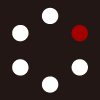
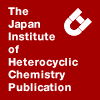
HETEROCYCLES
An International Journal for Reviews and Communications in Heterocyclic ChemistryWeb Edition ISSN: 1881-0942
Published online by The Japan Institute of Heterocyclic Chemistry
e-Journal
Full Text HTML
Received, 11th March, 2010, Accepted, 28th April, 2010, Published online, 28th April, 2010.
DOI: 10.3987/REV-10-671
■ Chemistry and Biological Activities of Vibsane-Type Diterpenoids
Yoshiyasu Fukuyama,* Miwa Kubo, Tomoyuki Esumi, Kenichi Harada, and Hideaki Hioki
Faculty of Pharmaceutical Sciences, Tokushima Bunri University, 180 Nishihamabouji, Yamashiro-machi, Tokushima, 770-8514, Japan
Abstract
This review focuses on the structural diversity, biological activities and synthesis of vibsane-type diterpenoids. Vibsane-type diterpenoids are considered to be rarely occurring natural products because they have been found exclusively in a few Viburnum species such as V. awabuki, V. odoratissimum, and V. suspensum. These diterpenoids are further classified into 11-membered ring, 7-membered ring, and rearranged (neovibsanin) types, and therefore, their chemical diversity forms a unique chemical library. We describe the absolute stereochemistry of the typical 11-membered ring vibsanins B and F, a variety of vibsane-type diterpenoids, the chemical correlations between the three subtypes, their biological activities, and the synthesis of vibsanin F and neovibsanin B.INTRODUCTION
The genus Viburnum consists of about 150 species of shrubs or small trees that were previously included in the family Caprifoliaceae. However, recent classifications based on molecular phylogeny have put them in the family Adoxaceae.1 They are distributed in the temperate Northen Hemisphere, with a few species extending into tropical regions in South America and Southeast Asia, and about 15 species are distributed in Japan.2 There is a long history of the folk medicinal use of Viburnum species. For example, the dried bark of V. opulus, which is known as "Cramp Bark," is used to alleviate painful menstrual cramps as well as a sedative.3 Native American women took black haw (V. prunifolium) to treat the menopause and menstrual cramps.4 The genus Viburnum has been documented to contain a variety of compounds such as iridoids, terpenoids, and aromatic compounds.5, 6 Among the chemical contents of Viburnum species, vibsane-type diterpenoids are considered to be characteristic of the Viburnum species because they have not been found in other higher plants. In this review, we focus on the structural diversity, biological activities and synthesis of vibsane-type diterpenoids.
1. Vibsane-type diterpenoids
In 1980, Kawazu reported the isolation of vibsane-type diterpenoids from the leaves of Viburnum awabuki, and they were shown to consist of a unique fumulane skeleton with an additional C5 unit (Figure 1).
However, there has been little interest in their chemical structure and biological activity since their discovery. Since 1996, we have continued to investigate the vibsane-type diterpenoids that are specific to Viburnum species and their biological activities, resulting in the discovery of about 60 new diterpenoids. They possess unique structures, some of which have unexpected chemical reactivity and interesting biological activity. The first vibsane-type diterpenoids that were reported by Kawazu were vibsanins A (17), B (1), and F (3), which possess an 11-membered ring, and vibsanins C (2) – E (16), which have a 7-membered ring (Figure 2).7 Their stereochemistry has remained unexplored except for that of vibsanin E (16).8 Since these diterpenoids consist of a new carbon skeleton, we have proposed the new term of “Vibsane” for diterepenoids possessing a fumulane carbon framework with an additional isoprene unit.9
2.1. The stereochemistry of vibsanins B (1) and C (2)11
It was necessary to determine the absolute stereochemistry of vibsanins B (1) and C (2) before discussing the structures of the newly isolated vibsane-type diterpenoids. The 1H NMR of 1 showed two kinds of broad signals at room temperature, but a pair of the sharp signals was observed at 0˚C. This phenomenon indicated that vibsanin B (1) is present in solution as two conformational isomers.
Two conformers, 1a and 1b, were elucidated on the basis of NOESY data and J values (Figure 3). The main conformer, 1a, demonstrated the NOE correlations shown in Figure 3 and had a high J8,9 (9.3 Hz) value. These NMR data suggested that 1a adopts a chair-like conformation for the sequential bonds from C-5 to C-10 with a dihedral angle of 180˚ between C8-H and C9-H and takes a transoid geometry for the α,β-unsaturated ketone at C4–C6, whereas 1b consists of a boat conformation and has a cisoid form according to NOE analysis and its small J8,9 (2.2 Hz). Additionally, 1a and 1b were consistent with the two most stable conformers, CT and BC, found by the MM2 calculations.31 In the course of the VT (variable temperature) experiments for 1 in DMSO-d6, we found that 1 induced an irreversible change at 110˚C. To elucidate this thermal transformation in detail, a solution of 1 in toluene was refluxed for 1 h to give rise to four products, which eventually were found to correspond to 7-membered ring vibsanin C (2) (85.9%) and its stereoisomers 2a (11.2%), 2b (1.6%), and 2c (0.2%) (Scheme 1). Vibsanin C (2) and 5-epi-vibsanin C (2b) are natural products, but 2a and 2c have been not found in nature. The formation of the four 7-membered ring vibsanins can be ascribed to the oxy-Cope rearrangement of 1. The major product, vibsanin C (2), and its 5-epimer, 2b, which contains a Δ8,9 E olefin, are considered to rearrange through the CT and BC conformers, respectively; whereas, 2a and 2c, which contain Δ8,9 Z olefin, are presumably transformed through the CC and BT conformers, which were found to be within 6 kcal/mol of the global minimum energy by MM2 calculations (Figure 4).
