage between C1’ in the furanose ring and a nitrogen atom in the heteroaromatics as unnatural nucleobases, and the latter involves a linkage between C1’ and a carbon atom of the unnatural nucleobase. For the construction of several N-nucleoside-type 2’,4’-BNA monomers, we chose 2-pyridone,27 5-methyl-2-pyridone,28 4-(3-benzamidophenyl)-2-pyridone,31 1-isoquinolone,29 imidazole and 2-aminoimidazole30 as unnatural nucleobases. These heteroaromatics were designed for recognition of C•G or T•A interuption sites in a homopurine•homopyrimidine dsDNA. The synthesis of the 2’,4’-BNA monomers bearing unnatural nucleobase 98 was accomplished by coupling these heteroaromatics and furanose derivative 96, 2’-O-deacetylation and subsequent ring closure, and 3’-O- and 5’-O-debenzylation (Scheme 17). Thus, this approach, based on Wengel’s synthetic route to 2’,4’-BNA/LNA (Scheme 12), is a good route for the construction of N-nucleoside-type 2’,4’-BNA/LNA bearing unnatural nucleobases. Koshkin also reported the N-nucleoside-type 2’,4’-BNA/LNA bearing other heteroaromatics.34
Compared to N-nucleosides, C-nucleosides can possess a great variety of heteroaromatics as unnatural nucleobases; therefore, we developed a synthetic route for C-nucleoside-type 2’,4’-BNA/LNA monomers.31,98,99 As shown in Scheme 18, aldehyde 104 as a key intermediate was prepared straightforwardly from methyl 4-hydroxymethylribofuranoside derivative 99. Aldehyde 104 was coupled with the lithium derivative of several heteroaromatics to afford diols 105 and 105’ as diastereomeric mixtures. On the other hand, the corresponding magnesium derivative of the heteroaromatics gave the desired diol 105 stereoselectively. Each stereoisomer of diols 105 and 105’ was separated and converted into ring closure products 106 and 106’ under Mitsunobu conditions. Subsequent debenzylation of desired β-isomer 106 afforded the C-nucleoside-type 2’,4’-BNA/LNA monomers. 98,99
Wengel et al. also prepared 2’,4’-BNA/LNA derivatives bearing benzene, naphthalene, and pyrene as nucleobases (Scheme 19).32,33 Starting material 107, where the p-methoybenzyl group was used to protect the 3-OH group, was converted into di-O-mesylate 108. Methanolysis and base-induced cyclization afforded methyl furanoside 110 as an anomeric mixture. Replacement of the mesyloxy group by the acetoxy group, followed by deacetylation and p-methoxybenzylation, gave 111, which was then treated with acetic acid to yield aldehyde 112. Likewise to our results (Scheme 18), coupling of aldehyde 112 with several aryl Grignard reagents, followed by cyclization under Mitsunobu conditions, proceeded smoothly to afford 114. Oxidative removal of the p-methoxybenzyl group was achieved using DDQ to give the desired diol 115.
A carbocyclic 2’,4’-BNA derivative was synthesized from the known dibenzyl derivative 116 by Chattopadhyaya et al. (Scheme 20).35 Oxidation of the primary alcohol of 116 followed by Wittig reaction gave 117, which was subjected to hydroboration-oxidation reaction to give alcohol 118. Swern oxidation and further Wittig reaction provided 119 into which a thymine nucleobase was incorporated. Deacetylation and esterification with phenyl chlorothioformate afforded 121. Cyclization by a radical mediated procedure followed by debenzylation provided the carbocyclic BNA monomer 122.
Another carbocyclic five-membered BNA analogue, 133 (Scheme 21), was synthesized very recently using the same starting material 116.36 Triflation, replacement with cyanide, acetolysis, and introduction of a thymine nucleobase afforded 123. Deacetylation of 123 and subsequent DIBAL reduction gave aldehyde 124, which was treated with O-benzylhydroxylamine to produce oxime 125. Esterification with phenyl chlorthioformate (compound 126) followed by radical cyclization afforded a mixture of two diastereomers, 127a and 127b, with 127a (7’R) as the major product. Oxidation of the benzyloxyamine of 127a with mCPBA provided oxime 128. Dess-Martin periodinate oxidation gave ketone 129 which was reduced stereoselectively to alcohol 130. Esterification with sterically less bulky (methylthio)thiocarbonate (comapred to the phenyl thiocarbonate group in 126) afforded 131, which was transformed to benzyl-protected carba-2’,4’-BNA analogue 132 via radical deoxygenation. Debenzylation provided the carbocyclic 2’,4’-BNA monomer 133.
5. 2’,4’-BNA/LNA DERIVATIVES WITH EXPANDED RING SIZE
2’,4’-BNA/LNA and its analogues have an additional methylene linkage between the 2’-oxygen/amino and 4’-carbon atoms to form a bicyclo[2.2.1]heptane skeleton. The sugar conformation is exclusively N-type, locked by the five-membered bridged structure. Various BNA analogues with expanded ring size having different bridge moiety architectures were also developed, some with a 2’-O linked bridge, some with a 2’-N bridge, and some with a 2’-C linked or carbocyclic BNA structure.
Ethylene-bridged nucleic acid (ENA) is one example of a six-membered BNA analogue.39,40 The synthesis of ENA was accomplished from the known dibenzyl derivative 116 as shown in Scheme 22. Compound 116 with a hydroxymethyl group was converted to compound 118 with an additional methylene group by Swern oxidation, Wittig reaction and hydroboration, followed by oxidation. After tosylation and acetolysis, nucleobases were introduced to give the 4’-(hydroxyethyl)ribonucleoside derivative 135 via 134. Base-induced one-pot deacetylation and ring closure followed by Pd-mediated hydrogenolysis afforded the desired ENA monomer 136. ENA monomers with a variety of bases have been synthesized.40
A thymine derivative of a 2’-aza analogue of ENA was reported by Chattopadhyaya et al.41 The synthesis of aza-ENA is shown in Scheme 23. Known dibenzyl derivative 116 was converted to 123 as described in Scheme 21. Deacetylation of 123 with NaOMe afforded alcohol 124, which was directly mesylated to give 137. Compound 137 was treated with DBU to produce 2,2’-anhydronucleoside 138, which was refluxed with 0.1 M H2SO4 in acetone to provide the arabino-type product 139 quantitatively. Compound 139 was converted to triflate 140 and the cyano group was reduced to an amino group using sodium tris(trifluoroacetoxy)borohydride (prepared in situ from NaBH4 and TFA) which spontaneously underwent intramolecular cyclization to give 141. Debenzylation with Pd(OH)2-C/ammonium formate followed by BCl3 provided the aza-ENA monomer 142. For efficient incorporation into oligonucleotides, N-protected aza-ENA analogues were synthesized. N-Trifluoroacetyl and N-phenoxyacetyl aza-ENA derivatives 143 and 144 were synthesized from 142 and 141, respectively. Compound 142 was reacted with excess ethyl trifluoroacetate in methanol to give N-trifluoroacetyl aza-ENA analogue 143. On the other hand, 141 was treated with Pac-Cl in pyridine and the resultant phenoxyacetyl derivative was subjected to debenzylation to produce the phenoxyacetyl derivative of aza-ENA 144. Both these derivatives were incorporated into oligonucleotides successfully.
