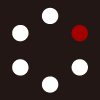
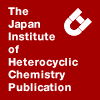
HETEROCYCLES
An International Journal for Reviews and Communications in Heterocyclic ChemistryWeb Edition ISSN: 1881-0942
Published online by The Japan Institute of Heterocyclic Chemistry
e-Journal
Full Text HTML
Received, 21st April, 2010, Accepted, 31st May, 2010, Published online, 2nd June, 2010.
DOI: 10.3987/COM-10-S(E)22
■ Asymmetric Syntheses of Highly Functionalized Bicyclo[2.2.2]octene Derivatives
Masafumi Iwatsu, Daisuke Urabe, and Masayuki Inoue*
Graduate School of Pharmaceutical Sciences, University of Tokyo, 7-3-1 Hongo, Bunkyo-ku, Tokyo 113-0033, Japan
Abstract
Highly functionalized bicyclo[2.2.2]octene derivatives bearing two quaternary carbon centers were synthesized in an asymmetric fashion. The key transformations were the regioselective Diels-Alder reaction between 3,6-dimethyl-o-quinone monoacetal and 1,1-diethoxyethylene and the subsequent enzymatic resolution. The optically active bicyclo[2.2.2]octene derivatives obtained should serve as versatile chiral building blocks for highly functionalized natural products such as ryanodine.INTRODUCTION
Many natural terpenoids possess complex carbocycles decorated by various oxygen functionalities and alkyl appendages. Among the diverse structural units found in terpenoids, quaternary carbon centers pose a particular challenge to synthetic organic chemists. The challenge is exacerbated further when multiple quaternary stereocenters are present within the complex carbocycles.1 Recently, we reported the synthesis of racemic C2-symmetric bicyclo[2.2.2]octene (±)-1, which bears two quaternary stereocenters (Scheme 1).2 The diketone functionalities of (±)-1 were then utilized as the chemical handles for its derivatization into (±)-2, which directly corresponds to the tricyclic skeleton of the pentacyclic sesquiterpenoid, ryanodine.3 This synthesis demonstrated the utility of the bicyclo[2.2.2]octene (±)-1 as an intermediate for construction of complex carboskeletons with multiple quaternary carbons.
Aside from the ryanodine structure, the 1,4-syn-oriented dimethyl groups of (±)-1 at the quaternary centers are structural units of various terpenoids, such as 3-hydroxy-15-rippertene,4 cyanthiwigin F,5 and correolide (Figure 1).6 Therefore, compound 1 and related highly functionalized bicyclo[2.2.2]octene derivatives should act as versatile building blocks for diverse polycyclic natural products. In this context, we decided to establish an asymmetric synthetic route to 1 and its analogous structures.
RESULTS AND DISCUSSION
Enantiopure (+)-1 was planned to be synthesized through enzymatic resolution of racemic compounds (Scheme 2).7 To do so, the secondary hydroxy and acetoxy groups were retrosynthetically introduced to the bicyclo[2.2.2]octene structure for the enzymatic acetylation and deacetylation, respectively. In the synthetic direction, the resolution of the racemic compounds (±)-3, (±)-4, (±)-5 or (±)-6 would generate the corresponding enantiomerically pure acetate or alcohol, which were to be converted into (+)-1. The two quaternary stereocenters of bicycles (±)-3-6 would be in turn constructed using the regioselective Diels-Alder reaction in a single step. Specifically, the ketene equivalent and 3,6-dimethyl-o-quinone monoacetal 7 were used as the dienophile and diene, respectively, to establish the bicyclic structure.8 Compound 7 with two sp2-connected methyl groups would be synthesized from 2,5-dimethylphenol 8 via oxidative dearomatization.9
As shown in Scheme 3, 3,6-dimethyl-o-quinone monoacetal 7 was synthesized from 2,5-dimethylphenol 8 in four steps. Ortho-selective formylation of 810 and subsequent Dakin reaction afforded catechol 911 in 99% yield. Monoetherification of 9 with ethylene carbonate in the presence of n-Bu4NI,12 followed by oxidative intramolecular acetalization of 10 using PhI(OAc)2, furnished cyclic acetal 7 in 42% yield (two steps).
Despite the general tendency toward facile dimerization through self Diels-Alder reaction of o-quinone monoacetals,8 3,6-dimethyl-o-quinone monoacetal 7 was remarkably stable even above 100 °C. Most importantly, diene 7 smoothly underwent Diels-Alder reaction with the electron-rich ketene equivalent, 1,1-ethoxyethylene, at 150 °C to introduce the two quaternary stereocenters. The reaction resulted in formation of bicyclo[2.2.2]octene (±)-11 as the sole product in 95% yield.
Both the exceptional stability and the exclusive regioselectivity in the cycloaddition should arise from the unique structural features of 7 (Figure 2). The bulky ketal and the two methyl groups prevent the dimerization pathway via TS-A, while the serious steric repulsion between the ketal and the ethoxy group of 1,1-diethoxyethylene inhibits the Diels-Alder reaction via the transition state TS-C. Consequently, TS-B is favored over the other two transition states, leading to predominant formation of (±)-11.
