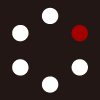
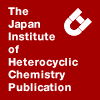
HETEROCYCLES
An International Journal for Reviews and Communications in Heterocyclic ChemistryWeb Edition ISSN: 1881-0942
Published online by The Japan Institute of Heterocyclic Chemistry
e-Journal
Full Text HTML
Received, 30th June, 2010, Accepted, 20th August, 2010, Published online, 24th August, 2010.
DOI: 10.3987/REV-10-SR(E)4
■ Properties and Bioactivities of Peptoids Tagged with Heterocycles
Irene Izzo, Chiara De Cola, and Francesco De Riccardis*
Department of Chemistry, University of Salerno, Via Ponte don Melillo, I-84084 Fisciano (SA), Italy
Abstract
N-substituted oligoglycines, also called “peptoids”, form a major class of pseudopeptides encompassing a wide range of structures and properties. Their inherent features make them useful in areas as diverse as medicinal chemistry, catalysis, and molecular recognition. In this review, the authors, with selected examples, show the wide range of properties of oligomeric peptoids tagged with heterocycles. It is also revealed how the imaginative structures created by synthetic chemists are exciting and varied, and, in which terms, their potential has just started to be addressed.CONTENTS
1 Introduction
2 Peptoids: a new class of peptidomimetics
3 Properties and bioactivities of peptoids
3.1 Metal complexing peptoids
3.2 Bioactive peptoids
3.3 Catalytic peptoids
3.4 Peptoids tagged with nucleobases
3.5 Peptoids tagged with sugars
3.5.1 O-Linked glycopeptoids
3.5.2 N-Linked glycopeptoids
3.5.3 C-Linked glycopeptoids
3.5.4 S-Linked glycopeptoids
4. Conclusions
5. Acknowledgements
6. References
1 INTRODUCTION
Through the eyes of a chemist the irreducible complexity of the natural world appears as an immensely sophisticated molecular edifice, embodying myriad of forms and architectures. Seen from a nanoscale perspective, the copious diversity of the living systems can be reduced to a simple basic principle: chemical recognition. The mutual specific interactions of the living matter multiple constituents represent the driving force for the structural accretion of the informative and catalytic life subunits. Chemical recognition not only deviates the evolution course of the material systems towards complexity increase: it configures, in the chemists imagination, as a pervading source of inspiration.
If the responsiveness to the molecular shape, through reciprocal atomic interactions, constitute the phenomenological essence of chemical recognition,1 the development of tailored molecular objects, represents its scope.2
In the continue confrontation of the chemists with the living systems macromolecules, peptides, more than other classes of natural polymers, for their conspicuous versatility and functional diversity, constitute a preferred object of investigation. Regulation of the protein-protein interactions plays a decisive role in unicellular and multicellular organisms, including man, and represents the quintessence of molecular recognition. Most of the biological processes are based on these specific interactions and their regulation or disruption can evoke striking effects on living matter.
Modern medicine tends to interpret pathologies at a molecular level and uses drugs as nanoscopic tools to restore the physiological conditions. Peptidomimetics, much stable and better performing than peptides in vivo, have surpassed the natural counterparts in the drug discovery quest.3 The exploration of the chemical space associated to abiotic scaffolds has produced an innumerable amount of analogues. Fortunately, in a “cladistic” perspective, all of them can be easily secluded in a few classes of congeners.3 In this review the attention will be focused on an emerging group of peptidomimetics: the peptoids4 and, in particular, the authors will report on peptoids tagged with heterocyclic rings. The analysis will be restricted to those examples in which the side chains’ heterocycles elicit biological activities or special properties. The overall discussion will emphasize on function, rather than synthesis, being, this last, quite easy to perform. The selected examples, not extended to cyclic pepotoids,5 will allow the readers to get a glimpse of the potentials of this class of pseudopeptides and gain inspiration for interdisciplinary research based on those biomimetic oligomers.
2 PEPTOIDS: A PROMISING CLASS OF PEPTIDOMIMETICS
Peptides are extensively used by nature for their remarkable versatility. Metabolism regulation, cell signalling, structural role, gene expression, are some of the their inescapable functions. Successful synthetic methods (such as the solid-phase peptide synthesis developed by B. Merrifiled)6 and the widespread combinatorial approach (for the elaboration and screening of peptide libraries)7 accelerated the use of peptides as lead for the drug discovery. However, their low stability against proteolysis (with the consequent short duration of the in vivo activity) offered the opportunity to explore new molecular species with the aim to “convert the information contained in peptides into small nonpeptide structures”.8 The evolution of the field, now identified with the term “peptidomimetics”,9 has substantially diversified in the last two decades producing a huge number of peptide models with higher proteolytic stability, better bioavailability and, often, showing impressing potencies and selectivities.3,9
The optimal design of peptidomimetics is based on the accurate knowledge of the three dimensional key residues arrangement in the parent peptides’ bioactive form. It is well known that most of the short peptides exist in aqueous solution in a high number of interconverting conformations10 (defined by the Ramachandran plots)11 thus, non-peptide compounds should be designed to mimic the active pharmacophore/agonist topology of the peptides from which they are derived.
In a seminal paper appeared in 1992,4a a promising approach in the search for active peptide mimics, was suggested. In that article, the side chains of the peptide α-carbon were shifted by one position on the peptide backbone producing the disappearance of all the intra-chain stereogenic centres and the formation of a sequence of variously substituted N-alkylglycines (Figure 1).
