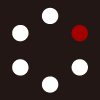
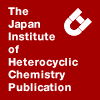
HETEROCYCLES
An International Journal for Reviews and Communications in Heterocyclic ChemistryWeb Edition ISSN: 1881-0942
Published online by The Japan Institute of Heterocyclic Chemistry
e-Journal
Full Text HTML
Received, 25th August, 2010, Accepted, 26th October, 2010, Published online, 16th November, 2010.
DOI: 10.3987/COM-10-S(E)113
■ Differentiated Cyclization of the Ketoacid Derived from Tokinolide B.
Alejandra León, Rubén A. Toscano, J. Antonio Cogordán, and Guillermo Delgado*
Institute of Chemistry, National Autonomous University of Mexico, University City, Coyoacan 04510, Mexico
Abstract
Treatment of the ketoacid derivative (5) of the bioactive natural dimeric phthalide tokinolide B under weakly basic conditions and pressure, produced the novel ketal compound 6 via addition of the carboxylate of 5 to the ketone, followed by Michael addition of the alcoholate (O-alkylation) and equilibration. On the other hand, treatment of 5 with strong base (NaOH, THF, MeOH) promoted C-alkylation, affording cyclotokinolide B (7). Relative theoretical energies indicated that derivatives 6 and 7 are energetically more stable than the starting material 5. The cytotoxicities of the natural product 3 and its analogues 5−7 against selected human cancer cells are reported.INTRODUCTION§
Phthalides are a small group of less than one hundred natural acetogenins that includes around twenty natural dimeric substances derived from [π2σ + π2σ] and [π4σ + π2σ] cycloadducts of Z-ligustilide (1) or structural analogues. These substances are found mainly in plants belonging to the family Umbelliferae (Apiaceae) used in traditional medicine in Asia, Europe, and North America.1 In Northern Mexico and the Southern United States Ligusticum porteri is considered one of the most important medicinal remedies in the region2 and is used for the treatment of stomachaches, tuberculosis, bronchitis, colds, pneumonia, and diabetes. Chemical analysis of this species allowed the isolation of 1, and the dimeric racemic compounds diligustilide (levistolide A) (2), tokinolide B (3) and riligustilide (4) (Scheme 1).3 The preparation of linear dimers from Z-ligustilide (1) has been reported,4 as well as the relay synthesis of 2−4 from the same monomer (1).5 Our earlier studies on the chemical reactivity of these intriguing racemic cycloadducts revealed the feasibility of intramolecular reactions to afford polycyclic compounds.5 In accordance with the diverse ethnomedicinal uses of plants containing dimeric phthalides, preliminary bioassays indicated that these substances displayed various biological activities.1,6−8
Our consideration of the limited predictive knowledge of the chemistry of dimeric phthalides, the experimental difficulties in obtaining derivatives, and the unknown potential of these types of substances as cytotoxic agents, led us to investigate the chemical reactivity of the ketoacid of tokinolide B (5), a derivative of the natural dimeric phthalide 3, in order to obtain derivatives that could be bioevaluated. Our results, reported herein, encompass (a) the characterization of an unexpected cyclization product from 5 whose preparation was compared with the formation of cyclotokinolide B (7), (b) the determination of the relative theoretical energies of the starting material and the obtained products, and (c) the evaluation of the cytotoxic potential of compounds 3, 5, 6, and 7 against three human tumor cell lines.
RESULTS AND DISCUSSION
Compound 5 was obtained in good yield by chemoselective lactone ring opening of the natural product 3 with base in acetone under reflux,9 but treatment of 5 with (R)-(+)- or (S)-(−)-α-methylbenzylamine at 0 °C or at room temperature using different solvents (iPrOH, EtOAc, CH2Cl2) did not afford products. The absence of the expected salts could be due to the steric hindrance of both the carboxylic acid and the amine, preventing the formation of the product. Therefore, we considered the possibility of obtaining diastereomeric amides. For this purpose, we undertook several attempts toward the preparation of the corresponding acyl chloride, which in turn could react directly with the chiral amine. However, treatment of 5 using SOCl2 with different solvents (CH2Cl2, THF) at different temperatures (–78 °C, 0 °C, room temp.) afforded only tokinolide B (3) as the sole product in good yields (Scheme 2).