We also developed 2’,4’-BNANC which contains a unique O-N linkage in the six-membered bridge structure (Scheme 24).43-46 The key step in the construction of 2’,4’-BNANC is the O-N bond formation. First, we attempted direct amination of the 2’-OH group of ribonucleoside derivative 145, but none of the desired product was obtained. Therefore, the O-N linkage was introduced by the following multi-step sequence. Ribonucleoside derivative 145 was converted into arabinonucleoside 146 by 2’-O-mesylation and concomitant hydrolysis. After debenzylation of the 3’- and 5’-OH groups, the resultant triol was selectively silylated with the tetraisopropyldisiloxy group to give 147. Triflation of the 2’-OH group, and subsequent SN2 reaction with N-hydroxyphthalimide afforded 148. Then, compound 148 was treated with hydrazine in the presence of DABCO and pyridine, a one-pot deprotection and cyclization reaction, to give 149 with the desired six-membered bridged moiety containing an O-N linkage. Protection or substitution on the nitrogen of the additional bridge followed by desilylation of the 3’- and 5’-OH groups gave the 2’,4’-BNANC monomer 150.
Several other six-membered 2’-C,4’-C-BNA analogues have been synthesized. In 1999, Wang et al. described the synthesis of 2’-C,4’-C bridged bicyclonucleotide 163 (Scheme 25).37 The hydroxyl groups at the 3- and 5-positions of 1-α-methyl arabinoside (compound 153) were protected with a silyl group to give 154. Oxidation of 154 followed by Wittig reaction afforded 155. Removal of the silyl group at the 3- and 5-positions, and further protection of the primary alcohol with a TBDMS group and the secondary alcohol with a benzyl group, afforded 156. Hydroboration of the olefin and protection with a dimethoxytrityl (DMTr) group gave 157. Desilylation followed by oxidation of the resulting alcohol furnished the aldehyde 158. Aldol condensation, Cannizzaro reaction and subsequent mesylation afforded 160. After removal of the DMTr group of 160, the obtained alcohol 161 was cyclized under basic conditions and then converted to 162. Reaction of 162 with a silylated nucleobase in the presence of SnCl4 and concomitant deprotection gave the nucleoside derivative 163.
Two BNA analogues with 3 carbons at the 2’,4’-linkage were synthesized by Nielsen et al. using a ring closing metathesis.42 The synthesis used 3’,5’di-O-protected uridine 164 as the starting material as shown in scheme 26. Compound 164 was converted to 2’-C-allyl derivative 166 by radical-mediated alkylation via 165. Selective removal of the 5’-O-TBDMS group with 80% acetic acid provided an alcohol, which was oxidized to the ketone and ultimately converted to diol 167 by aldol condensation and reduction. The more reactive 4’-C-hydroxymethyl group of 167 was protected with a benzoyl group, the remaining 5’-OH group was then protected with TBDMSCl, then the 4’-hydroxymethyl group was released via deprotection with sodium methoxide to deliver 169. Compound 169 was converted to diene 170 efficiently by Dess-Martin periodinate followed by Wittig reaction. Treatment with Grubbs catalyst caused the two terminal alkynes of 170 to react by ring closing metathesis to furnish the bicyclic nucleoside 171. The silyl protecting group was removed to give monomer 172 with an unsaturated bridged moiety. Hydrogenolysis using Adam’s catalyst provided the saturated derivative 173. Both 172 and 173 were incorporated into oligonucleotides via a phosphoramide protocol.
Synthesis of a six-membered carbocyclic BNA analogue was recently reported by Chattopadhyaya et al. (Scheme 27).35 Hydroboration-oxidation of 119 gave alcohol 174, which was subjected to Swern oxidation and Wittig reaction to afford olefin 175. Introduction of the thymine base by conventional methods gave 176. Deacetylation followed by esterification with phenyl chlorothioformate yielded ester 177. Radical-mediated cyclization produced exo product 178, which was converted to the desired nucleoside analogue 179.
2’,4’-BNA/LNA analogues with a seven-membered bridged structure such as PrNA40 and 2’,4’-BNACOC were also developed.47,48 The synthesis strategy for the PrNA monomer is depicted in Scheme 28. Swern oxidation and carbon chain elongation by Horner-Wadsworth-Emmons olefination of the pentofuranose derivative 180 gave the (E)-enolate 181. Selective reduction of the olefine using Pd(OH)2 afforded 182, which was then treated with L-selectride to give 4-(hydroxypropyl)furanose derivative 183. Tosylation, acetolysis and introduction of thymine nucleobase afforded 184. Deacetylation and subsequent ring closure proceeded smoothly under alkaline conditions to give 185. Deprotection of the 5’- and 3’-OH groups afforded the PrNA monomer 186.