Differently from the classic solid-phase peptide synthesis (which contemplates the monomeric growing of the peptide through condensation of a N-protected aminoacid), the most blazoned approach for the construction of linear peptoids consists in the submonomer synthesis method developed by Zuckermann and co-workers (Scheme 1).12 The peptoid oligomers are built through amination of an α-bromomethylacyl residue linked to the resin. The resulting N-alkylglycine is then amidated in the presence of α-bromoacetic acid and the α-bromomethylacyl residue is again substituted with a chosen alkylamine. The process is iterated to obtain the desired oligomer, which is then cleaved from the resin and purified.
Recent contributions, report yields optimisation and acceleration of the amination/amidation reaction through micro-wave irradiation.13
The peptoid nomenclature uses the aminoacid abbreviation, preceded by “N”, when the residue on the nitrogen is identical to that on the stereogenic α-carbon (Phe becomes NPhe, Lys becomes NLys), alternatively, the three letters code identifies a chiral substituent: Nrpe for the (R)-N-(1-phenylethyl)glycine (note that the first letter reports the configuration). A two letter code, instead, is used for achiral substituents: Nbe for the N-(2-benzyloxyethyl)glycine.
Interestingly, peptoid oligomers resist to protease degradation14 and can be easily sequenced by Edman degradation or by mass spectrometry.15
3 PROPERTIES AND BIOACTIVITIES OF PEPTOIDS
Because of their ease of synthesis and structural similarity to peptides, peptoids are prime candidates as models for medicinally attractive lead compounds and scaffolds for the search of new properties. While early peptoids research focused on the generation of large combinatorial libraries, today, also thanks to the advances in their modular synthesis, more complex entities are reported, showing unexpected capabilities of the oligomeric assemblies.
In this section we will describe some interesting contribution in the peptoid field, focusing our attention on two major areas: molecular recognition and α-peptide mimics. In all the analyzed peptoid oligomers the presence of the heterocyclic component is the primary reason for their inherent properties and/or bioactivities.
3.1 METAL COMPLEXING PEPTOIDS
A desirable attribute for biomimetic peptoids is the ability to show binding towards receptor sites. This property can be evoked by proper backbone folding due to: 1) local side-chain stereoelectronic influences, 2) coordination with metallic species, and 3) presence of hydrogen-bond donor/acceptor patterns. Those three factors can strongly influence the peptoids’ secondary structure, which is difficult to observe due to the lack of the intrachain C=O···H–N bonds, present in the parent peptides.
The induction of a helical arrangement by incorporation of bulky, chiral side chains, will not be described here in that it does not necessarily involve heterocyclic substitution.16
Coordination of heterocyclic nitrogens with metallic species and branching of the N-alkyl glycine backbone with nucleobases (or other hydrogen bond donor/acceptor heterocycles) represent efficient ways to fold oligomeric peptoid sequences. However, the incorporation of the nucleophilic heterocyclic side chains into the growing peptoid backbone can represent an insurmountable obstacle for their synthesis, due to the iterative condensation of the electrophilic bromoacetate (see Scheme 1).
To solve this problem, an efficient chemical strategy, relying on the use of the less reactive chloroacetic acid,17 has emerged for the assembly of heterocyclic-tagged peptoid oligomers. This improved method (tested on a peptoid pentamer 1, Figure 2) allowed the efficient incorporation of side chains including unprotected imidazoles, pyridines, pyrazines, indoles, and quinolines.
Optimised reaction conditions were also used to build a 15-mer sequence containing six different side chains with unprotected heterocyclic nitrogens (Figure 3).
A final direct comparison between the products of a chloroacetic acid versus a bromoacetic acid submonomeric synthesis was made: the 15-mer prepared with chloroacetic acid was 82% pure and exhibited the correct molecular weight by mass spectrometry. By contrast, the expected 15-mer produced with bromoacetic acid was almost undetectable.17
The sub-monomer approach, using chloroacetic acid, was utilized by Zuckermann and co-workers for the synthesis of biomimetic peptoids with zinc-binding sites.18 Classic zinc-binding motifs, present in proteins and including thiol and imidazole moieties, were aligned in two helical peptoid sequences, in a way that they could form a binding site. Fluorescence resonance energy transfer (FRET) reporter groups were located at the edge of this biomimetic nanostructure in order to measure the distance between the two helical segments and probe, at the same time, the zinc binding propensity (Figure 4).
Folding of the two helix bundles was allowed by a Gly-Gly-Pro-Gly middle region. The study demonstrated that certain peptoids were selective zinc binders at nanomolar concentration.
Metal binding can be also used to force small peptoids into proper helical arrangements. To demonstrate this capability, Kirshenbaum and co-workers19 reported a preliminary study on the synthesis of peptoid oligomers bearing multidentate ligands (4 and 5, Figure 5). In a subsequent contribution, Ward and Kirshenbaum,20 showed that Cu(II) and Co(II) could easily bind to quinoline ligands and reinforce the inherently chiral helical secondary structures of linear peptoids (due to the presence, in the sequence of the chiral (S)-1-phenylethyl groups (Nspe), Figure 5).
The influence of the multidentate metal-binding groups on the oligomers’ helicity, has been clearly demonstrated on the basis of circular dicroism (CD). Solutions of the metal complexes, in fact, exhibited increase in the magnitude of the CD signals near 200 nm and 220 nm, relative to the metal-free peptoids.