Thus obtained (±)-11 was converted to the four substrates (±)-3, (±)-4, (±)-5 and (±)-6 for the enzymatic resolution (Scheme 3). Reduction of (±)-11 with DIBAL-H, followed by treatment with aqueous 1M HCl, gave rise to (±)-3 in 77% yield (two steps) through chemoselective removal of the diethyl acetal. The stereochemistry of the resultant alcohol of (±)-3 was determined by NOE experiments. Alternatively, stepwise reaction with DIBAL-H then aqueous sulfuric acid transformed bis-acetal (±)-11 into diketone (±)-4 in 73% yield over two steps. Further one-step conversion from (±)-3 and (±)-4 gave acetylated derivatives (±)-5 (100%) and (±)-6 (97%), respectively.
Next, the four racemic bicyclo[2.2.2]octene derivatives were subjected to either enzymatic acetylation or deacetylation in order to deliver the corresponding enantiopure compounds.13 Both alcohols (±)-3 and (±)-4 failed to react with acetylation agents under the various enzymatic conditions, presumably because the hydroxy groups of (±)-3 and (±)-4 were hindered by the sterically cumbersome bicyclic structures. In fact, the acetates (±)-5 and (±)-6, the reacting carbonyls of which are more distant from the bicycles than the hydroxys, had higher reactivity toward the enzymes. Compounds (±)-5 and (±)-6 were smoothly hydrolyzed into alcohols (+)-4 (13% ee, entry 1) and (+)-3 (58% ee, entry 2), respectively, by the action of pig liver esterase (PLE) in pH 7 buffer.14 To improve the enantioselectivity, the co-organic solvent was screened by employing the more promising substrate (±)-5 (entries 3-5). Consequently, addition of isopropyl ether (entry 5) to the reaction media was demonstrated to have beneficial effect in comparison to dimethyl sulfoxide (entry 3) or tert-butanol (entry 4), resulting in formation of (+)-3 of 93% ee in 26% yield.15 After further optimization of temperature and pH, we found the resolution of (±)-5 was best performed in a mixture of pH 8 buffer and isopropyl ether at 30 °C to afford optically active (+)-3 (41% yield and 84% ee, entry 6) and (–)-5 (52% yield and 82% ee).16 Recrystallization of (+)-3 from hexane and ethyl acetate increased its optical purity to 96% ee (78% yield). As shown in Figure 3, the absolute structure of (+)-3 was unambiguously determined by X-ray crystallographic analysis of the (R)-MTPA ester derivative 14.
The enantioselectivity of the enzymatic reaction from (±)-5 was remarkably higher than that from (±)-6 (see entries 1 and 2, Table 1). These distinct outcomes should originate from the steric difference between the C2’-cyclic acetal and the C2’-ketone of 5 and 6, respectively (Figure 4). Namely, the reactivities of (+)-5 and (–)-5 toward the enzyme were effectively discriminated by the steric effect of the bulky C2’-substituted acetal, and consequently hydrolysis of (+)-5 was favored over that of (–)-5. On the other hand, the same enzyme hydrolyzed both (+)-6 and (–)-6 non-selectively, due to the similar sizes of the pseudo-equatorially oriented ketone and methyl groups, leading to lower enantiomeric excess of the generated (+)-4.
The obtained enantiopure (+)-3 was successfully converted to the optically active C2-symmetric bicyclo[2.2.2]octene 1, the intermediate for the synthetic study of ryanodine (Scheme 4). Hydrolysis of the ketal in alcohol (+)-3 (96% ee) under acidic conditions provided diketone (+)-4 in 95% yield. Dehydroxylation of diketone (+)-4 was accomplished by a two-step sequence. Chloromesylation of the hydroxy group converted (+)-4 into (+)-15 in 94% yield. Finally, treatment of α-chloromesylated ketone (+)-15 with NaI17 in the presence of TsOH gave rise to C2-symmetric bicyclo[2.2.2]octene 1 (62% yield) through α-iodoketone formation and subsequent reductive cleavage of the carbon-iodine bond by nucleophilic attack of iodide.18
In summary, we established an asymmetric synthetic route to highly functinalized bicyclo[2.2.2]octene derivatives bearing two quaternary carbon centers. The regioselective Diels-Alder reaction of the 3,6-dimethyl-o-quinone monoacetal to install the 1,4-syn-oriented methyl groups, and the subsequent enzymatic resolution were the two key reactions to produce bicyclo[2.2.2]octene derivatives (+)-3 and (–)-5 in a highly enantioselective fashion. Additionally, the optically active C2-symmetric bicyclo[2.2.2]octene (+)-1, an intermediate for our synthetic study of ryanodine, was obtained from (+)-3 in three steps. Studies toward an asymmetric total synthesis of ryanodine and other complex natural products from these bicyclo[2.2.2]octene derivatives are currently underway in our laboratory.