We then proposed that direct reaction of the keto group of 5 with a chiral amine under pressure could afford the corresponding diastereomeric addition products. After extensive experimentation, however, we found that treatment of 5 with (S)-(−)-α-methylbenzylamine in toluene (or with (R)-(+)-α-methylbenzylamine or with (±)-α-methylbenzylamine) under 50 psi unexpectedly resulted in a mixture of the racemic ketal 6 and tokinolide B (3) in 42% and 8% yield, respectively, and 22% of starting material (Scheme 2). The transformation did not proceed in the absence of base.
The structure of the new compound 6 was determined according to the following spectroscopic evidence. The molecular formula (C24H30O5 established by HRMS) indicated that the product was isomeric with the starting material; the 13C NMR data for 6 showed 24 signals which were assigned (by DEPT analysis) to two lactonic carbonyls, six quaternary carbons, four methines, ten methylenes and two methyl groups. The disappearance of the 13C NMR signals assigned to the ketone group, the carboxylic group and the C(6)–C(7), C(3a)–C(7a) double bonds for 6, in comparison with the starting material, indicated that these groups were involved in the transformation. The new signals at δc 115.01, 129.75 and 141.58 corresponded to a ketalic carbon and a trisubstituted double bond (assignments by HSQC, HMBC, and NOESY experiments) respectively, establishing the structure 6 for the product, which was confirmed by X-ray analysis (Figure 1). The structure of the additional product (3) was secured by direct comparison with an authentic sample from our laboratory.
Complementary to this transformation, treatment of 5 with NaOH, THF, MeOH afforded cyclotokinolide B (7)9 (Scheme 3).
The suggested mechanistic routes for the formation of 3, 6 and 7 from 5 are shown in Scheme 4. The abstraction of the carboxylic proton by methylbenzylamine (R–NH2) afforded entity A (pathway a), promoting the nucleophilic attack on the C−3′ carbonyl group (via 5-exo-trigonal cyclization) to form the intermediate B. Michael addition of the oxyanion to the double bond C(3a)–C(7a) with a second 5-exo-trigonal cyclization (O-alkylation) and subsequent equilibration afforded the unexpected enone 6. The formation of 3 could be explained via protonation and dehydration of B. Therefore, there is competition between the formation of 3 and 6. Complementary, the transformation of 5 into 7 could be described via formation of the enolate which is C-alkylated (via 5-enolendo-exo-trigonal cyclization)10 to afford cyclotokinolide B (7) (Scheme 4, pathway b). These results clearly demonstrated that keto acid 5 is endowed with potential to generate polycyclic structures.
To determine the relative stabilities of 5, 6 and 7 (they are isomeric compounds), we conducted theoretical total energy calculations. For this purpose, each compound was modeled and once the structure was completed, global geometry optimization was computed. Molecular modeling procedures were made with GaussView.11 All molecular computations were performed at the B3LYP/6−311G level of theory using the suite of programs in Gaussian 03.12 The performance of the B3LYP functional over a set of organic molecules has been described.13 From the computed total energies we obtained ΔE (5−6) = 6.95 kcal/mol and ΔE (5−7) = 4.71 kcal/mol.
This theoretical analysis of total energies of the optimized structures indicated that compound 6 was energetically more stable than 7 by 2.24 kcal/mol. The stability of 6 could be attributed to the greater number of rings, in this case six rings, in contrast with 7 which incorporates five rings. 5 was found to be the energetically less stable compound, perhaps due to its tetracyclic nature and its inability to maintain conformationally rigid alkyl chains (Figure 2).