Thus, the absolute configuration of the 11-membered ring vibsanin B (1) was completely correlated with that of the 7-membered ring vibsanin C (2) via an oxy-Cope rearrangement. Next, the enol ester of 2 was saponified under basic conditions, which was followed by an intramolecular aldol condensation reaction to give rise to the aldehyde 2d, which was converted to the bromophenyl carbamate 2e. X-ray crystallographic analysis of 2e unambiguously established the absolute 5S, 10R, and 11S configurations of vibsanin C (2) (Scheme 2). This result means that vibsanin B (1) has chiral centers of 7R, 8R, and 11S. Thus, the absolute structures of 1 and 2, which were previously unsolved, have been established (Figure 5).
2.2. Determination of the absolute configuration of vibsanin F (3) by synthesis12
Although vibsanin F (3), which was isolated from the leaves of V. awabuki in 1980, belongs to the simplest 11-membered ring form of the vibsane-type diterpenoids, its stereochemistry has never been solved.7 We have decided to unambiguously determine the absolute stereochemistry of 3 via its asymmetric synthesis. Vibsanin F (3) has three chiral centers, among which C-7 and C-11 are anticipated to be 7S and 11S, respectively, based on those of vibsanin B (1) as shown in Figure 6. However, the chirality of C-6 may be 3a (6S) or 3b (6R).
First, we selected 3a as the first synthetic target. The synthetic procedures used are outlined in Schemes 3 and 4. Asymmetric epoxidation of the allyl alcohol 4 by the Sharpless protocol13 provided 5, which had 6S and 7S chiral centers that corresponded to those of 3a. Regioselective epoxidation of 5 with m-chloroperbenzoic acid exclusively gave the diepoxide 6 as a diastereomeric mixture. The primary hydroxyl group of 6 was converted to its triflate, with which a large excess of the dianion of methyl acetoacetate was reacted at 0˚C to give rise to 7 in high yield. The introduction of a 4-methyl-3-pentenyl unit using sodium hydride and 15-crown-5 in DMSO gave rise to the precursor 8 in good yield, which was required for the subsequent palladium-catalyzed macrocyclization. Subjecting 8 to the Tsuji-Trost reaction using 10 mol% Pd(PPh3)4 in DMSO afforded the sole product 9 in a moderate yield.14 The stereoselective formation of 9 can be explained by assuming that transition states A and B, as shown in Figure 7, are involved in this cyclization. If the nucleophilic displacement of the π-allylpalladium intermediate with the anion of the β-ketoester moiety proceeds through a product-like transition state, transition state A, which should lead to the more stable product 9 with a pseudoequatorial C6 unit and a pseudoaxial methyl group, probably favors over transition state B, producing a less stable product with the opposite stereochemistry at the quaternary center.
Although the 11-membered ring was diastereoselectively constructed, the Z-geometry of the trisubstituted olefin in 9 has to be converted to E-geometry. First, the alcohol 9 was protected with TBDMSCl, before the reduction of both carbonyl groups with LiAlH4 to give rise to the diol 10, which was then mesylated (Scheme 4). The resultant dimesylate was subjected to elimination of the secondary mesylate under basic conditions, giving rise to the monomesylate 11 in a moderate yield over three steps. Subsequent reductive demesylation of 11 was successfully achieved using a NaBH4-DMPU system, resulting in the formation of 12 in good yield. The aldehyde 13, which was derived from 12, was treated with AIBN and thiophenol to produce the desired E-olefin 14. Finally, the conjugate aldehyde in 14 was reduced by the Luche protocol to afford 3a. The 1H NMR of 3a, however, was not identical to that of natural vibsanin F (3). Thus, each epoxide ring of the synthetic product 3a and vibsanin F was reduced with LiAlH4, resulting in the preparation of the same diol 15. All the spectroscopic data for both diols were identical to each other, and therefore, the absolute configuration of vibsanin F has been established to be 3b with 6R, 7S, and 11S forms.
2.3. 11-Membered ring vibsane-type diterpenoids
Vibsane-type diterpenoids consist of three sub-types, 11-membered ring, 7-membered ring, and the rearranged types. These diterpenoids occur exclusively in V. awabuki, V. odratissimum, V. suspensum, and V. sieboldi. The 11-membered ring and 7-membered ring vibsane-type diterpenoids are common to these plants. Most 11-membered ring vibsanins consist of a fumulane-like skeleton containing a β, β-dimethylacrylate group at the C-8 position except for vibsanin F (3). Vibsanin A (17)7 and vibsanins P (18) – T (22),15 which contain oxidatively modified C6 units at the C-11 position, were isolated from both Japanese and Taiwanese V. odoratissimum.