EXPERIMENTAL
All reactions sensitive to air or moisture were carried out under argon or atmosphere in dry or freshly distilled solvents under anhydrous conditions, unless otherwise noted. All other reagents were used as supplied unless otherwise noted. Analytical thin-layer chromatography (TLC) was performed using E. Merck Silica gel 60 F254 pre-coated plates. Column chromatography was performed using 100-210 μm Silica Gel 60N (Kanto Chemical Co., Inc.) and flash column chromatography was 50-60 μm Silica Gel 60 (Kanto Chemical Co., Inc.). 1H and 13C NMR spectra were recorded on a JEOL JNM-ECX-500, JNM-ECA-500, or a JNM-ECS-400 spectrometer. Chemical shifts were reported in ppm on the δ scale relative to residual CHCl3 (δ = 7.26 for 1H NMR and δ = 77.0 for 13C NMR) as an internal reference. Signal patterns are indicated as s, singlet; d, doublet; m, multiple. Infrared (IR) spectra were recorded on a Perkin Elmer Spectrum BX FT-IR spectrometer, or a JASCO FT/IR-4100 spectrometer. High resolution mass spectra were measured on a BRUKER DALTONICS APEX III (ESI), a BRUKER DALTONICS BioTOF-Q (ESI), a Shimadzu Biotec Axima-TOF2 (MALDI), or a JEOL JMS-700 Mstation (FAB). Optical rotations were recorded on a JASCO DIP-370 Polarimeter or a JASCO DIP-1000 Digital Polarimeter. Melting points were measured on a Yanaco MP-S3 micro melting point apparatus or a Yanaco MP-J3 micro melting point apparatus, and are uncorrected.
Cathecol 9.11 A mixture of 2,5-dimethyl phenol 8 (5.00 g, 40.9 mmol), anhydrous MgCl2 (5.85 g, 61.4 mmol), and Et3N (17 mL, 0.12 mol) in MeCN (27 mL) was stirred for 15 min at room temperature, and then paraformaldehyde (3.69 g, 0.12 mol) was added to the mixture. After being stirred for 2.5 h at 100 °C, the mixture was cooled to room temperature, and diluted with Et2O (40 mL) and 1M HCl (40 mL). After the resulting organic layer was separated, the aqueous layer was extracted with Et2O (40 mL x 2). Combined organic layers were dried over anhydrous Na2SO4, filtered, and concentrated to give the crude aldehyde, which was used to the next reaction without further purification.
To a solution of the crude aldehyde in THF (17 mL) and H2O (3 mL) was added 5M aqueous NaOH solution (8.1 mL) and 30% aqueous H2O2 solution (4.2 mL) at 0 °C. After being stirred at room temperature for 20 min, the reaction mixture was diluted with Et2O (20 mL) and 2N HCl (40 mL). After the resulting organic layer was separated, the aqueous layer was extracted with Et2O (20 mL x 3). Combined organic layers were dried over anhydrous Na2SO4, filtered, and concentrated. The residue was purified by column chromatography (silica gel 50 g, hexane/AcOEt 50:1 to 10:1) to afford cathecol 9 (5.58 g, 40.4 mmol) in 99% yield over 2 steps.
Alcohol 10. A mixture of cathecol 9 (1.27 g, 9.19 mmol), ethylene carbonate (850 mg, 9.65 mmol), and n-Bu4NI (167 mg, 0.45 mmol) was stirred for 7.5 h at 150 °C. Additional ethylene carbonate (81 mg, 0.92 mmol) and n-Bu4NI (167 mg, 0.45 mmol) were added to the solution. After 2.5 h at 150 °C, additional ethylene carbonate (40 mg, 0.45 mmol) and n-Bu4NI (83 mg, 0.22 mmol) were added to the solution. After being stirred for 2 h at 150 °C, the reaction mixture was cooled to room temperature, and diluted with AcOEt (10 mL). The resulting mixture was directly subjected to column chromatography (silica gel 20 g, hexane/AcOEt 25:1 to 10:1) to afford alcohol 10 (953 mg, 5.23 mmol) in 57% yield: dark red oil; IR (neat) ν 3447, 2995, 1770, 1759, 1374, 1246, 1059, 913, 744 cm–1; 1H NMR (500 MHz, CDCl3) δ 2.22 (3H, s, CH3), 2.26 (3H, s, CH3), 3.95-3.99 (2H, m, OCH2CH2O), 4.01-4.04 (2H, m, OCH2CH2O), 6.57 (1H, d, J = 7.8 Hz, CHA=CHB), 6.79 (1H, d, J = 7.8 Hz, CHA=CHB), 7.46 (1H, s, OH); 13C NMR (125 MHz, CDCl3) δ 15.6, 15.8, 61.9, 74.1, 120.7, 123.90, 126.1, 128.4, 144.3, 147.7; HRMS (MALDI) calcd for C10H14O3 182.0937 (M+), found 182.0944.
3,6-Dimethyl-o-quinone monoacetal 7. To a solution of alcohol 10 (1.43 g, 7.85 mmol) in (CF3)2CHOH (5.2 mL) was added PhI(OAc)2 (2.65 g, 8.23 mmol) at room temperature. After being stirred for 20 min at room temperature, the reaction mixture was concentrated. The residue was purified by column chromatography (silica gel 35 g, hexane/AcOEt 1:0 to 30:1) to afford 3,6-dimethyl-o-quinone monoacetal 7 (1.02 g, 5.66 mmol) in 72% yield: yellow oil; IR (neat) ν 2974, 2898, 1675, 1657, 1450, 1382, 1180, 1066, 1021, 980, 951 cm–1; 1H NMR (500 MHz, CDCl3) δ 1.80 (3H, s, CH3), 1.83 (3H, s, CH3), 4.13-4.18 (2H, m, OCH2CH2O), 4.36-4.40 (2H, m, OCH2CH2O), 5.95 (1H, d, J = 6.4 Hz, CHA=CHB), 6.58 (1H, d, J = 6.3 Hz, CHA=CHB); 13C NMR (125 MHz, CDCl3) δ 14.5, 16.5, 55.4, 66.0, 98.8, 123.2, 130.3, 137.1, 144.7, 200.3; HRMS (ESI) calcd for C10H13O3 181.0859 (M+H+), found 181.0858.