The synthesis of 2’,4’-BNACOC bearing various nucleobases is shown in Scheme 29. Pentofuranose derivative 180 was converted to triacetate 187.47,48 Pyrimidine nucleobase (thymine or N4-benzoylcytosine) was introduced using silylated base to give 188. Deacetylation of 188 gave diol 189, which was cyclized by treatment with paraformaldehyde under acidic conditions to obtain 190 bearing a seven-membered bridged structure. Desilylation and debenzylation gave 2’,4’-BNACOC monomer 191 with a pyrimidine nucleobase. Synthesis of a 2’,4’-BNACOC analogue with a purine nucleobase was reported recently by our group using compound 187. To incorporate adenine nucleobase, triacetate 187 was coupled with N6-benzoyladenine to afford compound 192. Synthesis of the 2’,4’-BNACOC structure (methylene acetal formation) from this nucleoside analogue using a procedure similar to that applied for the pyrimidine base was troublesome due to depurination under acidic conditions. After several attempts, we found methyleneacetal formation proceeded using 193, where the N6-amide nitrogen is protected by a BOM group, upon treatment with NBS and DMSO. Sequential deprotection of the benzoyl, silyl, benzyl and BOM groups yielded 2’,4’-BNACOC-A monomer 195. 2’,4’-BNACOC with a guanine nucleobase was also synthesized from the same triacetate derivative 187.48 Introduction of the guanine nucleobase was carried out using O6-(diphenylcarbamoyl)-N2-isobutyrylguanine to give 196, which was deacetylated and then cyclized to form a seven-membered ring with a methylene acetal moiety (compound 198) using the same conditions used for the adenine derivative. Removal of the diphenylcarbamoyl group, desilylation and debenzylation furnished 2’,4’-BNACOC-G monomer 199.
Synthesis of light-responsive 2’,4’-BNACOC derivatives 203 (nucleoside monomer, Scheme 30) and 204 (oligonucleotide-containing 203, Scheme 31) was also accomplished in our laboratory recently.49 As shown in Scheme 30, two O-benzyl groups of 200 were removed by catalytic hydrogenolysis and the resulting diol was reprotected with a silyl group to give 201. Deacetylation of 201 with aqueous methylamine produced diol 202, which was treated with 6-nitroveratraldehyde in the presence of zinc chloride to give a bicyclic nucleoside, which was desilylated to afford the desired light-responsive 2’,4’-BNACOC monomer 203. This monomer was then transformed into the phosphoramidite derivative and incorporated into oligonucleotides via a conventional phosphoramidite protocol. Photoirradiation converted the oligonucleotide containing this BNA residue (structure 204, Scheme 31) into the unbridged nucleic acid derivative 205 with reduced target binding affinity. This derivative reacted with several nucleophiles such as ammonia or glutathione to produce 4’-C-hydroxymethyl RNA analogue 206, which possessed high binding affinity to ssRNA.
6. BRIDGED NUCLEIC ACIDS WITH S-TYPE SUGAR CONFORMATION
Apart from the N-type BNAs described above, we also synthesized several BNAs with S-type sugar conformation. S-type sugar puckered BNAs are interesting candidates for application in post-genome technologies such as DNA microarrays and decoy nucleic acids. The first S-type BNA synthesized by us was 3’,4’-BNA52-55 which was synthesized prior to 2’,4’-BNA and is described in the section 3. A slight modification of this BNA analogue, i.e., replacing 3’-oxygen atom by a nitrogen, resulted in a sugar-fused azetidine ring containing the nucleic acid derivative, 3’-amino-3’,4’-BNA.56 The sugar conformation of this compound is thought to be S-type, due to constraints on the azetidine ring. The synthesis of 3’-amino-3’,4’-BNA is shown in Scheme 32. The 3’-azido derivative 207 prepared from 85 was reduced by conventional hydrogenolysis and the resulting amino alcohol was treated with p-toluenesulfonyl chloride to give compound 208. Ring-closure and detosylation of the cyclized product 209 produced 210, which was also obtained from 207 in a single step using Staudinger’s conditions.100 After benzyloxycarbonylation or trifluoroacetylation (compounds 211 and 212, respectively), thymine nucleobase was introduced (compound 213) using standard procedures. Finally, one-pot deacetylation, followed by desilylation, furnished azetidine nucleosides 214 and 215. Unsubstituted azetidine derivative 216 was prepared by removing the trifluoroacetyl group with conc. ammonia.
Another nitrogen containing BNA analogue with the S-type sugar conformation, 5’-amino-3’,5’-BNA, was synthesized in our laboratory from 217 as depicted in Scheme 33.57,58 Osmium tetraoxide-mediated stereoselective dihydroxylation followed by tosylation of the primary alcohol afforded 218. Epoxidation of 218 and subsequent azidation provided 219, whose C5’ trityl (Tr) group was replaced with a tosyl group to give 220. Reduction of the azido group by palladium on carbon under a H2 atmosphere resulted in pyrrolidine ring formation to afford bicyclic nucleoside 221 as a salt, from which 5’-amino-3’,5’-BNA monomer 222 was obtained by treating with Et3N. Compound 221 can be converted without purification to the monomethoxytrityl (MMTr) derivative 223 for oligomerization.
Wengel et al. reported a 3’-amino-1’,3’-BNA analogue with S-type sugar pucker.67 The synthesis strategy is shown in Scheme 34. Known nucleoside analogue 223 was transformed into 224 via tritylation and mesylation. Compound 224 was converted to the azido derivative 226 by two different procedures. Intramolecular nucleophilic attack to form 2,3’-anhydro product 225 followed by nucleophilic opening (conditions iii and iv, respectively) resulted in 226 in relatively poor yield. Alternatively, inversion of the C3’ configuration (compound 227) followed by nucleophilic substitution provided 226 in higher yield. Removal of the dimethoxytrityl (DMTr) groups and subsequent mesylation gave dimesylate 228. Reduction of the azido group followed by cyclization afforded bicyclonucleoside 229, which was tritylated and demesylated to furnish 231 via 230.
The synthesis of α-L-ribo-configured BNA or LNA, α-L-LNA, was described by Wengel et al. (Scheme 35).68 Thymine nucleoside derivative 233 was synthesized from the β-L-threo-pentofuranose 232 straightforwardly as described in another report by Wengel et al.101 Mesylation of the hydroxyl groups and cyclization afforded 2’-O,5’-C-methylene linked bicyclofuranose derivative 235. This conversion from 234 to 235 is a reaction cascade involving anhydro intermediate formation, hydrolysis to afford C2’ inversion, intramolecular nucleophilic attack on the 5’-mesyloxy group, and removal of the remaining mesyloxy group. Debenzylation of 235 provided α-L-LNA monomer 236.