Since the conformations of the 11-membered ring vibsanins A and P – T, which bear a 6,7-epoxide ring, are fixed, analysis of their NMR can be performed normally. On the other hand, vibsanin B (1) and vibsanols A (23) and B (24),16 which contain a cross-conjugated diene, show complex NMR signals due to the presence of several kinds of conformational isomers. Therefore, careful structure elucidation should be performed by a combination of VT NMR experiments and MM2 calculations.11
2.4. 7-Membered ring vibsane-type diterpenoids
The 7-membered ring vibsanins are made up of a diverse range of compounds, which possess two ketones and an isoprene unit attached to the 7-membered ring. There are two basic types, the (5S,10R) and (5R,10R) stereoisomers. Vibsanins C (2), G (25), H (27), and 18-O-methylvibsanin G (26), which belong to the (5S,10R) type, occur commonly in Viburnum species.17, 18 Vibsanins I (30), J (31), K (32), and 18-O-methylvibsanin K (33)19 as well as 14,15-epoxyvibsanin C (34) were isolated from V. awabuki in Tokushima17,19 and V. awabuki in Taiwan, respectively. On the other hand, 5-epi-vibsanins C (35), H (36), I (38), K (39) and their 18-O-methyl and/or 15-O-methyl congeners 37 and 4020 have been found in all Viburnum species except for V. suspensum. Vibsanin M (41), which possesses a Δ4,5 double bond18 and the bicyclic vibsanin N (46)21 were isolated from V. odoratissimum and V. awabuki in Taiwan. Furthermore, aldovibsanins A (42) – C (45) were isolated from V. odoratissimum.22, 18
Additionally, interesting tricyclic 7-membered vibsanins are known (Figure 10). Vibsanin E (16) and 16-hydroxyvibsanin E (47),23 which were isolated from V. awabuki, have an ether bond between C-15 and C-18; whereas, cyclovibsanin A (48) and 15-O-methylcyclovibsanin A (49), 15-O-methylcyclovibsanin B (50), and 3-hydroxy-15-O-methylcyclovibsanin A (51), which are composed of a tricyclo[6.3.2.00,0]tridecane skeleton, contain C-C bonds between C-18 and C-16 or C-17. Cyclovibsanins have no oxygen atoms between C-18 and C-15.24
Vibsanin E (16) can be readily converted from vibsanin C (2) via a cationic process (a) by treating it with BF3OEt2 as shown in Scheme 5.23 On the other hand, another plausible biosynthetic pathway for cyclovibsanins is (b) shown in Scheme 5, in which a proton is eliminated from one of two methyl groups in 52 to give rise to 52a. Dehydration produces the exomethylene ketone 52b, the C-4 carbonyl group of which is then protonated to trigger cyclization through the cationic intermediate 52b, resulting in the formation of a tricyclic framework such as that seen in the cyclovibsanins.
Furanovibsanins, a diverse range of 7-membered ring vibsanins, which are presumed to be produced by two ketones at the C-4 and C-7 positions, have also been found (Figure 11). The examples shown are furanovibsanin A (53) and its 3-O-methyl congener 54, which is derived from 3-hydroxyvibsanin E (47). The additional examples are furanovibsanin B (55) and its 7-epimer 56, and furanovibsanins C (57) – G (61). These diterpenoids were isolated from V. awabuki collected in Tokushima.25
2.5. Rearranged vibsane-type diterepenoids (neovibsanins)
The rearranged vibsane-type diterpenoids (neovibsanins), which contain a β, β-dimethylacrylate ester and an isoprene unit-substituted cyclohexene ring core fused to a tetrahydrofuran ring, are rarely occurring natural products (Figure 12). In 1996, the first rearranged vibsane-type diterpenoids, neovibsanins A (62) and B (63), were isolated from V. awabuki.10 Recently, we have ascertained the presence of neovibsanin (86) in the fresh leaves of V. awabuki and also found that neovibsanin is changed to neovibsanins A (62) and B (63) when kept in methanol at room temperature, indicating that neovibsanins A and B are artifacts derived from neovibsanin.19 Since neovibsanins A (62) and B (63) were reported, a number of rearranged vibsane-type diterpenoids have been found in V. awabuki, V. suspensum, and V. sieboldi, and have become known as a characteristic compound group of the Viburnum species as summarized in Figure 12.
Among them, neovibsanin C (64) is the first example of a natural product with a macrocyclic structure formed through an endo-peroxide group.26 The unusual structure of 64 was established by converting it from neovibsanin B (63) as outlined in Scheme 6. The reduction of 64 with Zn in EtOH-AcOH resulted in
the formation of the diol 83, which was treated with methanol under acidic conditions to give rise to the methyl acetal 84. The acetal 84 was also derived from neovibsanin B (63) by photosensitized oxidation, followed by reduction of the formed peroxy group [7-epi-neovibsanin D (66)]. In addition, when 66 was treated with pTsOH in anhydrous benzene, 64 was generated in good yield, presumably by an acetal exchange reaction. Thus, the structure of neovibsanin C (64) including its absolute configuration was established.
