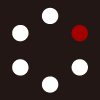
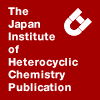
HETEROCYCLES
An International Journal for Reviews and Communications in Heterocyclic ChemistryWeb Edition ISSN: 1881-0942
Published online by The Japan Institute of Heterocyclic Chemistry
e-Journal
Full Text HTML
Received, 6th April, 2010, Accepted, 6th May, 2010, Published online, 7th May, 2010.
DOI: 10.3987/REV-10-672
■ Synthesis of Nitrogen- and Oxygen-Bridged Seven- to Ten-Membered Carbocycles Using Metathesis Reactions
Ken-ichi Takao* and Kin-ichi Tadano*
Department of Applied Chemistry, Faculty of Science and Technology, Keio University, Hiyoshi, Kohoku-ku Yokohama 223-8522, Japan
Abstract
This review describes the synthesis of nitrogen- and oxygen-bridged seven- to ten-membered carbocycles using metathesis reactions and their applications to natural products synthesis achieved in the past decade.Contents
1. Introduction
2. Synthesis of nitrogen-bridged seven- to ten-membered cycloalkenes
2.1. Synthesis of azabicylo[n.3.1]alkenes
2.2. Total synthesis of (–)-adaline
2.3. Synthesis of calystegine analogues
2.4. Total synthesis of (+)-anatoxin-a
2.5. Formal synthesis of (+)-anatoxin-a
2.6. Total synthesis of (+)-ferruginine
3. Synthesis of oxygen-bridged seven- to ten-membered cycloalkenes
3.1. Synthesis of oxabicyclo[4.2.1]nonenes
3.2. Synthesis of oxabicyclo[n.2.1]alkenes
3.3. Synthesis of calystegine analogue
4. Synthesis of oxygen-bridged cyclooctadiene derivatives
4.1. Total synthesis of (±)-mycoepoxydiene
4.2. Total synthesis of natural (+)-mycoepoxydiene
4.3. Total syntheses of (–)-1893A and (+)-1893B
5. Conclusions
6. References
1. INTRODUCTION
Metathesis is established as a remarkably valuable synthetic tool in current organic chemistry.1 The innovative development of the metathesis strategy has depended on refinements of the catalysts. Representative examples of commonly used metathesis catalysts are illustrated in Figure 1. The Schrock group produced the first efficient metal-catalyst for metathesis, i.e. molybdenum carbene complex (1), in 1990.2 After that Grubbs and co-workers developed an even more easy-to-use catalyst, the air- and moisture-tolerant ruthenium-based complex (2), which is known as the first-generation Grubbs catalyst.3 In 1999, the novel N-heterocyclic carbene-derived catalyst (3), the second-generation Grubbs catalyst, was found to be more active in olefin metathesis.4 In addition, the isopropoxystyrene-derived catalyst (4), a structurally resembling catalyst to 3, was developed by Hoveyda and co-workers.5 These catalysts (1–4), all commercially available at present, have been verified as efficient mediators for constructing a variety of cyclic olefins.
Organic compounds containing a medium-sized heterocycle are frequently found in nature and many of them exhibit interesting biological activities. The medium-sized rings are generally difficult to be constructed by traditional cyclization methodologies because of enthalpic and entropic factors.6 Therefore the direct construction of medium-sized rings is still a challenge subject in current organic synthesis. In 1992, Grubbs and Fu realized a breakthrough in the field of heterocycles synthesis featuring an olefin metathesis reaction; the ring-closing metathesis (RCM) reaction was applied to the formation of five- to seven-membered cyclic ethers using the Schrock catalyst (1).7 For example, the RCM reaction of diene (5) provided a seven-membered oxygen-containing heterocycle (6) in good yield (Scheme 1).