Diels-Alder adduct (±)-11. A solution of 3,6-dimethyl-o-quinone monoacetal 7 (1.02 g, 5.66 mmol) and ketene diethyl acetal (1.5 mL, 11 mmol) in toluene (1.1 mL) was stirred at 150 °C for 17 h. The reaction mixture was cooled to room temperature, and concentrated. The residue was purified by column chromatography (silica gel 25 g, hexane/AcOEt 50:1 to 10:1) to afford ketone (±)-11 (1.60 g, 5.40 mmol) in 95% yield: colorless oil; IR (neat) ν 2977, 2936, 2896, 1741, 1453, 1133, 1074, 1046, 1025, 951; 1H NMR (500 MHz, CDCl3) δ 1.11-1.16 (6H, m, OCH2CH3 x 2), 1.14 (3H, s, CH3), 1.34 (3H, s, CH3), 1.70 (1H, d, J = 13.7 Hz, C-CHAHB), 2.27 (1H, d, J = 13.7 Hz, C-CHAHB), 3.49-3.58 (4H, m, OCH2CH3 x 2), 4.00-4.07 (2H, m, OCH2CH2O), 4.29-4.36 (2H, m, OCH2CH2O), 5.67 (1H, d, J = 8.3 Hz, CHA=CHB), 6.13 (1H, d, J = 8.3 Hz, CHA=CHB); 13C NMR (125 MHz, CDCl3) δ 12.2, 15.5, 15.6, 16.3, 41.7, 41.9, 58.2, 58.3, 60.0, 66.5, 66.7, 101.8, 104.8, 132.4, 139.4, 205.5; HRMS (ESI), calcd for C16H24O5Na 319.1516 (M+Na+), found 319.1514.
Alcohol (±)-3. To a solution of ketone (±)-11 (10.8 g, 36.4 mmol) in CH2Cl2 (37 mL) was added DIBAL-H (1.0 M in hexane, 66 mL, 66 mmol) at -90 °C. After 20 min, additional DIBAL-H (3.3 mL, 3.3 mmol) was introduced to the mixture. After being stirred at -78 °C for 5 min, the reaction mixture was diluted with AcOEt (70 mL), sat Rochelle’s salt solution (40 mL) and saturated aqueous NH4Cl solution (40 mL). After the organic layer was separated, the aqueous layer was extracted with AcOEt (80 mL x3). Combined organic layers were dried over anhydrous Na2SO4, filtered, and concentrated to give the crude alcohol, which was used to the next reaction without further purification.
A solution of the crude alcohol in THF (33 mL) and 1M HCl (3.7 mL) was stirred at room temperature for 12 h, and the reaction was quenched with saturated aqueous NaHCO3 solution (50 mL). The resulting solution was extracted with AcOEt (50 mL x 3) and combined organic layers were washed with brine (150 mL), dried over anhydrous Na2SO4, filtered, and concentrated. The residue was purified by column chromatography (silica gel 50 g, hexane/AcOEt 10:1 to 3:1) to afford alcohol (±)-3 (6.31 g, 28.1 mmol) in 77% yield: colorless crystals; mp 72 °C (recrystallized from hexane/AcOEt); IR (neat) ν 3481, 2969, 2931, 2892, 1727, 1231, 1165, 1114, 1065, 953 cm–1; 1H NMR (400 MHz, CDCl3) δ 1.20 (3H, s, CH3), 1.33 (3H, s, CH3), 1.93 (1H, d, J = 18.8 Hz, C-CHAHB), 2.29 (1H, d, J = 18.8 Hz, C-CHAHB), 3.37 (1H, d, J = 4.6 Hz, OH), 3.38 (1H, d, J = 4.6 Hz, CH-OH), 3.90-4.00 (2H, m, OCH2CH2O), 4.07-4.14 (2H, m, OCH2CH2O), 5.76 (1H, d, J = 8.2 Hz, CHA=CHB), 6.16 (1H, d, J = 8.2 Hz, CHA=CHB); 13C NMR (100 MHz, CDCl3) δ 14.4, 16.5, 41.3, 43.8, 57.1, 64.9, 67.0, 78.7, 110.8, 131.8, 139.9, 209.9; HRMS (ESI), calcd for C12H16O4Na 247.0941 (M+Na+), found 247.0940.