Neovibsanins H (67) and I (69),28 and their 2-O-methyl congeners 68 and 70 are neovibsanins that do not possess an acetal group. Similar compounds containing an additional 6-membered ring [neovibsanins F (71) and its 14-epimer 73, and 14-epi-18-oxoneovibsanin F (74)] were isolated from V. suspensum.28
Spirovibsanin A (82) is the first example of a nor-vibsane-type diterpenoid.29 Recently, neovibsanins J (79), K (80), and P (81), in which two hydroxy groups at the C-4 and C-18 positions are involved in acetal formation on the C-7 carbonyl, were found.30
It has been shown that a 7-membered ring vibsanin C (2) can be derived from vibsanin B (1), an 11-membered ring vibsane-type diterpenoid, by thermal oxy-Cope rearrangement. However, no formation of neovibsanins was observed even upon heating 1 under acidic or basic conditions. It should be noted that neovibsanins A (62) and B (63) can be produced from vibsanin B (1) by photochemical reaction. Surprisingly, irradiation of vibsanin B (1) in benzene with a high pressure Hg lamp afforded 87, which possesses a neovibsanin-framework, in 4% yield, along with 85 (18%) and 2c (27%) (Scheme 7).10 These compounds have been neither found in natural sources. When the photochemical reaction of 1 was carried out in MeOH, neovibsanins A (62) and B (63) were produced in 12% and 20% yields, respectively, in addition to 88 (9%) and 89 (8%) as over-reacted products.27 The generation of 88 and 89 was presumably due to a series of fragmentation and cationic cyclizations triggered by the methanolysis of a β,β-dimethylacryl ester group.27 Furthermore, irradiation of 1 in 50% aqueous MeOH for 1 h directly yielded neovibsanin (86). This photochemical reaction of 1 forces the E/Z isomerization of the double bond at C-5 to generate (5Z)-vibsanin B (85). The MM2 calculations for 85 and 1, in which the C12–C17 side chain was replaced with a t-butyl group, were performed using MacroModel 31 and provided the most stable conformers, 1-(5Z) and 1-(5E), for each molecule as shown in Figure 13. In the case of 1-(5Z), the distance between the C-4 carbonyl and the C-7 OH is 1.65 Å, whereas 1-(5E) has a distance of 4.98 Å as depicted in Figure 13. This means that a 1,7-hydrogen shift from the OH group at C-7 to the carbonyl at C-4 occurs in 1-(5Z), but not in 1-(5E). This hydrogen shift not only causes a breakage of the C-7/C-8 bond as well as cyclization between C-10 and C-4, leading to the neovibsanin-framework, but also undergoes another oxy-Cope rearrangement via 1a to give rise to a 7-membered ring (8Z)-10-epi-vibsanin C (86), which is not formed by the thermal oxy-Cope rearrangement of vibsanin B (1).
Taking the aforementioned results into consideration, we wish to propose plausible biosynthetic mechanisms for neovibsanins, as outlined in Scheme 8. The biosynthesis of all neovibsanins starts from the key intermediate A, which is derived from vibsanin B (1). The C-18 hydroxy group undergoes 1,4-addition to yield B. In the case of route a, a hemiacetal E is generated, leading to neovibsanins A–D. Likewise, route b gives the intermediate allyl cation C prior to dehydration, which is trapped by some nucleophiles such as water to afford neovibsanins H–I (route c) and causes cationic cyclization to give D, resulting in the formation of neovibsanins F–G (route d). Additionally, neovibsanins J–K are presumably converted from the key intermediate A via the production of a bicyclic acetal of the C-7 carbonyl containing C-18 and C-4 hydroxy groups.30 However, a question still remains about how the key intermediate A is generated from vibsanin B (1) by enzyme-catalyzed reaction.
3. Biological activities of vibsane-type diterpenoids
The leaves of V. awabuki have been used as a fish poison for catching fishes in Okinawa and Southeast Asia for a long time. In 1980, Kawazu reported the first isolation of a piscicidal compound, vibsanin A, and a plant growth inhibitor, vibsanin B (1).7 Later, many vibsane-type diterpenoids were reported, among which some diterpenoids such as vibsanin C (2), 5-epi-vibsanin C (35),20 vibsanol A (23),16 and vibsanins K (32) and P (18)18, 32 exhibited significant and/or moderate cytotoxicity against tumor cells. Additionally, vibsanin B (1) and neovibsanin F (71) showed moderate toxic activity in a brine shrimp lethality assay.28
3.1 Neurotrophic activities of neovibsanins
A remarkable pathological symptom of Alzheimer’s disease (AD) is the loss of neuronal cells in the brain. Correspondingly, the overall strategy for treatment of AD is to prevent neuronal death or to produce new neuronal cells in the degenerative regions. Neurotrophins, such as nerve growth factor (NGF), brain-derived neurotrophic factor (BDNF), neurotrophin 3 (NT-3) and glial cell line-derived neurotrophic factor (GDNF), are recognized as important regulatory substances in the nervous system.33 Thus, neurotrophins are expected to have therapeutic efficacy for the treatment of AD. However, they can not cross brain-blood barrier because of the properties of its high molecular polypeptide and are easily metabolized by peptidases under physiological conditions.34 To address this issue, considerable efforts have been made to find small molecules that have neurotrophic properties or are capable of enhancing the action of NGF in appropriate cell populations.35 Rat pheochromocytoma (PC12) cells have been used as a good in vitro model of neuronal differentiation. After stimulation with NGF, PC12 cells differentiate to extend neurites and develop the characteristics of sympathetic neurons.40 For example, two iridoids, picrosides I and II34 and some clerodane-type diterpenoids, 6α,7α-dihydroxyannonene, and 7α,20-dihydroxyannonene37 have been demonstrated to show neurite outgrowth-promoting activity in NGF-mediated PC12 cells. Also some synthetic compounds, N-benzyloxycarbonyl-Leu-Leu-leucinal (ZLLLal),38 AIT-082,39 SR57746,40 and Aroclor 1254,41 have been reported to accelerate the action of NGF in PC12 cells. Recently, we have found that neovibsanin (86), and neovibsanins A (62) and B (63) have neurotrophic properties. Namely, they promote neurite outgrowth of NGF-mediated PC12 cells at concentrations ranging from 10 µM to 40 µM.42,43
Evaluation was carried out for PC12 cells neurite outgrowth according to a previously reported experiment procedure.40,44 As shown in Figure 14, neovibsanin (B), neovibsanin A (C) and neovibsanin B (D) significantly promoted neurite outgrowth from NGF (10 ng/mL)-treated PC12 cells at 40 µM. Among three compounds, neovibsanin A seems less potent than neovibsanin and neovibsanin B. However, all of them had no effect on morphology of PC12 cells in the absence of NGF. Additionally, other vibsane-type diterpenoids such as 7-membered and 11-membered ring subtypes have not been so far found to show neurotrophic properties in PC12 cells.