3.2 BIOACTIVE PEPTOIDS
Peptoids’ libraries have also been shown to be an excellent source of protein-binding compounds with affinities similar to those exhibited by the peptide ligands. In a recent paper, Kodadek and co-workers,21 employed a two-color cell-based screen for the identification of five presumed vascular endothelial grown factor receptor 2 (VEGFR2)-binding peptoids from a library of over 250 000 compounds. Two of those peptoids (8 and 9) are reported in Figure 6. In particular, a dimeric derivative of 8 (not reported), demonstrated to be even more active both in vitro on angiogenesis and tumor growth in vivo (mouse models).
In a subsequent paper,21b Kodadek demonstrated that the peptoid antagonists do not compete with vascular endothelial grown factor (VEGF) for binding to VEGFR2. In this way he identified a new possible “hotspot” distinct from the hormone-binding site.
Again, combinatorial chemistry was crucial for the discovery of bioactive peptoids. With the use of proper heterocycles, Zuckermann22 discovered high affinity ligands for the µ-specific opiate receptors from a combinatorial peptoid library of 5000 synthetic dimer and trimer N-(substituted)glycines. Compound 10 (Figure 7), with a Ki of 6 nM, showed to be the most promising of the series.
A combinatorial approach was also used to identify nonpeptidic channel blockers of vanilloid receptor subunit 1 (VR1),23 which is recognized to play a critical role in the transduction of noxious chemical and thermal stimuli by sensory nerve endings in peripheral tissues. The discovered trialkylglycine with a N-methyl pyrrolidine terminal unit (11, Figure 7), showed an IC50 = 500 nM.
A tripeptoid tagged with an indol, was discovered to be a micromolar inhibitor of the bombesin receptor (12, Figure 7) among more than three hundred thousand different derivatives.24
From a combinatorial library of almost 100 000 hexameric peptoids, Kodadek and co-workers25 isolated a cell-permeable peptoid in a screen against the KIX domain of the coactivator CBP (CREB-binding protein), acting as a potent activation domain (AD) equivalent in living mammalian cells. Three peptoids were identified that bound the protein much better than the other compounds in the library. Two of these were successfully sequenced and are shown in Figure 8 (13 and 14). In particular, peptoid 13 was capable of serving as an AD, with a half maximal effective concentration (EC50) = 8 µM.
In 2007, Kodadek and co-workers26 identified the first chemical modulator of the proteasome 19S regulatory particle (which is part of the 26S proteasome, an approximately 2.5 MDa multi-catalytic protease complex responsible for most non-lysosomal protein degradation in eukaryotic cells). A “one bead one compound” peptoid library was constructed by split and pool synthesis. Each peptoid molecule was capped with a purine analogue in hope of biasing the library toward targeting one of the ATPases, which are part of the 19S regulatory particle. Approximately 100 000 beads were used in the screen and a purine-capped peptoid heptamer (15, Figure 9) was identified as the first chemical modulator of the 19S regulatory particle. In an effort to evidence the pharmacophore of the peptoid heptamer 1527 (by performing a “glycine scan”, similar to the “alanine scan” in peptides) it was shown that just the core tetrapeptoid was necessary for the activity. Interestingly, the synthesis of the shorter peptoid 16 gave, in the experiments made on cells, a 3- to 5-fold increase in activity relative to 15. The higher activity in the cell-based essay was likely due to increased cell uptake, as 16 do not contains charged residues.
As reported in Section 2 of this review, one strategy to design successful inhibitors of protein-protein interactions is to recreate the three-dimensional arrangement of side chains that are involved in the binding of one protein to another, using a nonnatural scaffolds as the attachment point for the side chains. In a beautiful study by Appella and co-workers,28 oligomeric peptoids were used as scaffold for inhibition of HDM2-p53 interaction. The disruption of the HDM2-p53 interactions can have important effects on cell proliferation, in fact, the free p53 acts as a transcription factor able to promote production of other proteins that result in cell cycle arrest or apoptosis. In the Appella contribution, it was found that the achiral peptoid 17 (Figure 10) acted as effective inhibitor of HDM2-p53 binding.
3.3 CATALYTIC PEPTOIDS
An interesting example of the imaginative use of reactive heterocycles in the peptoid field, can be found in the “foldamers” mimics. “Foldamers” mimics are synthetic oligomers displaying conformational ordering. They have never been explored as platform for asymmetric catalysis. In an elegant paper by Ward and Kirshenbaum,29 it was reported the synthesis of a library of helical “peptoid” oligomers enabling the oxidative kinetic resolution (OKR) of 1-phenylethanol induced by the catalyst TEMPO (2,2,6,6-tetramethylpiperidine-1-oxyl) (Figure 11).
The TEMPO residue was covalently integrated in properly designed chiral peptoid backbones which were used as asymmetric components in the oxidative resolution.
The study demonstrated that the enantioselectivity of the catalytic peptoids (built using the chiral (S)- and (R)-phenylethyl amines) depended on three factors: 1) the handedness of the asymmetric environment derived from the helical scaffold, 2) the position of the catalytic centre along the peptoid backbone, and 3) the degree of conformational ordering of the peptoid scaffold. The highest activity in the OKR (e.e. > 99%) was observed for the catalytic peptoids with the TEMPO group linked at the N-terminus, as evidenced in the peptoid backbones 21 and 22 (reported in Figure 12). These results revealed that the selectivity of the OKR was governed by the global structure of the catalyst and not solely from the local chirality at sites neighbouring the catalytic centre.