Since the cytotoxic potential of dimeric phthalides is not known, we tested the activities of the obtained compounds 3, 5, 6, and 7 against human leukemia (K562), colon (HCT-15), and lung (SKLU-1) cancer cell lines following standard protocols.14 The IC50 values (Table 1) indicated moderate selectivities toward certain cell lines, compared with the positive control (helenalin).
Natural product 3 exhibited moderate activity against the cell lines HCT-15 and SKLU-1, while 5 showed lack of cytotoxicity.
In conclusion, we have developed experimental conditions to afford different cyclization products from 5. Treatment with weak base and pressure afforded the unexpected ketal (6) via O-alkylation while treatment with strong base afforded cyclotokinolide B (7) via C-alkylation. The theoretical results indicated that 6 was the structure with relatively lower energy, followed by 7, and this outcome may be correlated with the number of rings and conformational constraints in the structures. These results are in agreement with previous observations suggesting that the intramolecular cyclizations of the dimeric phthalides are a general feature for these types of substances. Our demonstrations of these characteristics should extend our predictive capacity for the reactivity of dimeric phthalides. Compounds 3, 6, and 7 exhibited moderate cytotoxic activities toward the tested cell lines.
EXPERIMENTAL
GENERAL
Melting points were measured on a Fisher Johns apparatus and are uncorrected. Infrared spectra were recorder with FTIR Bruker TENSOR 27 instrument. Ultraviolet spectra were determinated on a Shimadzu UV160U Instrument. EISM and HRMS (FAB+) were recorded on a JEOL SX102A Mass Spectrometer. The 1H- and 13C-NMR experiments were performed at 25 ºC using Varian 500 spectrometer (at 500/125 MHz) and Bruker AVANCE (at 300/75 MHz), and the chemical shifts are expressed in parts per million (δ) relative to trimethylsilane. 1D and 2D homo- and heteronuclear NMR techniques, COSY, NOESY, gHMQC and gHMBC, were employed to elucidate the structure of 6. Analytical TLC was carried out using Merck aluminum silica gel 60 F254 plates and the visualization was accomplished with a UV lamp and a solution of ammonium cerium sulfate. Column chromatography was performed using silica gel 60 (230−400 meshes, Merck®). The solvent system is specified in each experiment. Tokinolide B (3) was isolated from the acetonic extract of the roots of Ligusticum porteri by successive column chromatographies.3
Treatment of 5 with (R)-(+)-α-Methylbenzylamine
5 (50.2 mg, 0.12 mmol) was dissolved in iPrOH (0.5 mL) at 55 ºC under nitrogen; the solution was cooled at room temperature. (R)-(+)-α-methylbenzylamine (16 μL, 0.12 mmol) was then added to the reaction mixture and kept from 12 to 96 h at room temperature at 0 °C. The same assays were carried out using different solvents (EtOAc, CH2Cl2, EtOH) and with (S)-(−)-α-methylbenzylamine. After usual work up, only the starting material was recovered.
Treatment of 5 with SOCl2
To a solution of 5 (91.1 mg, 0.22 mmol) in dry CH2Cl2 (3 mL) and dry pyridine (30 µL, 0.37 mmol) at –78 ºC was added SOCl2 (30 µL, 0.41 mmol) under nitrogen. The mixture was stirred for 2 h to reach 0 ºC. After this time, (R)-(+)-α-methylbenzylamine (50 µL, 0.45 mmol) was added and stirred for 2.5 h to reach room temperature. The reaction mixture was diluted with CH2Cl2 and usual work up allowed affording a yellow oil which was purified by column chromatography (n-hexane/EtOAc, gradient) to obtain compound 3 as the only reaction product (73.7 mg, 84%). The same results were obtained using THF instead of methylene chloride.