Quantitative analysis of the percentage of cells with neurites and the neurite length extending from the cell bodies (Figure 15) showed that neovibsanin (86) and neovibsainin A (62) significantly increased the percentage of PC12 cells bearing neurites and the neurite length compared with those of NGF-mediated PC12 cells at concentrations ranging from 5 to 40 µM, and, in the degree of activity, 62 (Figure 15, C and D) was likely to be less that 86 (Figure 15, A and B). On the other hand neovibsanin B (63) promoted efficiently the neurite outgrowth from of NGF-mediated PC12 cells in a dose-dependent manner at concentrations from 10 to 40 µM (Figure 15, E and F). In comparison of the percentage of cells with neurites and the average neurite lengths (Figure 16), neovibsanin B (63) seemed to be a more potent NGF-potentiator among three compounds. This result consists with morphological evaluation. It is assumed from these results that a stereochemistry on the acetal carbon may be related with affecting neurite outgrowth activity.
4. Synthesis of neovibsanin B
These unique molecular architectures and significant biological activities have strongly motivated organic chemists to devote their efforts to the syntheses of the vibsane-type diterpenoids. So far, the vibsane-type diterpenoids whose total syntheses have been achieved are only three molecules, i.e. (±)-2-O-methylneovibsanin H (68),45 (±)-neovibsanin B (63),46 and (±)-vibsanin E (16).47 From a synthetic point of view, the stereocontrol in the successive stereogenic centers involved in these diterpenoids has been challenging. Williams’s pioneering synthetic studies have addressed the issue of diastereoselective creation of the stereocenters involved in vibsane natural products. In fact, through many approaches toward the total syntheses of these molecules, the synthetic efforts have resulted in formation of diastereomers of the natual products (Figure 17), i.e. (6S)-vibsanin F (3a),12 (±)-5,10-bis-epi-vibsanin E (16a),48 (±)-5,14-bis-epi-spirovibsanin A (82a)49, and (±)-4,5-bis-epi-neovibsanins A (62a) and B (63a).50
This review is focused on the synthesis of neovibsanin B (63), which is not only a significant neurotrophic mimic but also the most challenging molecule among vibsane natural products.
In 2009, Imagawa and Nishizawa reported the first synthesis of (±)-neovibsanin B (63).46 They employed an intramolecular Diels-Alder reaction of 91 at 200˚C in dimethyl imidazolidinone (DMI) as a solvent for constructing the core cyclohexenone ring of neovibsanin, leading to a mixture of 92a and 92b (9:1) in 58% yield. DMI plays a crucial role in accelerating this reaction rate and thus makes it suitable for scale-up. Further manipulation of Diels-Alder adducts 92a and 92b involves introduction of a hydroxymethyl group by Baylis-Hillman reaction with formaldehyde to provide the key intermediate 95 (Scheme 9). It should be noted that 1,2-addition of nucleophiles to the C-4 ketone was dominated by addition of the less hindered undesired face to afford exclusively products having the undesired stereochemical disposition at C-4.45,50,55 Imagawa and Nishizawa overcame this steric drawback by devising tactic of using the oxygen of 2,4-dimethoxybenzyl (2,4-DMPM) group at C-10 to coordinate with the organolithium reagent and deliver the propargyl group from the same face. Reaction of excess lithio ethylpropiolate 96 with a toluene solution of 95 at -78˚C successfully proceeded to give rise to the
adduct 97 with the correct stereochemistry in 87% yield as a single diastereomer. 1,2-Addition of 96 to the C-4 ketone in 95 was completely controlled by the coordination of the two oxygen atoms of 2,4-DMPM group with 96, which is shown in Figure 18, to give 97 having the desired β-configuration at C-4. The triple bond of 97 was reduced with Red-Al to the α,β-unsaturated ester 98, which was in turn treated with TBAF for the deprotection of the TBS group, thereby triggering the subsequent Michael addition and lactonization to give rise to a tricyclic lactone 99 having the desired stereochemistry in good yield. The Deprotection of 2,4-DMPM in 99 by DDQ oxidation was troublesome due to the acidity of in situ formed DDQH. To avoid acidic conditions, this oxidation was carried out in a two phase system of CH2Cl2 and NaCl saturated-phosphate buffer to give a desired alcohol, which was protected again as the TBS group, giving rise to 100 in good yield. The resulting 100 was reacted with Tebbe reagent, and then treated with PPTS in methanol afforded a mixture of 102a and 102b (1:4.5), which were readily separated by HPLC. The major 102b was converted to the aldehyde 103, which was treated with KHMDS to generate a potassium enolate. This was in situ trapped with 3,3-dimethylacryloyl chloride completing the first total synthesis of (±)-neovibsanin B (63).