Since this landmark achievement, synthetic efforts directed toward the medium-sized heterocyclic compounds using metathesis reactions have been widely investigated.8 We have also been interested in developing a variety of metathesis approaches for the formation of medium-sized bridged bicyclic heterocycles, and their applications to natural product synthesis. This review summarizes (1) the synthesis of nitrogen-bridged seven- to ten-membered carbocycles using RCM of dienes and enynes (eqs 1 and 2 in Scheme 2), (2) the synthesis of oxygen-bridged seven- to ten-membered carbocycles using RCM of dienes (eq 3), and (3) the direct construction of oxygen-bridged cyclooctadiene using sequential metathesis, i.e., ring-opening/cross metathesis (ROCM) followed by RCM (eq 4). Most reports cited in this review are focused on the metathesis-based approaches aimed at natural products synthesis.
2. SYNTHESIS OF NITROGEN-BRIDGED SEVEN- TO TEN-MEMBERED CYCLOALKENES
2.1. Synthesis of Azabicyclo[n.3.1]alkenes
Nitrogen-bridged carbocycles such as the general structures 7–10 represent a unique class of alkaloids displaying intriguing biological profiles (Figure 2).9 The Martin group has developed a concise approach to the synthesis of azabicyclo[n.3.1]alkenes (n = 3, 4, 5) using the RCM reaction of cis-2,6-dialkenyl N-alkoxycarbonyl piperidine derivatives.10 This is the first example that employed RCM to prepare azabicyclo[n.3.1]alkanes with a nitrogen atom in the one-atom bridge.
The requisite cis-2,6-dialkenylpiperidines were prepared starting from glutarimide (11) (Scheme 3). Hydride reduction of 11 in the presence of acid, followed by treatment of the resultant δ-ethoxy δ-lactam with sodium benzenesulfinate, gave δ-sulfonyl δ-lactam (12), which was reacted with alkenyl Grignard reagents to provide lactams (13a–c, n = 0, 1, 2). Treatment of 13a–c with benzyl chloroformate (CbzCl) provided imides (14a–c). Hydride reduction of the lactam carbonyl group in 14a–c, followed by allylation of the resultant hemiaminals with allyltrimethylsilane in the presence of BF3·OEt2, furnished cis-2-allyl-6-alkenylpiperidines (15a–c) with high stereoselectivities (cis/trans = 16–20:1).
The dialkenyl piperidines (15a–c) underwent efficient RCM at room temperature in the presence of the first generation Grubbs catalyst (2) in dichloromethane to give the corresponding nitrogen-bridged cycloalkenes (16a–c) in 82–91% yield (Scheme 4). The N-Cbz group played an important role in these reactions. The cis-2,6-disubstituted N-Cbz piperidines are expected to exist in the chair conformer TS-B preferentially, wherein the substituents at the 2- and 6-positions are in axial orientations in order to avoid A1,3-strain with the N-Cbz group that is present in the alternate chair conformer TS-A.11 The two alkenyl groups in TS-B are thus properly disposed to undergo facile RCM to give the bicyclic alkenes (16a–c).
Bubnov and co-workers have also reported the analogous approach to nitrogen-bridged cycloalkenes from pyridine and pyrrole featuring the RCM reaction.12
2.2. Total Synthesis of (–)-Adaline
(–)-Adaline (17) is the major defensive alkaloid isolated from the European ladybug and possesses a nitrogen-bridged cyclooctanone skeleton (Figure 3).13 Kibayashi and co-workers have achieved the total synthesis of 17 via the RCM reaction of cis-2,6-dialkenylpiperidine.14
Condensation of the enantiomeric amine (18), as a chiral auxiliary, with δ-keto acid (19) gave tricyclic lactam (20), which was treated with allyltrimethylsilane in the presence of TiCl4 (Scheme 5). The diastereoselective addition of an allyl group to the in situ generated N-acyliminium ion provided 6,6-disubstituted 2-piperidone (21).15 Reductive lactam-ring opening of 21, followed by Ley oxidation of the resultant amino alcohol (22), afforded tricyclic N,O-acetal (23). Upon treatment of 23 with lithium acetylide, an SN2-like alkynylation proceeded with concomitant removal of the chiral auxiliary, leading to trisubstituted piperidine (24) as a single diastereomer. The hydrochloride salt of 24 was reacted with trimethylorthoformate to give the N-formyl derivative (25), which was converted into cis-2,6-dialkenylpiperidine (26) by hemihydrogenation using Lindlar catalyst.