2’-Amino-α-L-LNA, a 2’-amino congener of α-L-LNA, was also synthesized from the same starting material 232 as shown in Scheme 36.69 Mesylation of 232 followed by treatment with acetyl chloride in methanol resulted in formation of an anomeric mixture of methyl furanoside 237. Azidation of 237 was accomplished via activation of the 2’-OH group to 2’-triflate to give 238. Introduction of thymine nucleobase was performed via acetolysis of 238 followed by treatment with silylated thymine in the presence of trimethylsilyl triflate. The anomeric mixture of azido nucleoside 239 was treated under Staudinger’s conditions100 to afford an easily separable bicyclic nucleoside 240. The α-anomer of 240 was transformed to 241 via protection of the secondary amine with a trifluoroacetyl group and subsequent demesylation by nucleophilic substitution with potassium acetate followed by deacetylation. The trifluoroacetyl group of 241 was removed to produce amino alcohol 242. Synthesis of 242 from 240 directly by demesylation was not achieved satisfactorily. Finally, tritylation and debenzylation afforded 2’-amino-α-L-LNA monomer 243.
We also synthesized a number of trans-3’,4’-BNA monomers59,60 (compounds 251, 253 and 256 in Scheme 37) from the known allyl derivative 244.102 Preparation of the hydroxymethyl derivative 245 was accomplished by a conventional three-step procedure using the aldol-Cannizzaro reaction. Selective benzylation and tosylation of the primary alcohols and oxidative cleavage of the olefin followed by reduction produced 246. Compound 246 was also accessible from a corresponding vinyl derivative via hydroboration albeit in lower yield.60 Incorporation of a thymine nucleobase into 246 according to Vorbruggen’s conditions afforded nucleoside derivative 247. Selective deacetylation of 247 followed by reprotection with a 2-methoxy-2-propyl (MOP) group and subsequent deprotection of the primary alcohol at the 3’-position furnished 248, which was cyclized to form 249 with a trans-3’,4’-BNA skeleton. Deprotection of the MOP protected hydroxyl group and debenzylation of the resulting 250 provided trans-fused 4,7-dioxabicyclo[4.3.0]nonane structure 251 or trans-3’,4’-BNA monomer. For effective incorporation of this monomer into oligonucleotides, the 2’-OH group must be removed or suitably protected. Removal of the 2’-OH was found to be difficult; therefore, a small protecting group (methyl group) was introduced to synthesize a 2-methoxy-trans-3’,4’-BNA analogue 253. To accomplish this, thymine nucleobase was protected with a benzyloxymethyl (BOM) group (compound 252), the 2’-OH group was methylated using a conventional procedure, and finally the BOM and benzyl groups were removed simultaneously to give 253. 1H-NMR and X-ray analysis revealed that 253 has a typical S-type sugar conformation. However, the trans-3,4-BNA analogue 253 could not be oligomerized since the corresponding phosphoramidite could not be synthesized. 60 This is attributed to the steric hindrance of the 2’-OMe group. Next, the arabino-type 2’-methoxy-trans-3,4’-BNA monomer 256 was synthesized by inverting the stereochemical configuration at the C2’-position. Oxidation of the 2’-OH to a keto group (compound 254) and subsequent stereoselective reduction (compound 255), followed by introduction of a
methyl group and debenzylation, provided arabino-type trans-3’,4’-BNA analogue 256 which was successfully incorporated into oligonucleotides.
Trans-fused 3’,4’-BNA with a 2’-substituent has low reactivity for both phosphoramidite formation and oligomerization reactions. Moreover, incorporation of arabino-type 2’-methoxy-trans-3’,4’-BNA 256 into oligonucleotides was found to destabilize DNA and RNA duplexes. Therefore, 2’-deoxy-trans-3’,4’-BNAs were synthesized as shown in Schemes 38 and 39.61,62,65 The first deoxy-type trans-3’,4’-BNA monomer synthesized (compound 262) has a seven-membered ring with a methylene acetal fused at the 3’- and 4’-positions of the furanose ring (3,5,8-trioxabicyclo[5.3.0]decane structure). 61,62 As shown in Scheme 38, stereoselective oxidation of the olefin of 220 and BOM protection of the thymine produced 257, which was transformed to 258 by protection-deprotection sequences. Oxidation of the primary hydroxyl group followed by aldol-Cannizzaro reaction gave 260 via 259. Selective benzylation provided 260 which was cyclized under acidic conditions and finally debenzylated to furnish 2’-deoxy-trans-3’,4’-BNA monomer 262.
Another deoxy-type trans-3’,4’-BNA with a six-membered fused ring having the 4,7-dioxabicyclo[4.3.0]nonane structure was also synthesized (Scheme 39).62,65 Selective benzylation of 260 gave 261, which was converted to 263 via tosylation and MOM deprotection. Oxidation of 263 followed by Wittig reaction of the resulting aldehyde 264 afforded enol ether 265. Compound 265 was transformed to 266 by removal of the methyl group followed by reduction. Ring-closure and debenzylation provided the 2’-deoxy-trans-3’,4’-BNA analogue 268 via 267. The phosphoramidites of this deoxy analogue were recently synthesized and incorporated into oligonucleotides. This analogue showed pronounced increased thermal stability of the duplex, in contrast to the arabino-type 2’-methoxy-trans-3’,4’-BNA analogue.63
Recently, another 2’-deoxy-trans-3’,4’-BNA monomer having a 4,8-dioxa-5-azabicyclo[5.3.0]decane skeleton was synthesized (Scheme 40).64 Mitsunobu reaction with 266 using N-hydroxyphthalimide gave 269, which was treated with hydrazine to deliver the aminoxy derivative 270. Carbamation of the amino group of 270 followed by cyclization afforded bicycle nucleoside 272 via 271. Removal of BOM produced 273, debenzylation of which gave the trans-3’,4’-BNA analogue 274.
A uridine analogue of 2’-O,5’-N bridged nucleic acid (2’,5’-BNAON) having S-type sugar pucker was synthesized using the known uridine derivative 275.66 Desilylation and mesylation of 275 produced mesylated diol 276, whose primary hydroxyl group was substituted by N,O-di-Boc-hydroxylamine under Mitsunobu conditions to give 277. Silyl protection and removal of the Boc groups afforded 278. Cyclization of 278 to form the 2’,5’-bridged structure failed to produce the desired product 279, but rather resulted in the formation of tetracyclic structure 280. Trying several experimental approaches resulted in the generation of 2’,5’-BNAON structure 281 from this tetracyclic compound 280 was achieved by N-acylative β-elimination using p-nitrophenyl chloroformate. Compound 281 was coupled with 3’-N-benzyloxymethyl-5’-O-(4,4-dimethoxytrityl)thymidine to give the fully protected dimer 282, which was transformed to dimer 283 containing the 2’,5’-BNAON residue. This dimer after phosphitylation was incorporated into oligonucleotide.