3.4 PEPTOIDS TAGGED WITH NUCLEOBASES
Self-recognition by nucleic acids is a fundamental process of life. Although, in nature, proteins are no carriers of genetic information, pseudopeptides bearing nucleobases, denominate “peptide nucleic acids” (PNA, 23, Figure 13),3a can mimic the biological functions of DNA and RNA (24 and 25, Figure 13).
The remarkable hybridization and biostability properties make PNA of considerable interest in several areas of chemistry, biology and medicine.30 However, despite the excellent attributes, PNA has two serious limitations: low water solubility31 and poor cellular uptake.32 A significant number of PNA derivatives have been designed and synthesised to circumvent these drawbacks, leading to a variety of promising analogs,33 but the quest for the optimal PNA derivative is still open.
The first example of “peptoid nucleic acid” was reported by Almarsson and Zuckermann.34 The shift of the amide carbonyl groups away from the nucleobase (towards the backbone) and their replacement with methylenes, resulted in a nucleosidated peptoid skeleton (26, Figure 13). Theoretical calculations showed that the modification of the backbone had the effect of abolishing the “strong hydrogen bond between the side chain carbonyl oxygen (α to the methylene carrying the base) and the backbone amide of the next residue”, which was supposed to be present on the PNA (27, Figure 14) and considered essential for the DNA hybridization.
The thyminylated peptoid nonamer synthesised (28, Figure 15),34 in fact, was not able to hybridize the complementary DNA single strand.
In subsequent contributions, it was shown that no spectral35 or crystallographic evidence36 ever supported the fact that in the PNA is present such intramolecular hydrogen bond.
The experimental observation that interchanging the position of –CH2– and side chain –CO– groups in PNA abolishes the interaction with DNA is still debated. It is interesting to note that a good hybridization with DNA is restored when methyl groups substitute the amide hydrogens, giving a full peptoid backbone (29, Figure 16).37 A lysinated decamer was synthesised on a benzhydrilamine resin using the dipeptoid building-block 30.
The hybridization experiment was conducted with d(A)10 and the observed Tm was 42 °C. This result demonstrated that the relative stability of a duplex consisting of the modified peptoid nucleic acid and the DNA was higher than that of the DNA/DNA double strand with equal nucleobases (Tm = 22 °C). Unfortunately, this finding (published by J. C. Xu, in the form of a brief communication on the Chinese Chemical Letters) was not followed by any full account.
Another interesting report, demonstrating that the peptoid backbone is compatible with hybridization, came from the Eschenmoser laboratory in 2007.38 This finding was part of an exploratory work on the pairing properties of triazine heterocycles (as recognition elements) linked to peptide and peptoid oligomeric systems. In particular, when the backbone of the oligomers was constituted by condensation of iminodiacetic acid (31 and 32, Figure 17), the hybridization experiments, conducted with oligomer 32 and d(T)12, showed a Tm = 22.7 °C.
This interesting result, apart from the implications in the field of prebiotic chemistry, suggested the preparation of a similar peptoid oligomers (made by iminodiacetic acid) incorporating the classic nucleobase thymine (33 and 34, Figure 18).39
The peptoid oligomers 33 and 34 showed thymine residues separated by the backbone by the same number of bonds found in nucleic acids (Figure 19, bolded black bonds). Also the spacing between the recognition units on the peptoid framework was similar to that present in the DNA (Figure 19, bolded grey bonds).
However, annealing experiments demonstrated that peptoid oligomers 33 and 34 do not hybridize complementary strands of d(A)16 or poly-r(A). It was claimed that possible explanations for those results resided in the conformational restrictions imposed by the charged oligoglycine backbone and in the high conformational freedom of the nucleobases (separated by two methylenes from the backbone).
Small backbone variations may also have large and unpredictable effects on the nucleosidated peptoid conformation and on the binding to nucleic acids as recently evidenced by Liu and co-workers,40 with their synthesis and incorporation (in a PNA backbone) of Nγ-ε-aminoalkyl residues (38, Figure 20).
In the Liu systematic study, it was found that short polar side chains (protruding from the γ-nitrogen of peptoid-based PNAs) negatively influence the hybridization properties of modified PNAs, while longer polar side chains positively modulate the nucleic acids binding. The reported data did not clarify the reason of this effect, but it was speculated that factors different from electrostatic interaction are at play in the hybridization.
3.5 PEPTOIDS TAGGED WITH SUGARS
The chemical synthesis of complex glycopeptides still remains an arduous task. The elaboration of glycoproteins, in fact, embodies the difficulties of oligosaccharide chemistry and those of the peptide synthesis. Demanding glycosyl coupling reactions, extensive protective group manipulation, challenging ligations, make glycopeptides formidable synthetic targets.
For all those reasons, while chemists experience new creative approach for the generation of artificial glycoproteins, efforts are made toward the design of new and effective glycopeptide mimetics.41 Priorities, for this scope, are a lower structural/synthetic complexity and a higher stability to endogenous enzymatic degradation.
Several studies on enzyme-catalyzed hydrolysis demonstrated that substitution of the glycosidic C–O or C–N bonds (present in O- and N-linked glycoproteins) with a C–S or C–C bond confers resistance to enzymatic hydrolysis.42 In this complex scenario, peptoids are playing their role and today the “glycopeptoids” represent an important class of promising mimetics.