Recently, we reported efficient construction of the chiral all-carbon quaternary center with a vinyl moiety that would permit post-functional group manipulation by the conjugate addition of lithium divinyl cuprate to (4S,2´E)-3-(6´-TBDPS-3´-methylhex-2´-enoyl)-4-phenyloxazolidin-2-one (115), and demonstrate that this method provides a versatile chiral quaternary carbon source 117 for the synthesis of natural products by its use to the synthesis of (+)-bakuchiol (Scheme 11).51
We have decided to apply this methodology to create the C-11 chiral quaternary carbon involved in vibsane natural products. As the first target, we selected the Imagawa-Nishizawa intermediate 95 that is the core framework of neovibsanin natural products. The asymmetric 1,4-additition reaction of (CH2=CH)2Cu(CN)Li2 to 118 bearing (R)-4-phenyl-2-oxazolidinone was employed to give 119 as a diastereomeric mixture of 95 (11S):5 (11R) in good yield. Each diastereomer was readily separated by silica-gel chromatography. The optically pure (11S)-119 was converted to (2Z,11S)-122 according to the procedures outlined in Scheme 12.
Previously, we reported that the modified Negishi palladium(0)-catalyzed carbonylative cyclization of (±)-122 provided the cyclohexene-1-one derivative (±)-125, which corresponds to the cyclohexene ring of neovibsanin.52 We examined this reaction in detail (Table 1). First, the reaction was performed using 5 mol % PdCl2(PPh3)2 and Et3N (1.5 equiv) in MeCN/ PhH (1:1) containing 4 equiv of MeOH at 100˚C in an autoclave, which gave rise to the desired diastereomeric mixture of 123 in 11% yield along with ca. 50% of 125 containing a small amount of 124 (6%) (Table 1, entry 1). On the other hand, the addition of an excess amount (48 equiv) of MeOH to this reaction system dramatically increased the yield of 123 to 54%, contaminated with 2% of the noncyclic ester 124 (entry 2). The use of high pressure (8 MPa) was found to be ineffective at suppressing the generation of 124 (entry 3), but low temperature (60˚C) was able to decrease the generation of 124 (entry 4). After several trials, we found that the following reaction
conditions; 24 equiv of MeOH, 4 MPa CO, temperature of 60˚C, led to the formation of 123 alone in ca. 70% yield as a diastereomeric mixture (10R:10S = 2.6:1) (entry 5). Each diastereomer of 123 was readily separated by silica gel column chromatography. It should be noted that 125 was exclusively generated in high yield when 1,2-dioxane was used as solvent (entry 6).
With (10R,11S)-123 in hand, we focused on the last few steps for the synthesis of Imagawa-Nishizawa’s intermediate 95 (Scheme 13). Treatment of (10R,11S)-123 with n-Bu4NF containing acetic acid gave 126, and the resultant hydroxy group was oxidized by Swern oxidation to its aldehyde, which was subjected to Wittig olefination to give the dimethyl olefin 127 in 69% yield over two steps. Reduction of 127 with DIBAL-H provided the cyclic hemiacetal 128 and diol 129 in 29 and 26% yields, respectively. The cyclic hemiacetal 128 was reduced with NaBH4 to give 129.
CONCLUSION
Since Prof. Kawazu’s first report in 1980, over 80 vibsane-type diterpenoids have been found, and the new term of “Vibsane” has now become accepted. These diterpenoids not only show a rich structural diversity, but also occur specifically in a few Viburnum species. Thus, they are very interesting natural products from chemical and taxonomic points of view, and moreover, their chemical diversity makes them a valuable chemical library. As neovibsanins A and B have been found to exhibit interesting neurotrophic activity, it is expected that new biological activities will be discovered among the vibsane-type diterpenoids. Recently, vibsane-type diterpenoids have attracted much attention from organic chemists due to their unique structures and important biological activities.54-60 We hope that this review has stimulated much attention in synthetic studies of these diterpenoids and that comprehensive reviews of synthetic studies of the vibsane-type diterpenoids will appear in the near future.
ACKNOWLEDGEMENTS
We thank our colleagues for their dedication toward these vibsane projects, whose names are listed in the literature cited in references. These works were supported by Grant-in-Aids for Scientific Research from the Ministry of Education, Culture, Sports, and Technology of Japan and the Open Research Fund from the Promotion and Mutual Aid Corporation for Private School of Japan.