7. PROPERTIES OF BRIDGED NUCLEIC ACIDS
Restricting the conformation of a nucleic acid via bridging dramatically improves the properties of the nucleic acid in most cases. Hybridizing ability, sequence selectivity and nuclease resistance towards exo and endonucleases has been improved greatly by introducing BNA analogues. Hybridizing ability was monitored by UV melting temperature (Tm). Unprecedented increases in the Tm of oligonucleotides against complementary ssRNA, ssDNA and dsDNA was noticed by 2’,4’-BNA modification which has N-type sugar conformation. Typical increases in Tm per modification (ΔTm/modification) were found to range between +4 to +8 °C for complementary ssRNA and +2 to +5 °C for ssDNA.82,92 The enzymatic stability of 2’,4’-BNA modified oligonucleotides against snake venom phosphodiesterase (SVPDE) was markedly superior to that of natural oligonucleotides, as described previously.25,82 Resistance to endonucleases in serum was also found to be superior.103-105 The 3’-amino-2’,4’-BNA analogue showed almost the same hybridizing ability as the 2’,4’-BNA analogue towards complementary ssRNA and dsDNA, and affinity for ssDNA is also only slightly lower compare to that of 2’,4’-BNA, indicating that it has slightly better RNA selectivity.23,24 In addition, the nuclease resistance of 3’-amino-2’,4’-BNA is much higher than that of 2’,4’-BNA.24 The mismatch discrimination of both nucleic acid derivatives is similar and generally superior to that of natural oligonucleotides. The 5’-amino-2’,4’-BNA analogue also has a very high hybridizing profile to form duplexes and triplexes, and has remarkably high nuclease resistance.25 This BNA analogue is cleavable under mild acidic conditions, allowing it to be utilized as a probe for sequence-specific dsDNA sensing.95,106 The 2’,4’-BNA analogue with an unnatural base such as 2-pyridone was found to form a stable triplex with a C•G or T•A base pair, where natural nucleobases were less effective.27,28,31 Unnatural C-nucleosides possess universal recognition properties.32,33 Although extensive hybridization studies with five-membered carbocyclic 2’,4’-BNA analogues 122 and 133 developed by Chattopadhyaya et al. have not been done, a comparison with 2’,4’-BNA reveals that the hybridizing ability to ssRNA is lower than that of 2’,4’-BNA.35 However, the affinity for DNA complements was similar or decreased compared to natural oligonucleotides.35,36 Resistance to nucleolytic digestion was enhanced greatly by employing carbocyclic analogues.36
Among the six-membered BNA analogues, ENA showed similar or slightly lower duplex forming ability compared to 2’,4’-BNA,39 however, triplex formation was enhanced, especially with the fully modified TFO.107 The nuclease resistance of this analogue was found to be superior to that of the 2’,4’-BNA analogue.40 The duplex-forming ability of aza-ENA was considerably higher than that of natural oligonucleotides,41 but slightly lower than that of 2’,4’-BNA.35 Triplex formation was not investigated. Aza-ENA modified oligonucleotides showed improved nuclease resistance in denatured PAGE experiments, and were also reported to have superior serum stability compared to 2’,4’-BNA.41 The six-membered BNA analogue 2’,4’-BNANC, developed by us, showed extraordinarily high duplex- and triplex-forming ability.43-46 The duplex-forming ability of 2’,4’-BNANC is slightly higher than that of 2’,4’-BNA, and its triplex-forming ability is enhanced compared to 2’,4’-BNA.43 The sterically hindered 2’,4’-BNANC[N-Me] analogue showed high binding affinity comparable to 2’,4’-BNA and with better RNA-selectivity.44 Fully modified TFO, or TFO composed of consecutive 2’,4’-BNANC analogues, showed remarkable enhancement of target affinity compared to 2’,4’-BNA.44,46 Resistance to nucleolytic degradation was also much higher than that of 2’,4’-BNA. The BNA analogue 163 developed by Wang et al. showed moderate increase in Tm value.38 Carbocyclic BNA analogues 172 and 173 developed by Nielsen showed an increase in Tm from +2.3 to +4.5°C per medification against ssRNA.42 This increase in Tm was lower than that of the 2’,4’-BNA analogue using the same sequence. The Tm value towards ssDNA was decreased, implying that the carbocyclic analogue is RNA selective.35,36,42
PrNA with a seven-membered bridge structure showed decreased hybridizing ability to both ssRNA and ssDNA,40 although nuclease resistance was very high. On the other hand, 2’,4’-BNACOC developed by us exhibited excellent RNA-selective hybridizing ability.47,48 Fully modified 2’,4’-BNACOC duplex was found to possess extraordinarily high thermal stability.48 The properties of N-type BNAs developed by our group are summarized in Table 1.
5-Triphosphate analogues of 2’,4’-BNA and its derivatives, such as 2’,4’-BNANC and 2’,4’-BNACOC, were prepared and enzymatically incorporated into nucleic acids or oligonucleotides.18,108-110 Although further evaluation is still needed, these results would be highly useful for development of a variety of biological tools, such as aptamer. 108
Among the S-type BNAs, 3’,4’-BNA showed slightly decreased affinity for ssRNA and a considerable decrease in Tm against DNA.82 The 1’,3’-BNA analogue reported by Wengel showed a large decrease in duplex stability67 whereas α-L-LNA improved the thermal stability of duplexes significantly.68 5’-Amino-3’,5’-BNA possessed excellent binding affinity towards ssRNA and ssDNA targets and showed high nuclease resistance profiles. While 5’-amino-DNA had greatly decreased target binding affinity, 5’-amino-3’,5’-BNA showed significantly increased target affinity.57 The increased target affinity of the 5’-amino-3’,5’-BNA analogue is attributed to optimization of the γ dihedral angle via bridging. The trans-3’,4’-BNA analogue with a 2’-O-methyl substituent displayed lower binding affinity towards ssRNA and ssDNA complements, possibly due to steric repulsion exerted by the methyl group.60 On the other hand, 2’-deoxy-type trans-3’,4’-BNA analogues showed increased binding affinity towards DNA and RNA complements.63 Lastly, 2’,5’-BNAON was found to significantly decrease the thermal stability of duplexes.66
8. ACKNOWLEDGEMENTS
The authors thank all coworkers of Graduate School of Pharmaceutical Sciences, Osaka University for their effort and cooperation. Some works mentioned in this review were supported by Grant-in Aid for Science Research (KAKENHI) from Japan Society for the Promotion of Science (JSPS).