Preparation of compound 6
To a solution of ketoacid of tokinolide B (5, 36 mg, 0.095 mmol) in anhydrous toluene (10 mL) placed in a stainless steel reactor (100 mL) was added (S)-(−)-α-methylbenzylamine or (R)-(+)-α-methylbenzylamine or (±)-α-methylbenzylamine (0.02 mL, 0.15 mmol) under nitrogen atmosphere. The reactor was sealed and heated (130 °C) for 18 h (pressure: 50 psi). After cooling to room temperature, the reaction mixture was concentrated at reduced pressure. EtOAc was added and the organic phase was washed with HCl (10%, 3 × 5 mL), and aqueous phase was re-extracted with EtOAc (3 × 10 mL). The organic layers were combined, washed with brine, dried with Na2SO4 and concentrated under vacuum. The residue was purified by column chromatography (n-hexane/EtOAc, gradient) to obtain compound 3 (3 mg, 8% as the less polar compound), 6 (white crystals, 15.4 mg, 42%) and the starting material was recovered (8 mg, 22%). Using longer reaction times for the reaction favored the formation of additional minor products, while shorter reaction times lowered the yield of 3 and 6. Attempts to carry out the transformation without solvent afforded an insoluble dark brown residue. The starting material was recovered from assays carried out with the absence of base. Mp 204–206 °C; RF: (n-hexane/AcOEt, 4:1) 0.51; UV (MeOH) λmax nm (ε): 217 (29077); IR (CHCl3) νmax (cm-1): 3028, 2875, 1757, 1686, 1665, 1329, 1126, 1068, 1041, 1011, 955, 887; 1H NMR (500 MHz, CDCl3, assignments by COSY, HSQC, HMBC, NOESY) δ: 7.43 (d, J = 7.0 Hz, 1H, H−7′), 7.01 (dt, J = 7.0, 2.5 Hz, 1H, H−7), 3.04 (m, 1H, H−6′), 2.46 (m, 1H, H−6a), 2.40 (m, 1H, H−4a), 2.14 (m, 1H, H−6b), 2.01 (m, 1H, H−5a′), 1.85 (m, 2H, H−5, H−8′), 1.67 (m, 1H, H−8), 1.62 (m, 1H, H−4a), 1.50 (m, 2H, H−9, H−9a), 1.33 (m, 2H, H−4, H−4b′), 1.09 (m, 2H, H−10b, H−9b), 0.89 (t, J = 7.5 Hz, 3H, H−11′), 0.87 (t, J = 7.5 Hz, 3H, H−11). 13C NMR (125 MHz, CDCl3, assignments by DEPT, HSQC, HMBC) δ: 167.61 (C−1), 165.17 (C−1′), 144.78 (C−7′), 141.58 (C−7), 135.51 (C−7a′), 129.75 (C−7a), 115.01 (C−3′), 94.27 (C−3), 86.15 (C−3a), 59.262 (C−3a′), 44.88 (C−8), 37.85 (C−6′), 35.56 (C−8′), 29.24 (C−4), 28.99 (C−9), 25.15 (C−6), 24.73 (C−9′), 22.76 (C−10′), 22.60 (C−4′), 21.09 (C−10), 17.36 (C−5), 16.72 (C−5′), 14.10 (C−11), 13.82 (C−11′). EIMS m/z (rel. int.): 398 [M+] (21), 380 (9), 356 (100), 338 (65), 309 (11), 296 (13), 271 (15), 253 (10), 207 (5), 190 (38), 189 (10), 149 (7), 91 (5), 79 (5); HRMS (FAB+) m/z: Calcd. for [C24H30O5 + H]+: 399.2094; found 399.2092. X-Ray analysis data: All data were collected on Bruker Smart Apex CCD diffractometer using 1.51θ-25.53θ at 294(2) K. Compound 6 belongs to the orthorhombic system, space group P b c a with a = 12.1979(9) Å, α = 90º, b = 12.744(1) Å, β = 90º, c = 26.941(2) Å, γ = 90º. The refinement methods was full-matrix least squares on F2. Crystallographic data for the structure reported in this paper has been deposited with the Cambridge Crystallographic Data Centre CCDC 712971. deposit@ccdc.cam.ac.uk http://www.ccdc.cam.ac.uk/deposit. Telephone: (44) 01223 762910.