In this section we will report the most notable examples of peptoids tagged with sugars. To date, interesting synthetic approaches to O-, N-, C- and S-linked glycopeptoids have appeared in the literature. We will analyze them in the subsequent sections, focusing our attention on their properties.
3.5.1 O-LINKED GLYCOPEPTOIDS
The first oligomeric glycopeptidomimetics were synthesized by R. Roy and co-workers.43 In particular, α-O-linked N-acetylgalactosamine clusters with 2, 3, 4, 6, and 8 repeating units were synthesized using an iterative blockwise approach. The glycomimetics 39-43 (Figure 21) were synthesized to evaluate the role of multivalency in the TN-antigen presentation in metabolically stable glycopeptide analogs.
In a parallel effort,44 a glycopeptoid mimicking the β-D-Xyl-(1→3)-O-L-Ser linkage region of proteoglycans was synthesised using the classic submonomer approach. The 2-aminoethyl β-D-xylopyranoside present in the target 44 (Figure 22) constituted the key glycan L-Ser mimic.
Another interesting contribution, in the field of O-linked glycomimetics came from Hashimoto and co-workers.45 In particular, it was found that the dansylated pentameric oligomannosyl peptoid (45, Figure 23) was able to bind the lectin protein concanavalin A.
3.5.2 N-LINKED GLYCOPEPTOIDS
The first N-linked glycopeptoid (46, Figure 24) was synthesised by Roy and Saha.46 It contained an N-acetylglucosamine and was constructed using a mixed monomer/submonomer approach.
The N-acetylglucosaminated peptoid 47 (Figure 25), as prototypical HIV-1 protease inhibitor, was built, using a blockwise approach, by the same authors.47 The analog showed a low inhibitory activity in standard HIV-1 protease assays.
Recent research focuses on the N-linked glycopeptoid synthesis proposing microwave assisted glycosidation,48 using hexafluoroacetone as protecting and activating agent,49 and on-resin click-glycoconjugation. 50
3.5.3 C-LINKED GLYCOPEPTOIDS
A method for the stereoselective synthesis of the C-linked glycopeptoid building block 48 (Figure 26) has been reported by Kessler and co-workers.51 Once incorporated in the glycopeptoid, the C-glycoside should provide additional stability towards chemical and enzymatic hydrolisis.
3.5.4 S-LINKED GLYCOPEPTOIDS
In 2009 a straightforward strategy has been developed for the preparation of S-glycosidated peptoids.52 It relies on the combination of a rapid synthesis of glycosidated building block from inexpensive precursors and their direct incorporation into a time-effective and high yielding submonomer solid-phase approach. The versatility of the method has been demonstrated by the preparation of the three glycopeptoids 49-51, reported in Figure 27.
4 CONCLUSIONS
Molecular recognition of a target and conformational control of a three-dimensional structure is not only the basis of the biological world, but also the foundation for rational drug design. Since the oligomers of N-substituted glycines were discovered, in the early 1990’s, they immediately became an attractive field of investigation for their chemical, biological and structural potential. Peptoids opened a new dimension in peptide chemistry and remain a preferred scaffold for biological application for their straightforward modular synthesis that allows the incorporation of a wide variety of side chains and functional groups.
The ultimate goal of this perspective was to evidence, with several examples, how a particular group of these compounds impacted on medicinal chemistry, catalysis, molecular recognition, biology and pharmacology.
In summary, the application of peptoids are exiting and varied and we feel that their potential has only just started to be addressed.
5 AKNOWLEDGEMENTS
Financial support from the University of Salerno.