References
1. S. T. Crooke, Annu. Rev. Med. 2004, 55, 61. CrossRef
2. S. Buchini and C. J. Leumann, Curr. Opin. Chem. Biol., 2003, 7, 717. CrossRef
3. E. A. Doherty and J. A. Doudna, Annu. Rev. Biophys. Biomol. Struct., 2001, 30, 457. CrossRef
4. C. D. Novina and P. A. Sharp, Nature, 2004, 430, 161. CrossRef
5. S. M. Freier and K.-H. Altmann, Nucleic Acids Res., 1997, 25, 4429. CrossRef
6. R. P. Iyer, A. Roland, W. Zhou, and K. Ghosh, Curr. Opin. Mol. Ther., 1999, 1, 344.
7. J. Kurreck, Eur. J. Biochem., 2003, 270, 1628. CrossRef
8. C. A. Stein and Y.-C. Cheng, Science, 1993, 261, 1004. CrossRef
9. S. T. Crooke, Antisense Nucl. Acid Drug Dev., 1998, 8, vii.
10. T. Imanishi and S. Obika, Chem. Commun., 2002, 1653. CrossRef
11. S. Obika, D. Nanbu, Y. Hari, K. Morio, Y. In, T. Ishida, and T. Imanishi, Tetrahedron Lett., 1997, 38, 8735. CrossRef
12. S. Obika, D. Nanbu, Y. Hari, J. Andoh, K. Morio, T. Doi, and T. Imanishi, Tetrahedron Lett., 1998, 39, 5401. CrossRef
13. M. Petersen, C. B. Nielsen, K. E. Nielsen, G. A. Jensen, K. Bondensgaard, S. K. Singh, V. K. Rajwanshi, A. A. Koshkin, B. M. Dahl, J. Wengel, and, J. P. Jacobsen, J. Mol. Recognit., 2000, 13, 44. CrossRef
14. S. K. Singh, P. Nielsen, A. A. Koshkin, and J. Wengel, Chem. Commun., 1998, 455. CrossRef
15. J. Wengel, Acc. Chem. Res., 1999, 32, 301. CrossRef
16. J. S. Jepsen and J. Wengel, Curr. Opin. Drug Discovery, 2004, 7, 188.
17. H. Kaur, B. R. Babu, and S. Maiti, Chem. Rev., 2007, 107, 4672. CrossRef
18. R. N. Veedu and J. Wengel, Mol. BioSyst., 2009, 5, 787. CrossRef
19. M. Frieden and H. Orum, IDrugs, 2006, 9, 706.
20. S. K. Singh, R. Kumar, and J. Wengel, J. Org. Chem., 1998, 63, 6078. CrossRef
21. S. K. Singh, R. Kumar, and J. Wengel, J. Org. Chem., 1998, 63, 10035. CrossRef
22. M. D. Sorensen, M. Petersen, and J. Wengel, Chem. Commun., 2003, 2130. CrossRef
23. S. Obika, M. Onoda, K. Morita, J. Andoh, M. Koizumi, and T. Imanishi, Chem. Commun., 2001, 1992. CrossRef
24. S. Obika, S. M. A. Rahman, B. Song, M. Onoda, M. Koizumi, K. Morita, and T. Imanishi, Bioorg. Med. Chem., 2008, 16, 9230. CrossRef
25. S. Obika, O. Nakagawa, A. Hiroto, Y. Hari, and T. Imanishi, Chem. Commun., 2003, 2202. CrossRef
26. S. Obika, Y. Hari, K. Morio, and T. Imanishi, Tetrahedron Lett., 2000, 41, 221. CrossRef
27. S. Obika, Y. Hari, M. Sekiguchi, and T. Imanishi, Angew. Chem. Int. Ed., 2001, 40, 2079. CrossRef
28. S. Obika, Y. Hari, M. Sekiguchi, and T. Imanishi, Chem. Eur. J., 2002, 8, 4796. CrossRef
29. Y. Hari, S. Obika, M. Sekiguchi, and T. Imanishi, Tetrahedron, 2003, 59, 5123. CrossRef
30. Y. Hari, S. Obika, H. Inohara, M. Ikejiri, D. Une, and T. Imanishi, Chem. Pharm. Bull., 2005, 53, 843. CrossRef
31. S. Obika, H. Inohara, Y. Hari, and T. Imanishi, Bioorg. Med. Chem., 2008, 16, 2945. CrossRef
32. B. R. Babu, A. K. Prasad, S. Trikha, N. Thorup, V. S. Parmar, and J. Wengel, J. Chem. Soc., Perkin Trans. 1, 2002, 2509. CrossRef
33. C. Verhagen, T. Bryld, M. Raynkaer, S. Vogel, K. Buchalova, and J. Wengel, Eur. J. Org. Chem. 2006, 2538. CrossRef
34. A. A. Koshkin, J. Org. Chem., 2004, 69, 3711. CrossRef
35. P. Srivastava, J. Barman, W. Pathmasiri, O. Plashkevych, M. Wenska, and J. Chattopadhyaya, J. Am. Chem. Soc., 2007, 129, 8362. CrossRef
36. J. Xu, Y. Liu, C. Dupouy, and J. Chattopadhyaya, J. Org. Chem., 2009, 74, 6534. CrossRef
37. G. Wang, J.-L. Girardet, and E. Gunic, Tetrahedron, 1999, 55, 7707. CrossRef
38. G. Wang, E. Gunic, J.-L. Girardet, and V. Stoissavljevic, Bioorg. Med. Chem. Lett., 1999, 9, 1147. CrossRef
39. K. Morita, C. Hasegawa, M. Kaneko, S. Tsutsumi, J. Sone, T. Ishikawa, T. Imanishi, and M. Koizumi, Bioorg. Med. Chem. Lett., 2002, 12, 73. CrossRef
40. K. Morita, M. Takagi, C. Hasegawa, M. Kaneko, S. Tsutsumi, J. Sone, T. Ishikawa, T. Imanishi, and M. Koizumi, Bioorg. Med. Chem., 2003, 11, 2211. CrossRef