References
1. R. C. Winkworth, Am. J. Botany, 2005, 92, 653. CrossRef
2. M. Fukuoka and M. Hotta, “Useful Plants of the World”, ed. by M. Hotta, K. Ogata, A. Nitta, K.
Hosikawa, M. Yanagi, and Yamazaki, K., Hebonsha, Tokyo, 1989, 1089.
3. A. M. Schevallier, “The Encyclopedia of Medicinal Plants”, ed. by D. Kinddersly, London, 1966, 148.
4. J. L. Swerdlow, “Nature’s Medicine”, National Geographic Society, New York, 2000, 388.
5. L. Q. Wang, Y. G. Chen, J. J. Xu, Y. L. Lin, X. M. Li, and Y. Zhao, Chemistry & Biodiversity, 2008, 5, 1879. CrossRef
6. Y. H. Kuo, the Formosan Science, 1992, 45, 99.
7. K. Kawazu, Agri. Biol. Chem., 1980, 44, 1367. CrossRef
8. K. Fukuyama, Y. Katsube, and K. Kawazu, J. Chem. Soc., Perkin Trans., 1980, 44.
9. J. R. Hanson, Nat. Prod. Rep., 2000, 17, 165. CrossRef
10. Y. Fukuyama, H. Minami, K. Takeuchi, M. Kodama, and K. Kawazu, Tetrahedron Lett., 1996, 37, 6767. CrossRef
11. Y. Fukuyama, H. Minami, S. Takaoka, M. Kodama, K. Kawazu, and H. Nemoto, Tetrahedron Lett., 1997, 38, 1435. CrossRef
12. H. Yuasa, G. Makado, and Y. Fukuyama, Tetrahedron Lett., 2003, 44, 6235. CrossRef
13. Y. Gao, R. M. Hanson, J. M. Klunder, S. Y. Ko, H. Masamune, and K. B. Sharpless, J. Am. Chem. Soc., 1987, 109, 5765. CrossRef
14. J. Tsuji, “Handbook of Organopalladium Chemistry for Organic Synthesis”, ed. by E. Negishi and A. de. Meijere, John Wiely & Sons, Inc., New York, 2002, Vol. 2, 39.
15. A. H. El-Gamal, S-K. Wang, and C-Y. Duh, J. Nat. Prod., 2004, 67, 333. CrossRef
16. Y.-H. Shen, C. V. S. Prakash, L.-T. Wang, C.-T. Chien, and C.-H. Hung, J. Nat. Prod., 2002, 65, 1052. CrossRef
17. H. Minami, S. Anzaki, M. Kubo, M. Kodama, K. Kawazu, and Y. Fukuyama, Chem. Pharm. Bull., 1998, 46, 1194. CrossRef
18. Y.-C. Shen, C.-L. Lin, S.-C. Chien, A. T. Khalil, C.-L. Ko, and C.-H. Wang, J. Nat. Prod., 2004, 67, 74. CrossRef
19. Y. Fukuyama, unpublished data.
20. Y. Fukuyama, H. Minami, A. Matsuo, M. Kitamura, M. Akizuki, M. Kubo, and M. Kodama, Chem. Pharm. Bull., 2002, 50, 368. CrossRef
21. C.-Y. Duh, A. H. El-Gamal, and S.-K. Wang, Tetrahedron Lett., 2003, 44, 9321. CrossRef
22. M. Kubo, I.-S. Chen, H. Minami, and Y. Fukuyama, Chem. Pharm. Bull., 1999, 47, 295. CrossRef
23. Y. Fukuyama, H. Minami, M. Kagawa, M. Kodama, and K. Kawazu, J. Nat. Prod., 1999, 62, 377. CrossRef
24. Y. Fukuyama, M. Morisaki, Y. Minoshima, H. Minami, Y. Takahashi, and Y. Asakawa, Lett. Org. Chem., 2004, 1, 189. CrossRef
25. Y. Fukuyama, M. Kubo, T. Fuji, A. Matsuo, Y. Minoshima, H. Minami, and M. Morisaki, Tetrahedron, 2002, 58, 10033. CrossRef
26. M. Kubo, H. Minami, E. Hayashi, M. Kodama, K. Kawazu, and Y. Fukuyama, Tetrahedron Lett., 1999, 40, 6261. CrossRef
27. Y. Fukuyama, M. Kubo, H. Minami, H. Yuasa, A. Matsuo, T. Fujii, M. Morisaki, and K. Harada, Chem. Pharm. Bull., 2005, 53, 72. CrossRef
28. Y. Fukuyama, H. Fujii, H. Minami, H. Takahashi, and M. Kubo, J. Nat. Prod., 2006, 69, 1098. CrossRef
29. M. Kubo, T. Fujii, H. Hioki, M. Tanaka, Y. Kawazu, and Y. Fukuyama, Tetrahedron Lett., 2001, 42, 1081. CrossRef
30. M. Kubo, Y. Minoshima, D. Arimoto, H. Minami, K. Harada, H. Hioki, and Y. Fukuyama, Heterocycles, 2009, 77, 539. CrossRef
31. F. Mohamadi, N. G. Richards, E. C. Guida, R. Liskamp, M. Lipton, C. Cafui, G. Chang, T. Hendrickson, and W. C. Still, J. Comput. Chem., 1990, 11, 440. CrossRef