The RCM reaction applied to 26 smoothly proceeded with 2 in benzene at 50 °C to give nitrogen-bridged cyclooctene (27) in 90% yield (Scheme 6). When the second-generation Grubbs catalyst (3) was employed, the reaction proceeded more efficiently to afford 27 in almost quantitative yield. On the other hand, the attempted RCM reaction of the hydrochloride salt (28),16 prepared from 24 by Lindlar reduction, failed to construct the bicyclic piperidine (29), otherwise resulting in recovery of the starting material. This failure is most probably due to diequatorial arrangement of the 2,6-dialkenyl substituents in the chair conformer of 28.
Dihydroxylation of 27 with osmium tetroxide, followed by regioselective protection of the resultant diol, provided mono-O-t-butyldimethylsilyl (TBS) ether (30) (Scheme 7). Transformation of 30 to 31 was performed by the Barton–McCombie radical deoxygenation procedure. Removal of the TBS and formyl protecting groups in 31 furnished amino alcohol (32), which was finally converted into (–)-adaline (17) by pyridinium chlorochromate (PCC) oxidation.
2.3. Synthesis of Calystegine Analogues
Calystegines (for example, 33–35) are nortropane alkaloids with three to five hydroxy groups in various positions of 8-azabicyclo[3.2.1]octane skeleton, and show inhibitory activities against several glycosidase enzymes (Figure 4).17 Among a number of calystegines isolated to date, calystegine B2 (34) is the most abundant in nature. Kaliappan and co-workers have synthesized several unnatural calystegine analogues as potential glycosidases inhibitors, relying on the RCM reaction for constructing the nitrogen-bridged cycloheptene structure.18
Addition of allylmagnesium chloride to D-mannose-derived nitrone (36) occurred stereoselectively from the convex face of the bicyclic structure, affording α-allylated N-OH pyrrolidine derivative (37) (Scheme 8). The N–O bond in 37 was cleaved with powdered zinc in the presence of a catalytic amount of indium. The resulting amine was protected as N-t-butoxycarbonyl (Boc) to provide carbamate (38). The O-isopropylidene group in the side chain of 38 was hydrolyzed selectively to give vicinal diol (39), which was converted into diene (40) by the reductive reaction with triphenylphosphine, iodine, and imidazole.
The cis-2-allyl-5-vinylpyrrolidine (40) was subjected to the RCM reaction with 2 in refluxing dichloromethane (Scheme 9). The expected nitrogen-bridged cycloheptene derivative (41) was obtained in an excellent yield of 95%.
The RCM product (41) was further utilized as an advance precursor for the syntheses of several analogues of calystegine. Dihydroxylation of 41 provided exo-diol (42) as a sole diastereomer (Scheme 10). Simultaneous cleavage of the acetonide and the N-Boc group was achieved with 6 M HCl to afford tetrahydroxylated azabicyclic compound (43) as the hydrochloride salt. In comparison to the naturally occurring calystegines, the resulting 43 could be categorized as an analogue of calystegine B (a nortropane alkaloid with four hydroxy groups). By using the similar synthetic route, other calystegine analogues having two to five hydroxy groups were also synthesized. Some of the synthetic analogues exhibited glycosidase inhibitory activity.
2.4. Total Synthesis of (+)-Anatoxin-a
The Martin group completed the total synthesis of (+)-anatoxin-a (44),19 isolated from the toxic blooms of the blue-green freshwater alga (Figure 5).20 In their total synthesis, enyne ring-closing metathesis was applied to construct the nitrogen-bridged cyclooctene structure.