41. O. P. Varghese, J. Barman, W. Pathmasiri, O. Plashkevych, D. Honcharenko, and J. Chattopadhyaya, J. Am. Chem. Soc., 2006, 128, 15173. CrossRef
42. N. Albaek, M. Petersen, and P. Nielsen, J. Org. Chem., 2006, 71, 7731. CrossRef
43. S. M. A. Rahman, S. Seki, S. Obika, S. Haitani, K. Miyashita, and T. Imanishi, Angew. Chem. Int. Ed., 2007, 46, 4306. CrossRef
44. K. Miyashita, S. M. A. Rahman, S. Seki, S. Obika, and T. Imanishi, Chem. Commun., 2007, 3765. CrossRef
45. S. M. A. Rahman, S. Seki, K. Utsuki, S. Obika, K. Miyashita, and T. Imanishi, Nucleosides Nucleotides Nucleic Acids, 2007, 26, 1625. CrossRef
46. S. M. A. Rahman, S. Seki, S. Obika, H. Yoshikawa, K. Miyashita, and T. Imanishi, J. Am. Chem. Soc., 2008, 130, 4886. CrossRef
47. Y. Hari, S. Obika, R. Ohnishi, K. Eguchi, T. Osaki, H. Ohishi, and T. Imanishi, Bioorg. Med. Chem., 2006, 14, 1029. CrossRef
48. Y. Mitsuoka, T. Kodama, R. Ohnishi, Y. Hari, T. Imanishi, and S. Obika, Nucleic Acids Res., 2009, 37, 1225. CrossRef
49. K. Morihiro, T. Kodama, M. Nishida, T. Imanishi, and S. Obika, ChemBioChem, 2009, 10, 1784. CrossRef
50. A. Sassolas, B. D. Leca-Bouvier, and L. J. Blum, Chem. Rev., 2008, 108, 109. CrossRef
51. R. Morishita, J. Higaki, N. Tomita, and T. Ogihara, Circ. Res., 1998, 82, 1023.
52. S. Obika, K. Morio, D. Nanbu, and T. Imanishi, Chem. Commun., 1997, 1643. CrossRef
53. S. Obika, K. Morio, Y. Hari, and T. Imanishi, Bioorg. Med. Chem. Lett., 1999, 9, 515. CrossRef
54. S. Obika, K. Morio, Y. Hari, and T. Imanishi, Chem. Commun., 1999, 2423. CrossRef
55. S. Obika, K. Morio, D. Nanbu, Y. Hari, H. Itoh, and T. Imanishi, Tetrahedron, 2002, 58, 3039. CrossRef
56. S. Obika, J. Andoh, M. Onoda, O. Nakagawa, A. Hiroto, T. Sugimoto, and T. Imanishi, Tetrahedron Lett., 2003, 44, 5267. CrossRef
57. S. Obika, M. Sekiguchi, R. Somjing, and T. Imanishi, Angew. Chem. Int. Ed., 2005, 44, 1944. CrossRef
58. M. Sekiguchi, S. Obika, R. Somjing, and T. Imanishi, Nucleosides Nucleotides Nucleic Acids, 2005, 24, 1097. CrossRef
59. S. Obika, M. Sekiguchi, T. Osaki, N. Shibata, M. Masaki, Y. Hari, and T. Imanishi, Tetrahedron Lett., 2002, 43, 4365. CrossRef
60. M. Sekiguchi, S. Obika, Y. Harada, T. Osaki, R. Somjing, Y. Mitsuoka, N. Shibata, M. Masaki, and T. Imanishi, J. Org. Chem., 2006, 71, 1306. CrossRef
61. S. Obika, T. Osaki, M. Sekiguchi, R. Somjing, Y. Harada, and T. Imanishi, Tetrahedron Lett., 2004, 45, 4801. CrossRef
62. T. Osaki, S. Obika, Y. Harada, Y. Mitsuoka, K. Sugaya, M. Sekiguchi, R. Somjing, and T. Imanishi, Tetrahedron, 2007, 63, 8977. CrossRef
63. T. Kodama, K. Sugaya, Y. Harada, Y. Mitsuoka, T. Imanishi, and S. Obika, Heterocycles, 2009, 77, 1209. CrossRef
64. T. Kodama, K. Sugaya, T. Baba, T. Imanishi, and S. Obika, Heterocycles, 2009, 79, 873. CrossRef
65. T. Osaki, S. Obika, Y. Harada, Y. Mitsuoka, K. Sugaya, M. Sekiguchi, R. Somjing, and T. Imanishi, Nucleosides Nucleotides Nucleic Acids, 2007, 26, 1079. CrossRef
66. T. Kodama, C. Matsuo, H. Ori, T. Miyoshi, S. Obika, K. Miyashita, and T. Imanishi, Tetrahedron, 2009, 65, 2116. CrossRef
67. L. Kvaerno, R. H. Wightman, and J. Wengel, J. Org. Chem., 2001, 66, 5106. CrossRef
68. M. D. Sorensen, L. Kvaerno, T. Bryld, A. E. Hakansson, B. Verbeure, G. Gaubert, P. Herdewijn, and J. Wengel, J. Am. Chem. Soc., 2002, 124, 2164. CrossRef
69. T. S. Kumar, A. S. Madsen, J. Wengel, and P. J. Hrdlicka, J. Org. Chem., 2006, 71, 4188. CrossRef
70. S. Obika, Y. Takashima, Y. Matsumoto, K. Kuromaru, and T. Imanishi, Tetrahedron Lett., 1995, 36, 8617. CrossRef
71. A. Waldner, A. D. Mesmaeker, and J. Lebreton, Bioorg. Med. Chem. Lett., 1994, 4, 405. CrossRef
72. S. Obika, Y. Takashima, Y. Matsumoto, A. Shimoyama, Y. Koishihara, Y. Ohsugi, Y. Doi, and T. Imanishi, Bioorg. Med. Chem. Lett., 1996, 6, 1357. CrossRef