Acetate (±)-5. A mixture of alcohol (±)-3 (3.01 g, 13.4 mmol), Ac2O (2.3 mL, 24 mmol), pyridine (2.3 mL, 28 mmol), and DMAP (327 mg, 2.68 mmol) in CH2Cl2 (6.7 mL) was stirred at room temperature for 12 h. The reaction was quenched with saturated aqueous NH4Cl solution (10 mL). The resulting mixture was extracted with AcOEt (10 mL x 2), and combined organic layers were washed with brine (20 mL), dried over anhydrous Na2SO4, filtered, and concentrated. The residue was purified by column chromatography (silica gel 15 g, hexane/AcOEt 20:1 to 7.5:1) to afford acetate (±)-5 (3.56 g, 13.4 mmol) in 100% yield: colorless crystals; mp 89 °C (recrystallized from hexane/AcOEt); IR (neat) ν 2974, 2934, 2898, 1733, 1372, 1249, 1225, 1116, 1021, 956 cm–1; 1H NMR (500 MHz, CDCl3) δ 1.16 (3H, s, CH3), 1.22 (3H, s, CH3), 1.93 (1H, d, J = 18.9 Hz, C-CHAHB), 2.06 (3H, s, Ac), 2.38 (1H, d, J = 18.9 Hz, C-CHAHB), 3.78-4.05 (2H, m, OCH2CH2O), 4.00-4.11 (2H, m, OCH2CH2O), 4.54 (1H, s, CH-OAc), 5.74 (1H, d, J = 8.0 Hz, CHA=CHB), 6.20 (1H, d, J = 8.0 Hz, CHA=CHB); 13C NMR (125 MHz, CDCl3) δ 14.5, 16.3, 20.8, 40.5, 44.6, 54.2, 64.9, 67.5, 78.9, 110.0, 129.9, 141.4, 170.5, 208.3; HRMS(ESI), calcd for C14H18O5Na 289.1046 (M+Na+), found 289.1045.
Hydroxy ketone (±)-4. To a solution of ketone (±)-11 (423 mg, 1.43 mmol) in CH2Cl2 (2.8 mL) was added DIBAL-H (1.0 M in hexane, 4.3 mL, 4.3 mmol) at -78 °C. After 1 h at -60 °C, additional DIBAL-H (1.4 mL, 1.4 mmol) was introduced to the mixture. After being stirred at -60 °C for 1 h, additional DIBAL-H (0.7 mL, 0.7 mmol) was added to the mixture. After being stirred at -40 °C for 30 min, the reaction mixture was diluted with AcOEt (10 mL), sat Rochelle’s salt solution (10 mL) and saturated aqueous NH4Cl solution (10 mL). After the organic layer was separated, the aqueous layer was extracted with AcOEt (10 mL x3). Combined organic layers were dried over anhydrous Na2SO4, filtered, and concentrated to give the crude alcohol, which was used to the next reaction without further purification.
A solution of the crude alcohol in a mixture of THF (0.9 mL), H2SO4 (3.7 mL), and H2O (2.3 mL) was stirred at 50 °C for 26 h. Then, the mixture was washed with saturated aqueous NaHCO3 solution (10 mL x 2), dried over anhydrous Na2SO4, filtered, and concentrated. The residue was purified by column chromatography (silica gel 10 g, hexane/AcOEt 10:1 to 5:1 to 1:1) to afford alcohol hydroxy ketone (±)-4 (188 mg, 1.04 mmol) in 73% yield.
Acetate (±)-6. A mixture of hydroxy ketone (±)-4 (75 mg, 0.42 mmol), pyridine (34 µL, 0.42 mmol) and DMAP (10 mg, 83 µmol) in CH2Cl2 (1.0 mL) was stirred at room temperature for 1.5 h, and saturated aqueous NH4Cl solution (5 mL) was added to the mixture. After separation, the aqueous layer was extracted with AcOEt (5 mL x 2), and combined organic layers were washed with brine (10 mL), dried over anhydrous Na2SO4, filtered, and concentrated. The residue was purified by column chromatography (silica gel 5 g, hexane/AcOEt 20:1 to 10:1) to afford acetate (±)-6 (90 mg, 0.40 mmol) in 97% yield: IR (neat) ν 2978, 2936, 1757, 1735, 1371, 1218, 1121, 1054, 1027 cm–1; 1H NMR (400 MHz, CDCl3) δ 1.25 (3H, s, CH3), 1.33 (3H, s, CH3), 2.10 (3H, s, Ac) 2.31 (1H, d, J = 18.6 Hz, C-CHAHB), 2.36 (1H, d, J = 18.6 Hz, C-CHAHB), 5.13 (1H, s, CH-OAc), 6.08 (1H, d, J = 8.2 Hz, CHA=CHB), 6.22 (1H, d, J = 8.2 Hz, CHA=CHB); 13C NMR (125 MHz, CDCl3) δ 13.4, 16.2, 20.4, 42.1, 49.5, 55.7, 71.3, 134.5, 137.7, 169.7, 203.1, 205.8; HRMS(FAB), calcd for C12H15O4 223.0970 (M+H+), found 223.0978.
Enzymatic deacetylation of acetate (±)-5. A solution of acetate (±)-5 (5.04 g, 18.9 mmol) and PLE (5.04 g, 27 units/mg) in i-Pr2O (38 mL) and pH 8 phosphate buffer (0.1 M, 150 mL) was stirred at 30 °C for 24 h and extracted with Et2O (150 mL x 15). Combined organic layers were dried over anhydrous Na2SO4, filtered and concentrated. The residue was purified by column chromatography (silica gel 60 g, hexane/AcOEt 11:1 to 1.5:1) to afford alcohol (+)-3 (1.72 g, 7.67 mmol, 84% ee) and acetate (–)-5 (2.61 g, 9.80 mmol, 82% ee, [α]D22 –278.8, c 1.05, CHCl3) in 41% and 52% yield, respectively. Recrystallization of (+)-3 from AcOEt/hexane (2:1, at 35-22 °C) improved its optical purity to 96% ee (1.35 g, 6.02 mmol, 78% yield): [α]D22 407 (c 1.00, CHCl3).