Preparation of cyclotokinolide B (7) from 5
To a solution of ketoacid of tokinolide B9 (5, 22 mg, 0.06 mmol) in THF (5 mL) and MeOH (1 mL) was added NaOH (33.1 mg, 0.83 mmol), after 2 h of reflux under nitrogen, the reaction mixture was cooled to room temperature and concentrated under vacuum. The reaction mixture was diluted with methylene CH2Cl2 (10 mL), washed with aq. HCl (10%, 2 × 10 mL), the aqueous layer was re-extracted with methylene CH2Cl2 (3 × 10 mL). The organic layers were combined and washed with brine (2 × 10 mL), dried over Na2SO4 and concentrated under vacuum. The residue was purified by preparative thin layer chromatography (n-hexane/EtOAc, 3:2) to obtain compound 79 (13.8 mg, 63%).
Computational Details
To compute the total energy here reported for 5, 6 and 7, it was necessary to proceed first to a geometry optimization procedure for each molecule. Our geometry optimization paradigm was: a) model and geometry optimize the largest rigid molecular fragment of the compound in consideration, b) to the fragment from the previous step add a new molecular fragment and perform a geometry optimization, c) carry out a relaxed potential energy scan (RPES) over the dihedral angle between the two recently added molecular fragments in step b). This RPES consists in the geometry optimization of all degrees of freedom of the molecule, with the only exception, in this case, of the assigned value to the dihedral angle. In our calculations this angle was varied from zero to 360 degrees, with increments of 45 degrees. d) The molecular conformation with the minimum energy in step c) was selected for a global geometry optimization. The steps b) to d) were repeated until the compound in consideration was completed. The identified molecular fragments with mobility were the n-propyl chains for 5, 6 and 7, in addition, for 5 and 7 the fragment O(1’)−C(1’)−O(2’)−H(2’) was considered, and for 6, the lactone ring fused with the cyclohexene was considered as another fragment. GaussView was used in all the modeling steps11. All molecular calculations were calculated at B3LYP/6−311G level of theory and were carried out with the suite of programs in Gaussian 03.12
Cytotoxic assay
Colon (HCT-15), leukaemia (K-562) and lung (SKLU-1) human tumor cell lines were supplied by National Cancer Institute (NCI), USA. The cytotoxicity of the tumors cells with the test compounds was determined using the protein-binding dye sulforhodamine B (SRB) in microculture assay to measure cell growth [23]. The cell lines were cultured in RPMI-1640 (Sigma Chemical Co., Ltd., St. Louis, MO, USA), supplemented with 10% fetal bovine serum, 2 μM L-glutamine, 100 IU/mL penicillin G, 100 μg/mL streptomycin sulfate, and 0.25 μg/mL amphotericin B (Gibco). They were maintained at 37 ºC in a 5% CO2 atmosphere with 95% humidity. For the assay, 5 × 104 cell/mL (K562), 10 × 104 cell/well (HCT-15) and 7500 cell/mL (SKLU-1), and 100 µL/well of these cells suspension were seeded in 96-well microtiter plates and incubated to allow the cell attachment. After 24 h, 100 µL of each test compounds and positive substances were added to each well. Later 48 h, adherent cell cultures were fixed in situ by adding 50 µL of cold 50% (wt/vol) trichloroacetic acid (TCA) and incubated for 60 min at 4 ºC. The supernatant was discarded and the plates were washed three times with water and air-dried. Cultured fixed with TCA were stained for 30 min with 100 µL of 0.4% SRB solution. Protein-bound dye was extracted with 10 µM unbuffered tris base and the optical densities were read on an Ultra Microplate Reader (Elx 808, BIO-TEK Instruments, Inc.), with a test wavelength of 515 nm. Results were expressed as inhibitory concentration 50 (IC50) values, they were calculated according to the protocol of Monks14 where a dose-response curve was plotted for each compound, and the concentration giving 50% inhibition (IC50) was estimated from linear regression equations. The IC50 values (mean ± standard error) are shown in Table 1.