73. M. Tarkoy, M. Bolli, B. Schweizer, and C. Leumann, Helv. Chim. Acta, 1993, 76, 481. CrossRef
74. U. Niedballa and H. Vorbruggen, J. Org. Chem., 1974, 39, 3654. CrossRef
75. H. Vorbruggen, K. Krolikiewicz, and B. Bennua, Chem. Ber. 1981, 114, 1234. CrossRef
76. M. Tarkoy, M. Bolli, and C. Leumann, Helv. Chim. Acta, 1994, 77, 716. CrossRef
77. R. Steffens and C. Leumann, Helv. Chim. Acta, 1997, 80, 2426. CrossRef
78. K.-H. Altmann, R. Kesselring, E. Francotte, and G. Rihs, Tetrahedron Lett., 1994, 35, 2331. CrossRef
79. K.-H. Altmann, R. Imwinkelried, R. Kesselring, and G. Rihs, Tetrahedron Lett., 1994, 35, 7625. CrossRef
80. K. Ninomiya, T. Shioiri, and S. Yamada, Tetrahedron, 1974, 30, 2151. CrossRef
81. R. Zou and M. D. Matteucci, Tetrahedron Lett., 1996, 37, 941. CrossRef
82. T. Imanshi and S. Obika, J. Synth. Org. Chem., 1999, 57, 77.
83. K. Morita, M. Kaneko, S. Obika, T. Imanishi, Y. Kitade, and M. Koizumi, ChemMedChem, 2007, 2, 1703. CrossRef
84. P. Nielsen, H. M. Pfundheller, and J. Wengel, Chem. Commun., 1997, 825. CrossRef
85. S. Obika, Y. Hari, T. Sugimoto, M. Sekiguchi, and T. Imanishi, Tetrahedron Lett., 2000, 41, 8923. CrossRef
86. S. Obika, T. Uneda, T. Sugimoto, D. Nanbu, T. Minami, T. Doi, and T. Imanishi, Bioorg. Med. Chem., 2001, 9, 1001. CrossRef
87. H. Torigoe, S. Obika, and T. Imanishi, Nucleosides Nucleotides Nucleic Acids, 2001, 20, 1235. CrossRef
88. H. Torigoe, Y. Hari, M. Sekiguchi, S. Obika, and T. Imanishi, J. Biol. Chem., 2001, 276, 2354. CrossRef
89. H. Torigoe, T. Katayama, S. Obika, A. Maruyama, and T. Imanishi, Nucleosides Nucleotides Nucleic Acids, 2005, 24, 635. CrossRef
90. H. Torigoe, A. Maruyama, S. Obika, T. Imanishi, and T. Katayama, Biochemistry, 2009, 48, 3545. CrossRef
91. S. M. A. Rahman, T. Imanishi, and S. Obika, Chem. Lett., 2009, 38, 512. CrossRef
92. A. A. Koshkin, S. K. Singh, P. Nielsen, V. K. Rajwanshi, R. Kumar, M. Meldgaard, C. E. Olsen, and J. Wengel, Tetrahedron, 1998, 54, 3607. CrossRef
93. A. A. Koshkin, J. Fensholdt, H. M. Pfundheller, and C. Lomholt, J. Org. Chem., 2001, 66, 8504. CrossRef
94. S. Obika, J. Andoh, T. Sugimoto, K. Miyashita, and T. Imanishi, Tetrahedron Lett., 1999, 40, 6465. CrossRef
95. S. Obika, M. Tomizu, Y. Negoro, A. Orita, O. Nakagawa, and T. Imanishi, ChemBioChem., 2007, 8, 1924. CrossRef
96. H. Torigoe, Y. Hari, S. Obika, and T. Imanishi, Nucleosides Nucleotides Nucleic Acids, 2003, 22, 1571. CrossRef
97. H. Torigoe, Y. Hari, S. Obika, and T. Imanishi, Nucleosides Nucleotides Nucleic Acids, 2003, 22, 1097. CrossRef
98. S. Obika, Y. Hari, K. Morio, and T. Imanishi, Tetrahedron Lett., 2000, 41, 215. CrossRef
99. Y. Hari, S. Obika, M. Sakaki, K. Morio, Y. Yamagata, and T. Imanishi, Tetrahedron, 2002, 58, 3051. CrossRef
100. H. Staudinger and J. Meyer, Helv. Chim. Acta, 1919, 2, 619. CrossRef
101. V. K. Rajwanshi, R. Kumar, M. K. Hansen, and J. Wengel, J. Chem. Soc., Perkin Trans. 1, 1999, 1407. CrossRef
102. N. C. Bar, A. Roy, B. Achari, and S. B. Mandal, J. Org. Chem., 1997, 62, 8948. CrossRef
103. C. Wahlestedt, P. Salmi, L. Good, J. Kela, T. Johnsson, T. Hokfelt, C. Broberger, F. Porreca, J. Lai, K. Ren, M. Ossipov, A. Koshkin, N. Jakobsen, J. Skouv, H. Oerum, M. H. Jacobsen, and J. Wengel, Proc. Natl. Acad. Sci. USA, 2000, 97, 5633. CrossRef
104. J. Kurreck, E. Wyszko, C. Gillen, and V. A. Erdmann, Nucleic Acids Res., 2002, 30, 1911. CrossRef
105. R. Crinelli, M. Bianchi, L. Gentilini, and M. Magnani, Nucleic Acids Res., 2002, 30, 2435. CrossRef
106. S. Obika, M. Tomizu, Y. Negoro, T. Osaki, A. Orita, Y. Ueyama, O. Nakagawa, and T. Imanishi, Nucleosides Nucleotides Nucleic Acids, 2007, 26, 893. CrossRef
107. M. Koizumi, K. Morita, M. Daigo, S. Tsutsumi, K. Abe, S. Obika, and T. Imanishi, Nucleic Acids Res., 2003, 31, 3267. CrossRef
108. Y. Kasahara, S. Kitadume, K. Morihiro, M. Kuwahara, H. Ozaki, H. Sawai, T. Imanishi, and S. Obika, Bioorg. Med. Chem. Lett., 2010, 20, in press. CrossRef
109. M. Kuwahara, S. Obika, H. Takeshima, Y. Hagiwara, J. Nagashima, H. Ozaki, H. Sawai, and T. Imanishi, Med. Chem. Lett., 2009, 19, 2941. CrossRef
110. M. Kuwahara, S. Obika, J. Nagashima, Y. Ohta, Y. Suto, H. Ozaki, H. Sawai, and T. Imanishi, Nucleic Acids Res., 2008, 36, 4257. CrossRef