(R)-MTPA ester 14. A mixture of alcohol (+)-3 (12 mg, 0.054 mmol), (S)-MTPACl (15 μL, 0.082 mmol), and DMAP (3 mg, 0.02 mmol), pyridine (26 µL, 0.163 mmol) in CH2Cl2 (0.3 mL) was stirred at 50 °C for 16 h, and additional (S)-MTPACl (15 µL, 0.082 mmol) and DMAP (3 mg, 0.02 mmol) was added to the mixture. The reaction mixture was stirred at 50 °C for 11 h, and then quenched with saturated aqueous NH4Cl solution (3 mL). The resulting mixture was extracted with Et2O (3 mL x 3), and combined organic layers were washed with brine (10 mL), dried over anhydrous Na2SO4, filtered, and concentrated. The residue was purified by flash column chromatography (silica gel 1 g, hexane/AcOEt 60:1 to 15:1) to afford (R)-MTPA ester 14 (18 mg, 0.041 mmol) in 76% yield: colorless crystals; mp 139-140 °C; 1H NMR (500 MHz, CDCl3) δ 1.12 (3H, s, CH3), 1.16 (3H, s, CH3), 1.91 (1H, d, J = 18.8 Hz, C-CHAHB), 2.30 (1H, d, J = 18.8 Hz, C-CHAHB), 3.46 (3H, s, OCH3), 3.77 (1H, ddd, J = 10.1, 6.9, 6.7 Hz, OCHAHBCH2O), 3.87 (1H, ddd, J = 6.9, 6.4, 2.3 Hz, OCH2CHAHBO), 4.08 (1H, ddd, J = 8.2, 6.7, 2.3 Hz, OCH2CHAHBO), 4.16 (1H, ddd, J = 10.1, 8.2, 6.4 Hz, OCHAHBCH2O), 4.63 (1H, s, CH-OCO-), 5.71 (1H, d, J = 8.0 Hz, CHA=CHB), 6.21 (1H, d, J = 8.0 Hz, CHA=CHB), 7.39 (3H, m, Ph), 7.53-7.57 (2H, m, Ph).
Crystallographic data of 14.19
C22H23F3O6, M = 440.414, monoclinic, space group P21, a = 10.891(3) Å, b = 12.273(4) Å, c = 8.517(3) Å, α = 90.00°, β = 110.54(2)°, γ = 90.00°, V = 1066.1(6) Å3, density 1.372 g·cm-1, Z = 2, R value = 0.013.
Hydroxy ketone (+)-4. A solution of alcohol (+)-3 (2.74 g, 12.2 mmol) in a mixture of THF (5.5 mL), H2SO4 (1.1 mL), and H2O (5.5 mL) was stirred at 50 °C for 20 h. Then, AcOEt (10 mL) and saturated aqueous NaHCO3 solution (10 mL) were added at 0 °C. After the organic layer was separated, the aqueous layer was extracted with AcOEt (10 mL x 6). Combined organic layers were washed with brine (70 mL), dried over anhydrous Na2SO4, filtered, and concentrated. The residue was purified by column chromatography (silica gel 25 g, hexane/AcOEt 10:1 to 6:1) to afford hydroxy ketone (+)-4 (2.11 g, 11.7 mmol) in 96% yield: colorless oil; [α]D25 787 (c 0.98, CHCl3); IR (neat) ν 3447, 2974, 2934, 1730, 1454, 1123, 1064, 1026, 817, 774, 683 cm-1; 1H NMR (500 MHz, CDCl3) δ 1.34 (3H, s, CH3), 1.41 (3H, s, CH3), 2.22 (1H, d, J = 18.5 Hz, C-CHAHB), 2.31 (1H, d, J = 18.5 Hz, C-CHAHB), 2.83 (1H, d, J = 2.0 Hz, OH), 3.67 (1H, d, J = 2.0 Hz, CH-OH), 6.03 (1H, d, J = 7.9 Hz, CHA=CHB), 6.17 (1H, d, J = 7.9 Hz, CHA=CHB); 13C NMR (125 MHz, CDCl3) δ 13.6, 16.1, 42.3, 49.1, 56.7, 72.8, 135.0, 136.9, 206.8, 208.8.; HRMS(ESI) calcd for C10H12O3Na 203.0679 (M+Na+), found 203.0678.