32. Y. C. Shen, C. V. S. Prakasha, L. T. Wang, and C. T. Chien, J. Chin. Chem. Soc., 2003, 50, 297.
33. W. M. Cowan, Annu. Rev. Neurosci., 2001, 24, 551. CrossRef
34. P. Li, K. Matsunaga, T. Yamakuni, and Y. Ohizumi, Eur. J. Pharmacol., 2000, 406, 203. CrossRef
35. R. D. Brinton and R. S. Yamazaki, Pharm. Res., 1998, 15, 386. CrossRef
36. L. A. Greene and A. S. Tischler, Proc. Natl. Acad. Sci. U. S. A., 1976, 73, 2424. CrossRef
37. W. Tang, M. Kubo, K. Harada, H. Hioki, and Y. Fukuyama, Bioorg. Med. Chem. Lett., 2009, 19, 882. CrossRef
38. (a) S. Tsubuki, H. Kawasaki, Y. Saito, N. Miyashita, M. Inomata, and S. Kawashima, Biochem. Biophys. Res. Commun., 1993, 196, 1195; CrossRef (b) S. Tsubuki, Y. Saito, M. Tomioka, H. Ito, and S. Kawashima, J. Biochem., 1996, 119, 572.
39. P. J. Middlemiss, A. J. Glasky, M. P. Rathbone, E. Werstuik, S. Hindley, and J. Gysbers, Neurosci. Lett., 1995, 199, 131. CrossRef
40. A. Pradines, M. Magazin, P. Schiltz, G. L. Fur, D. Caput, and P. Ferrara, J. Neurochem., 1995, 64, 1954. CrossRef
41. W. G. R. Angus and M. L. Contreras, Neurosci. Lett., 1995, 191, 23. CrossRef
42. Y. Kishimoto, S. Maeda, and Y. Fukuyama, The 126th Annual Meeting of Pharmaceutical Society of Japan, Sendai, 2006.
43. M. Kubo, Y. Kishimoto, K. Harada, H. Hioki, and Y. Fukuyama, Bioorg. Med. Chem. Lett., 2010, 20, 2566. CrossRef
44. D. Vaudry, P. J. S. Stork, P. Lazarovici, and L. E. Eiden, Science, 2002, 296, 1648. CrossRef
45. A. P.-J. Chen and C. M. Williams, Org. Lett., 2008, 10, 3441. CrossRef
46. H. Imagawa, H. Saijo, T. Kurisaki, H. Yamamoto, M. Kubo, Y. Fukuyama, and M. Nishizawa, Org. Lett., 2009, 11, 1253. CrossRef
47. B. D. Schwartz, J. R. Denton, H. M. L. Davis, and C. M. Williams, Aust. J. Chem., 2009, 62, 980. CrossRef
48. H. M. L. Davis, Ø. Loe, and D. G. Stafford, Org. Lett., 2005, 7, 5561. CrossRef
49. (a) M. J. Gallen and C. M. Williams, Org. Lett., 2008, 10, 713; CrossRef (b) M. J. Gallen and C. M. Williams, Eur. J. Org. Chem., 2008, 27, 4697. CrossRef
50. A. P.-J. Chen, C. C. Müller, H. M. Cooper, and C. M. Williams, Org. Lett., 2009, 11, 3758. CrossRef
51. T. Esumi, H. Shimizu, A. Kashiyama, C. Sasaki, M. Toyota, and Y. Fukuyama, Tetrahedron Lett., 2008, 49, 6846. CrossRef
52. T. Esumi, M. Zhao, T. Kawakami, M. Fukumoto, M. Toyota, and Y. Fukuyama, Tetrahedron Lett., 2008, 49, 2692. CrossRef
53. T. Esumi, T. Mori, M. Zhao, M. Toyota, and Y. Fukuyama, Org, Lett., 2010, 12, 888. CrossRef
54. B. D. Schwartz, J. R. Denton, Y. Lian, H. M. L. Davies, and C. M. Williams, J. Am. Chem. Soc., 2009, 131, 8329. CrossRef
55. G. Mehta and B. A. Bhat, Tetrahedron Lett., 2009, 50, 2474. CrossRef
56. J. Nikolai, Ø. Loe, P. M. Dominiak, O. O. Gerlitz, J. Autchbach, and H. M. L. Davis, J. Am. Chem. Soc., 2007, 129, 10763. CrossRef
57. A. Srikrishna, V. H. Paradeshi, and G. Satyanarayana, Tetrahedron: Asymmmetry, 2008, 19, 1984. CrossRef
58. B. D. Schwartz, C. M. Williams, and P. V. Bemhardt, Beilstein J. Org. Chem., 2008, 4, 34. CrossRef
59. B. D. Schwartz, J. R. Denton, Y. Lian, H. M. L. Davis, and C. M. Williams, J. Am. Chem. Soc., 2009, 131, 8329. CrossRef
60. B. D. Schwartz, J. R. Denton, P. V. Bernhardt, H. M. L. Davis, and C. M. Williams, Synthesis, 2009, 2840. CrossRef