References
1. S. H. Gellman, Chem. Rev., 1997, 97, 1231. CrossRef
2. J.-M. Lehn, 'Supramolecular Chemistry: Concepts and Perspectives, ' VCH, Weinheim, 1995.
3. (a) 'Pseudo-peptides in Drug Development,' ed. by P. E. Nielsen, Wiley-VCH, Weinheim, 2004; For β-peptides, see: (b) D. H. Appella, L. A. Christianson, D. A. Klein, D. R. Powell, X. Huang, J. J. Barchi, Jr., and S. H. Gellman, Nature, 1997, 387, 381; CrossRef (c) D. Seebach, M. Overhand, F. N. M. Kühnle, B. Martinoni, L. Oberer, U. Hommel, and H. Widmer, Helv. Chim. Acta, 1996, 79, 913; CrossRef (d) B. L. Iverson, Nature, 1997, 385, 113; CrossRef (e) D. Seebach, J. L. Matthews, Chem. Commun., 1997, 21, 2015; CrossRef (f) S. H. Gellman, Acc. Chem. Res., 1998, 31, 173; CrossRef (g) E. A. Porter, X. Wang, H. S. Lee, B. Weiblum, and S. H. Gellman, Nature, 2000, 405, 298; CrossRef (h) D. Liu and W. F. DeGrado, J. Am. Chem. Soc., 2001, 123, 7553; CrossRef (i) N. Umezawa, M. A. Gelman, M. C. Haigis, R. T. Raines, and S. H. Gellman, J. Am. Chem. Soc., 2002, 124, 368; CrossRef (j) T. L. Raguse, J. R. Lai, P. R. LePlae, and S. H. Gellman, Org. Lett., 2001, 3, 3963; CrossRef (k) R. P. Cheng and W. F. DeGrado, J. Am. Chem. Soc., 2002, 124, 11564; CrossRef (l) D. S. Daniels, J. Petersson, J. X. Qui, and A. Schepartz, J. Am. Chem. Soc., 2007, 129, 1532; CrossRef (m) D. Seebach and J. Cardiner, Acc. Chem. Res., 2008, 41, 1366; CrossRef for γ-peptides, see: (n) T. Hintermann, K. Gademann, B. Jaun, and D. Seebach, Helv. Chim. Acta, 1998, 81, 983; CrossRef (o) S. Hanessian, X. Luo, R. Schuam, and S. Michnick, J. Am. Chem. Soc., 1998, 120, 8569; CrossRef (p) D. Seebach, L. Schaeffer, M. Brenner, and D. Hoyer, Angew. Chem. Int. Ed., 2003, 42, 776; CrossRef for azatides, see: (q) J. Han and K. D. Janda, J. Am. Chem. Soc., 1996, 118, 2539; CrossRef for oligopyrrolinones, see. (r) A. B. Smith III, M. C. Guzman, P. A. Sprengeler, T. P. Keenan, R. C. Holcomb, J. L. Wood, and R. Hirschmann, J. Am. Chem. Soc., 1994, 116, 9947; CrossRef for oligoureas, see: (s) K. Burgress, D. S. Linthicum, and H. Shin, Angew. Chem., Int. Ed. Engl., 1995, 34, 907; CrossRef (t) J.-M. Kim, T. E. Wilson, T. C. Norman, and P. G. Schultz, Tetrahedron Lett., 1996, 37, 5309; CrossRef (u) C. Hemmerlin, M. Marraud, D. Rognan, R. Graff, V. Semetey, J. P. Briand, and G. Guichard, Helv. Chim. Acta, 2002, 85, 3692; CrossRef for oligocarbamates, see: (v) C. Y. Cho, E. J. Moran, S. R. Cherry, J. C. Stephans, S. P. A. Fodor, C. L. Adams, A. Sundaram, J. W. Jacobs, and P. G. Schultz, Science, 1993, 261, 1303; CrossRef (x) E. J. Moran, T. E. Wilson, C. Y. Cho, S. R. Cherry, and P. G. Schultz, Biopolymers, 1995, 37, 213; CrossRef for oligoanthanilamides, see: (y) Y. Hamuro, S. J. Geib, A. D. Hamilton, J. Am. Chem. Soc., 1996, 118, 7529; CrossRef (w) J. T. Ernst, J. Becerril, H. S. Park, H. Yin, and A. D. Hamilton, Angew. Chem. Int. Ed., 2003, 42, 535; CrossRef for oligosulfones, see: (z) C. Gennari, B. Salom, D. Potenza, and A. Williams, Angew. Chem., Int. Ed. Engl., 1994, 33, 2067; CrossRef for @-tides, see: (z’) S. T. Philips, M. Rezac, U. Abel, M. Kossenjans, and P. A. Bartlett, J. Am. Chem. Soc., 2002, 124, 58. CrossRef
4. (a) R. J. Simon, R. S. Kania, R. N. Zuckermann, V. D. Huebner, D. A. Jewell, S. Banville, S. Ng, L. Wang, S. Rosenberg, C. K. Marlowe, D. C. Spellmeyer, R. Tan, A. D. Frankel, D. V. Santi, and F. E. Cohen, and P. A. Bartlett, Proc. Natl. Acad. Sci. U. S. A., 1992, 89, 9367; CrossRef (b) S. A. Fowler and H. Blackwell, Org. Biomol. Chem., 2009, 7, 1508; CrossRef (c) B. Yoo and K. Kirshenbaum, Curr. Opin. Chem. Biol., 2008, 12, 714; CrossRef (d) J. A. Patch, K. Kirshenbaum, S. L. Seurynck, R. N. Zuckermann, and A. E. Barron, 'Pseudo-peptides in Drug Development,' ed. By P. E. Nielsen, Wiley-VCH, Weinheim, 2004, pp. 1-31.
5. Two interesting reviews on cyclic peptoids are reported: (a) L. A. Wessjohann, C. K. Z. Andrade, O. E. Vercillo, and D. G. Rivera, 'Targets in Heterocyclic Systems,' ed. by O. A. Attanasi, D. Spinelli, Italian Society of Chemistry, 2007, vol. 10, pp. 24-53; (b) B. Yoo, S. B. Shin, M. L. Huang, and K. Kirshenbaum, Chem. Eur. J., 2010, 16, 5528. CrossRef
6. R. B. Merrifield, J. Am. Chem. Soc., 1963, 85, 2149. CrossRef
7. (a) K. S. Lam, M. Lebl, and V. Krchnak, Chem. Rev., 1997, 97, 411; CrossRef (b) P. T. Corbett, J. Leclaire, L. Vial, K. R. West, J.-L. Wietor, J. K. M. Sanders, and S. Otto, Chem. Rev., 2006, 106, 3652. CrossRef