Chloromesylate (+)-15. To a solution of hydroxy ketone (+)-4 (1.37 g, 7.60 mmol) and Et3N (1.8 mL, 13 mmol) in CH2Cl2 (15 mL) was added ClCH2SO2Cl (1.0 mL, 11 mmol) at 0 °C. After being stirred at room temperature for 2 h, the reaction mixture was quenched with saturated aqueous NH4Cl solution (20 mL). The resulting mixture was extracted with AcOEt (20 mL x 2). Combined organic layers were washed with brine (50 mL), dried over anhydrous Na2SO4, filtered, and concentrated. The residue was purified by column chromatography (silica gel 20 g, hexane/AcOEt 10:1 to 4:1) to afford chloromesylate (+)-15 (2.10 g, 7.17 mmol) in 94% yield: 1H NMR (500 MHz, CDCl3) δ 1.37 (3H, s, CH3), 1.44 (3H, s, CH3), 2.32 (1H, d, J = 18.9 Hz, C-CHAHB), 2.41 (1H, d, J = 18.9 Hz, C-CHAHB), 4.71 (1H, s, CH-OSO2CH2Cl), 4.80 (1H, d, J = 12.6 Hz, CHACHBCl), 4.89 (1H, d, J = 12.6 Hz, CHACHBCl), 6.09 (1H, d, J = 8.0 Hz, CHA=CHB), 6.24 (1H, d, J = 8.0 Hz, CHA=CHB); 13C NMR (125 MHz, CDCl3) δ 13.4, 16.1, 41.5, 49.3, 55.3, 55.7, 79.7, 134.1, 137.9, 201.3, 203.4.
C2-Symmetric bicyclo[2.2.2]octene (+)-1. A solution of chloromesylate (+)-15 (2.10 g, 7.17 mmol) and NaI (5.40 g, 35.9 mmol) in acetone (48 mL) was stirred at 50 °C for 12 h, and then TsOH·H2O (1.4 g, 7.5 mmol) was added to the mixture. After being stirred for 1 h at 50 °C, the reaction mixture was quenched with saturated aqueous Na2S2O3 solution (20 mL). The resulting mixture was extracted with Et2O (20 mL x 2). Combined organic layers were washed with brine (50 mL), dried over anhydrous Na2SO4, filtered, and concentrated. The residue was purified by column chromatography (silica gel 15 g, hexane/Et2O 30:1 to 15:1) to afford C2-symmetric bicyclo[2.2.2]octene (+)-1 (731 mg, 4.45 mmol) in 62% yield: pale yellow solid; [α]D25 1006 (c 1.01, CHCl3); IR (neat) ν 2973, 2932, 1726, 1452, 1405, 1383, 1118, 774, 683 cm–1; 1H NMR (500 MHz, CDCl3) δ 1.30 (6H, s, CH3 x 2), 2.24 (2H, d, J = 18.9 Hz, C-CHAHB x 2), 2.28 (2H, d, J = 18.9 Hz, C-CHAHB x 2), 6.12 (2H, s, CH=C x 2); 13C NMR (125 MHz, CDCl3) δ 16.5, 42.0, 51.1, 137.1, 209.1; HRMS (ESI) calcd for C10H12O2Na 187.0729 (M+Na+), found 187.0736.
ACKNOWLEDGEMENTS
This work was supported by a Grant-in-Aid for Young Scientists (S) (JSPS) to M. I. We are grateful to Prof. Tohru Fukuyama and Dr. Satoshi Yokoshima of the University of Tokyo for performing X-ray crystallographic analysis.
References
1. For recent reviews on construction of quaternary carbon centers, see; J. Chirstoffers and A. Mann, Angew. Chem. Int. Ed., 2001, 40, 4591; CrossRef I. Denissova and L. Barriault, Tetrahedron, 2003, 59, 10105; CrossRef E. A. Peterson and L. E. Overman, Proc. Natl. Acad. U.S.A., 2004, 101, 11943; CrossRef D. Urabe and M. Inoue, Tetrahedron, 2009, 65, 6271; CrossRef M. Bella and T. Gasperi, Synthesis, 2009, 1583. CrossRef
2. K. Hagiwara, M. Himuro, M. Hirama, and M. Inoue, Tetrahedron Lett., 2009, 50, 1035. CrossRef
3. E. F. Rogers, F. R. Koniuszy, J. Shavel, Jr., and K. Folkers, J. Am. Chem. Soc., 1948, 70, 3086; CrossRef K. Wiesner, Z. Valenta, and J. A. Findlay, Tetrahedron Lett., 1967, 8, 221; CrossRef S. N. Srivastava and M. Przybylska, Can. J. Chem., 1968, 46, 795; CrossRef For total synthesis of (+)-ryanodol, see; A. Bélanger, D. J. F. Berney, H.-J. Borschberg, R. Brousseau, A. Doutheau, R. Durand, H. Katayama, R. Lapalme, D. M. Leturc, C.-C. Liao, F. N. MacLachlan, J.-P. Maffrand, F. Marazza, R. Martino, C. Moreau, L. Saint-Laurent, R. Saintonge, P. Soucy, L. Ruest, and P. Deslongchamps, Can. J. Chem., 1979, 57, 3348. CrossRef
4. G. D. Prestwich, S. G. Spanton, J. W. Lauher, and J. Vrkoč, J. Am. Chem. Soc., 1980, 102, 6825. CrossRef