ACKNOWLEDGEMENTS
A. L. acknowledges the financial supports of CONACyT (scholarship 181999) and Dirección General de Estudios de Posgrado, UNAM. J. A. C. is grateful to Dirección General de Servicios de Cómputo Académico, UNAM for the allocation of CPU time. The authors thank María Teresa Ramírez-Apan, María Isabel Chávez, Eréndira García, Beatriz Quiroz-García, Luis Velasco, Javier Pérez Flores, and David Vázquez for technical assistance.
References
1. J. J. Beck and Sh.-C. Chou, J. Nat. Prod., 2007, 70, 891; CrossRef M. J. M.Gijbels, J. J. C. Scheffer, and A. B. Svendsen, Riv. Ital. EPPOS, 1979, 61, 335.
2. E. Linares and R. A. Bye, J. Ethnopharmacol., 1987, 19, 153. CrossRef
3. G. Delgado, R. G. Reza-Garduño, R. A. Toscano, R. A. Bye, and E. Linares, Heterocycles, 1988, 27, 1305. CrossRef
4. M. Y. Rios and G. Delgado, Rev. Soc. Quím. Méx., 1999, 43, 127.
5. B. Quiroz-García, S. Hernández-Ortega, O. Sterner, G. Delgado, Tetrahedron, 2004, 60, 3681; CrossRef M. Y. Rios, G. Delgado, and R. A. Toscano, Tetrahedron, 1998, 54, 3355; CrossRef Y. Ogawa, Y. Mori, M. Maruno, and T. Wakamatsu, Heterocycles, 1997, 45, 1869; CrossRef B. Quiroz-García, R. Figueroa, J. A. Cogordán, and G. Delgado, Tetrahedron Lett., 2005, 46, 3003. CrossRef
6. S. Deng, S-N. Chen, P. Yao, D. Nikolic, R. B. Van Breemen, J. L. Bolton, H. H. S. Fong, N. R. Farnsworth, and G. F. Pauli, J. Nat. Prod., 2006, 69, 536. CrossRef
7. L. S. Lim, P. Shen, Y. H. Gong, and E. L. Yong, Phytochemistry, 2006, 67, 728. CrossRef
8. S. Deng, S-N. Chen, J. Lu, Z. J. Wang, D. Nikolic, R. B. van Breemen, B. D. Santarsiero, A. Mesecar, H. H. S. Fong, N. R. Farnsworth, and G. F. Pauli, Phytochem. Anal., 2006, 17, 398. CrossRef
9. B. Quiroz-García, L. Hernández, R. A. Toscano, O. Sterner, and Delgado, G. Tetrahedron Lett., 2003, 44, 2509. CrossRef
10. J. E. Baldwin and M. J. Lusch, Tetrahedron, 1982, 38, 2939. CrossRef
11. R. Dennington II, T. Keith, and J. Millam, GaussView, Version 4.1, Semichem, Inc.; Shawnee Mission, KS. 2007.
12. Gaussian 03, Revision C 02, Gaussian, Inc.; Pittsburgh, P. A. 2004.
13. J. Tirado-Rives and W. L. Jorgensen, J. Chem. Theory Comput., 2008, 4 (2), 297. CrossRef
14. A. Monks, D. Scudiero, P. Skehan, R. Shoemaker, K. Paull, D. Vistica, C. Hose, J. Langley, P. Cronise, A. Vaigro-Wolff, M. Gray-Goodrich, H. Campbell, J. Mayo, and M. Boyd, J. Natl. Cancer Inst., 1991, 83, 757. CrossRef