8. R. A. Wiley and D. H. Rich, Med. Res. Rev., 1993, 33, 1699.
9. (a) A. Grauer and B. König, Eur. J. Org. Chem., 2009, 5099; CrossRef (b) Y.-D. Wu and S. Gellman, Acc. Chem. Res., 2008, 41, 1231. CrossRef
10. R. M. Freidinger, Trends. Pharmacol. Sci., 1989, 10, 270. CrossRef
11. G. N. Ramachandran and C. Ramakrishnan, Biophys. J., 1965, 5, 909. CrossRef
12. R. N. Zuckermann, J. M. Kerr, B. H. Kent, and W. H. Moos, J. Am. Chem. Soc., 1992, 114, 10646. CrossRef
13. (a) E. Nnanabu and K. Burgess, Org. Lett., 2006, 8, 1259; CrossRef (b) B. C. Gorske, S. A. Jewell, E. J. Guerard, and H. E. Blackwell, Org. Lett., 2005, 7, 1521; CrossRef (c) H. J. Olivos, P. G. Alluri, M. M. Reddy, D. Salony, and T. Kodadek, Org. Lett., 2002, 4, 4057; CrossRef (d) for N-substituted monomers, see: M. A. Fara, J. J. Díaz-Mochón, and M. Bradley, Tetrahedron Lett., 2006, 47, 1011. CrossRef
14. S. M. Miller, R. J. Simon, S. Ng, R. N. Zuckermann, M. J. Kerr, and W. H. Moos, Drug Dev. Res., 1995, 35, 20. CrossRef
15. (a) T. S. Burkoth, E. Beausoleil, S. Kaur, D. Tang, F. E. Cohen, and R. N. Zuckermann, Chem. Biol., 2002, 9, 647; CrossRef (b) W. Heerma, C. Verluis, C. G. de Koster, J. A. W. Kruijtzer, I. Zigrovic, and R. M. J. Liskamp, Rapid Commun. Mass Spectrom., 1996, 10, 459; CrossRef (c) W. Heerma, J.-P. J. L. Boon, C. Verluis, J. A. Kruijtzer, L. J. F. Hofmeyer, and R. M. J. Liskamp, J. Mass Spectrom., 1997, 32, 697; CrossRef (d) A. Boeijen and R. M. J. Liskamp, Tetrahedron Lett., 1998, 39, 3589. CrossRef
16. See, for example, ref. 3 (a) pp. 13–18 and: (a) K. Kirshenbaum, A. E. Barron, R. A. Goldsmith, P. Armand, E. K. Bradley, K. T. Truong, K. A. Dill, F. E. Cohen, and R. N. Zuckermann, Proc. Natl. Acad. Sci. U.S.A., 1998, 95, 4303; CrossRef (b) P. Armand, K. Kirshenbaum, R. A. Goldsmith, S. Farr-Jones, A. E. Barron, K. T. Truong, K. A. Dill, D. F. Mierke, F. E. Cohen, R. N. Zuckermann, and E. K. Bradley, Proc. Natl. Acad. Sci. U.S.A., 1998, 95, 4309; CrossRef (c) C. W. Wu, T. J. Sanborn, K. Huang, R. N. Zuckermann, and A. E. Barron, J. Am. Chem. Soc., 2001, 123, 2958. CrossRef
17. T. S. Burkoth, A. T. Fafarman, D. H. Charych, M. D. Connolly, and R. N. Zuckermann, J. Am. Chem. Soc., 2003, 125, 8841. CrossRef
18. B.-C. Lee, T. K. Chu, K. A. Dill, and R. N. Zuckermann, J. Am. Chem. Soc., 2008, 130, 8847. CrossRef
19. G. Maayan, B. Yoo, and K. Kirshenbaum, Tetrahedron Lett., 2008, 49, 335. CrossRef
20. G. Maayan, M. D. Ward, and K. Kirshenbaum, Chem. Commun., 2009, 56. CrossRef
21. (a) D. G. Udugamasooriya, S. P. Dineen, R. A. Brekken, and T. Kodadek, J. Am. Chem. Soc., 2008, 130, 5744; CrossRef (b) D. G. Udugamasooriya, C. Ritchie, R. A. Brekken, and T. Kodadek, Bioorg. Med. Chem., 2008, 16, 6338. CrossRef
22. R. N. Zuckermann, E. J. Martin, D. C. Spellmeyer, G. B. Stauber, K. R. Shoemaker, J. M. Kerr, G. M. Figliozzi, D. A. Goff, M. A. Siani, R. J. Simon, S. C. Banville, E. G. Brown, L. Wang, L. S. Richter, and W. H. Moos, J. Med. Chem., 1994, 37, 2678. CrossRef
23. C. Garcìa-Martıìnez, M. Humet, R. Planells-Cases, A. Gomis, M. Caprini, F. Viana, E. De la Peña, F. Sanchez-Baeza, T. Carbonell, C. De Felipe, E. Pérez-Payà, C. Belmonte, A. Messeguer, and A. Ferrer-Montiel, Proc. Natl. Acad. Sci. USA, 2002, 99, 2374. CrossRef
24. G. Hetzmann, P. Hildebrand, H. Tanner, S. Ketterer, A. Pansky, S. Froidevaux, C. Beglinger, and A. N. Eberle, J. Receptor Signal Transduct. Res., 1999, 19, 449. CrossRef
25. B. Liu, P. G. Alluri, P. Yu, and T. Kodadek, J. Am. Chem. Soc., 2005, 127, 8254. CrossRef
26. H.-S. Lim, C. T. Archer, and T. Kodadek, J. Am. Chem. Soc., 2007, 129, 7750. CrossRef