5. J. Peng, K. Walsh, V. Weedman, J. D. Bergthold, J. Lynch, K. L. Lieu, I. A. Braude, M. Kelly, and M. T. Hamann, Tetrahedron, 2002, 58, 7809; CrossRef For total syntheses of cyanthiwigins, see; J. A. Enquist, Jr. and B. M. Stoltz, Nature, 2008, 453, 1228; CrossRef M. W. B. Pfeiffer and A. J. Philips, J. Am. Chem. Soc., 2005, 127, 5334. CrossRef
6. M. A. Goetz, O. D. Hensens, D. L. Zink, R. P. Borris, B. F. Moralesˆ, G. Tamayo-Castilloˆ, R. S. Slaughter, J. Felix, and R. G. Ball, Tetrahedron Lett., 1998, 39, 2895. CrossRef
7. M. Meltz and N. A. Saccomano, Tetrahedron Lett., 1992, 33, 1201; CrossRef F. A. Luzzio and D. Y. Duveau, Tetrahedron: Asymmetry, 2002, 13, 1173; CrossRef Y. Luo and A. J. Carnell, J. Org. Chem., 2010, 75, 2057. CrossRef
8. For recent syntheses of related bicyclo[2.2.2]octane structures using intermolecular Diels-Alder reactions, see; C.-S. Chu, T.-H. Lee, and C.-C. Liao, Synlett, 1994, 635; CrossRef O. Arjona, R. Medel, and J. Plumet, Tetrahedron Lett., 1999, 40, 8431; CrossRef C.-C. Liao, C.-S. Chu, T.-H. Lee, P. D. Rao, S. Ko, L.-D. Song, and H.-C. Shiao, J. Org. Chem., 1999, 64, 4102; CrossRef S.-Y. Gao, Y.-L. Lin, P. D. Rao, and C.-C. Liao, Synlett, 2000, 421; CrossRef S.-Y. Gao, S. Ko, Y.-L. Lin, R. K. Peddinti, and C.-C. Liao, Tetrahedron, 2001, 57, 297; CrossRef Y. L. Dory, A. Roy, P. Soucy, and P. Deslongchamps, Org. Lett., 2009, 11, 1197; CrossRef Z. Hua, L. Chen, Y. Mei, and Z. Jin, Tetrahedron Lett., 2009, 50, 6621. CrossRef
9. For reviews on o-quione monoacetal, see; C.-C. Liao and R. K. Peddinti, Acc. Chem. Res., 2002, 35, 856; CrossRef D. Magdziak, S. J. Meek, and T. R. R. Pettus, Chem. Rev., 2004, 104, 1383. CrossRef
10. P. D. Knight, G. Clarkson, M. L. Hammond, B. S. Kimberley, and P. J. Scott, Organomet. Chem., 2005, 690, 5125; CrossRef R. X. Wang, X. Z. You, Q. J. Meng, E. A. Mintz, and X. R. Bu, Synth. Commun., 1994, 24, 1757; CrossRef G. Gasnati, M. Crisafulli, and A. Ricoa, Tetrahedron Lett., 1965, 6, 243. CrossRef
11. A. Pezzella, L. Lista, A. Napolitano, and M. d’Ischia, Tetrahedron Lett., 2005, 46, 3541. CrossRef
12. W. W. Carlson and L. H. Cretcher, J. Am. Chem. Soc., 1947, 69, 1952; CrossRef K. Yamaguchi, S. Negi, S. Kozakai, R. Nagano, H. Kuboniwa, A. Hirano, S. Nakahara, and N. Yamazaki, Bull. Chem. Soc. Jpn., 1988, 61, 2047. CrossRef
13. For recent reviews on biocatalyzed transformations, see; T. Hudlicky and J. W. Reed, Chem. Soc. Rev., 2009, 38, 3117; CrossRef H. Akita, Heterocycles, 2009, 78, 1667. CrossRef
14. For a review, see; P. D. de María, C. A. García-Burgos, G. Bargeman, and R. W. van Gemert, Synthesis, 2007, 1439. CrossRef
15. L. K. P. Lam, R. A. H. F. Hui, and J. B. Jones; J. Org. Chem. 1986, 51, 2047; CrossRef F. Björkling, J. Boutelje, S. Gatenbeck, K. Hult, T. Norin, and P. Szmulik, Bioorg. Chem., 1986, 14, 176; CrossRef G. Guanti, L. Banfi, E. Narisano, R. Riva, and S. Thea, Tetrahedron Lett., 1986, 27, 4639; CrossRef G. Guanti, L. Banfi, and E. Narisano, Tetrahedron Lett., 1989, 30, 2697; CrossRef M. Polla and T. Frejd, Tetrahedron, 1991, 47, 5883; CrossRef P. D. de María, B. Kossmann, N. Potgrave, S. Buchholz, H. Trauthwein, O. May, and H. Gröger, Synlett, 2005, 1746. CrossRef
16. Recovered PLE was able to recycle at least three times without the loss of the enantioselectivity.
17. N. Murata, S. Fujimori, Y. Ichihara, Y. Sato, T. Yamaji, H. Tsuboi, M. Uchida, H. Suzuki, M. Yamada, T. Oikawa, H. Nemoto, J. Nobuhiro, T. Choshi, and S. Hibino, Chem. Pharm. Bull., 2006, 54, 1567. CrossRef
18. The deoxygenation from the corresponding mesylate was sluggish.
19. CCDC 764823 contains the supplementary crystallographic data for this paper. These data can be obtained free of charge from The Cambridge Crystallographic Data Centre via www.ccdc.cam.ac.uk/data_request/cif.