27. H.-S. Lim, C. T. Archer, Y.-C. Kim, T. Hutchens, and T. Kodadek, Chem. Commun., 2008, 1064. CrossRef
28. T. Hara, S. R. Durell, M. C. Myers, and D. H. Appella, J. Am. Chem. Soc., 2006, 128, 1995. CrossRef
29. G. Maayan, M. D. Ward, and K. Kirshenbaum, Proc. Natl. Acad. Sci. USA, 2009, 106, 13679. CrossRef
30. (a) A. Porcheddu and G. Giacomelli, Curr. Med. Chem., 2005, 12, 2561; CrossRef (b) P. E. Nielsen, Curr. Opin. Biotechnol., 2001, 12, 16; CrossRef (c) E. Uhlmann, A. Peyman,G. Breipohl, and D. W.Will, Angew. Chem. Int. Ed., 1998, 37, 2796; CrossRef (d) P. E. Nielsen and G. Haaima, Chem. Soc. Rev., 1997, 26, 73. CrossRef
31. J. P. Vernille, L. C. Kovell, and J. W. Schneider, Bioconjugate Chem., 2004, 15, 1314. CrossRef
32. (a) U. Koppelhus and P. E. Nielsen, Adv. Drug. Delivery Rev., 2003, 55, 267; CrossRef (b) P. Wittung, J. Kajanus, K. Edwards, P. E. Nielsen, B. Nordén, and B. G. Malmstrom, FEBS Lett., 1995, 365, 27. CrossRef
33. (a) E. A. Englund, D. H. Appella, Angew. Chem. Int. Ed., 2007, 46, 1414; CrossRef (b) A. Dragulescu-Andrasi, S. Rapireddy, G. He, B. Bhattacharya, J. J. Hyldig-Nielsen, G. Zon, and D. H. Ly, J. Am. Chem. Soc., 2006, 128, 16104; CrossRef (c) P. E. Nielsen, Q. Rev. Biophys., 2006, 39, 1; CrossRef (d) A. Abibi, E. Protozanova, V. V. Demidov, and M. D. Frank-Kamenetskii, Biophys. J., 2004, 86, 3070. CrossRef
34. O. Almarsson, T. C. Bruice, J. Kerr, and R. N. Zuckermann, Proc. Natl. Acad. Sci. USA, 1993, 90, 7518. CrossRef
35. (a) S. C. Brown, S. A. Thomson, J. M. Veal, and D. G. Davis, Science, 1994, 265, 777; CrossRef (b) M. Eriksson and P. E. Nielsen, Nat. Struct. Biol., 1996, 3, 410. CrossRef
36. L. Betts, J. A. Josey, J. M. Veal, and S. R. Jordan, Science, 1995, 270, 1838. CrossRef
37. (a) Y. Wu and J.-C. Xu, Chin. Chem. Lett., 2000, 11, 771; for the Boc protected thyminylated dipeptoid monomers synthesis see: (b) Y. Wu, J.-C. Xu, J. Liu, and Y.-X. Jin, Thetrahedron, 2001, 57, 3373. CrossRef
38. G. K. Mittapalli, R. R. Kondireddi, H. Xiong, O. Munoz, B. Han, F. De Riccardis, R. Krishnamurthy, and A. Eschenmoser, Angew. Chem. Int. Ed., 2007, 46, 2470. CrossRef
39. R. Zarra, D. Montesarchio, C. Coppola, G. Bifulco, S. Di Micco, I. Izzo, and F. De Riccardis, Eur. J. Org. Chem., 2009, 6113. CrossRef
40. X.-W. Lu, Y. Zeng, and C.-F. Liu, Org. Lett., 2009, 11, 2329. CrossRef
41. L. A. Marcaurelle and C. R. Bertozzi, Chem. Eur. J., 1999, 5, 1384. CrossRef
42. M. A. Sparks, K. W. Williams, and G. M. Whitesides, J. Med. Chem., 1993, 36, 78. CrossRef
43. J. M. Kim and R. Roy, Tetrahedron Lett., 1997, 38, 3487. CrossRef
44. J. M. Kim and R. Roy, Carbohydr. Lett., 1997, 298, 173. CrossRef
45. (a) H. Yuasa, Y. Kamata, S. Kurono, and H. Hashimoto, Bioorg. Med. Chem. Lett., 1998, 8, 2139; CrossRef (b) H. Yuasa, H. Honma, H. Hashimoto, M. Tsunooka, and K. Kojima-Aikawa, Bioorg. Med. Chem. Lett., 2007, 17, 5274. CrossRef
46. U. K. Saha and R. Roy, Tetrahedron Lett., 1995, 36, 3635. CrossRef
47. U. K. Saha and R. Roy, Tetrahedron Lett., 1997, 38, 7697. CrossRef
48. J. Seo, N. Michaelian, S. C. Owens, S. T. Dashner, A. J. Wong, A. E. Barron, and M. T. Carrasco, Org. Lett., 2009, 11, 5210. CrossRef
49. (a) K. Burger, C. Böttcher, G. Radics, and L. Hennings, Tetrahedron Lett., 2001, 42, 3061; CrossRef (b) K. Burger, C. Böttcher, L. Hennings, and S. A. Essawy, Monatsh. Chem., 2004, 135, 865. CrossRef
50. A. S. Norgren, C. Budke, Z. Majer, C. Heggemann, T. Koop, and N. Sewald, Synthesis, 2001, 448.
51. M. A. Dechantsreiter, F. Burkhart, and H. Kessler, Tetrahedron Lett., 1998, 39, 253. CrossRef
52. D. Comegna and F. De Riccardis, Org. Lett., 2009, 11, 3898 CrossRef