The synthesis commenced with N-protection of methyl D-pyroglutamate (45) to provide the N-Cbz-γ-lactam (46) (Scheme 11). Addition of 3-butenyl Grignard reagent to 46 in the presence of tetramethyethylenediamine (TMEDA) afforded an α-amino acid derivative (47). Treatment of 47 with a premixed solution of triphenylsilane and BF3·OEt2 provided cis-2,5-disubstituted pyrrolidine (48) with high diastereoselectivity (cis/trans = 11:1). The bulky silane reagent provides a significant steric bias for the stereoselective reduction of the transient N-acyl iminium ion from the less-hindered face of the pyrrolidine ring. The methyl ester moiety of 48 was reduced with DIBALH, and the intermediate aldehyde was treated with Ohira–Bestmann reagent (49) to give acetylene (50). Methylation of the sodium acetylide generated from 50 afforded enyne (51), the substrate for enyne ring-closing metathesis.
The enyne (51) was exposed to 3 in dichloromethane at room temperature (Scheme 12). The key enyne ring-closing metathesis provided the desired nitrogen-bridged cyclooctene (52) cleanly in 87% yield.
Regioselective dihydroxylation of the less-substituted olefin in 52 with the complex of osmium tetroxide and triethylamine, followed by hydrolysis of the intermediate osmate ester, afforded the diol, which was subjected to oxidative cleavage to provide methyl ketone (53) (Scheme 13). The N-Cbz group in 53 was removed with iodotrimethylsilane, thus completing the total synthesis of (+)-anatoxin-a (44).
2.5. Formal Synthesis of (+)-Anatoxin-a
The Mori group has investigated extensively the RCM of enyne for construction of various ring-sized cyclic compounds, which was then utilized for natural product synthesis.21 They have successfully applied the developed enyne metathesis strategy to the formal synthesis of (+)-anatoxin-a (44).22
The known N-Boc protected γ-lactam (55, TBDPS = t-butyldiphenylsilyl), prepared from L-pyroglutamic acid (54), was reacted with 3-(benzyloxy)propyl Grignard reagent to give acyclic ketone (56) (Scheme 14). Deprotection of the Boc group with acid gave the cyclized imine (57). Stereoselective hydrogenation of 57, followed by N-tosylation and then deprotection of the benzyl group, afforded cis-2,5-disubstituted pyrrolidine derivative (58). Dess–Martin oxidation of 58 followed by Wittig reaction with Ph3P=CH2 provided terminal alkene (59). Deprotection of the TBDPS group in 59 followed by oxidation gave aldehyde, which was subjected to Corey–Fuchs dibromoolefination and subsequent treatment with n-butyllithium to afford terminal enyne (60).
As a preliminary study on enyne metathesis of terminal alkynes,23 the reaction of 60 was carried out using 2 in dichloromethane under ethylene gas (Scheme 15). However, the desired cyclized product (61) was obtained in only 15% yield along with 1,3-diene (62) in 13% yield. Although this RCM reaction was examined under various conditions (in the presence or absence of ethylene and using 2, 3 or 4 as a catalyst), the results were disappointing. This difficulty was overcome by protection of the terminal alkyne with a trimethylsilyl (TMS) group. Thus, the RCM reaction of the silylated alkyne (63) proceeded smoothly by use of 3 in refluxing dichloromethane under argon, giving nitrogen-bridged cyclooctene with a vinyl side chain, i.e. 61, as a result of the unexpected desilylation, in 85% yield.
Oxymercuration/reductive demercuration of 61, followed by Dess–Martin oxidation of the resultant secondary alcohol, provided the known N-tosylanatoxin-a (64) (Scheme 16). However, the [α]D value of the synthetic sample ((–)-64) possessed a levorotatory property, opposite to that of the expected enantiomer ((+)-64). This fact was explained as follows: the inversion of chirality took place during oxymercuration reaction. The authors rationalized the unusual inversion of chirality in terms of a stereospecific skeletal rearrangement of olefin-Hg complex (65) to allyl cation species (66). Subsequent nucleophilic attack of water to 66 resulted in the formation of 67, which was converted to (–)-64 by demercuration followed by oxidation. Compound (–)-64 had been already converted into (+)-anatoxin-a (44).24 Therefore, the formal synthesis of 44 was achieved.
2.6. Total Synthesis of (+)-Ferruginine
(+)-Ferruginine (69) is one of the tropane alkaloids, which possesses a nitrogen-bridged cycloheptane skeleton like the well-known cocaine (70), and shows the agonist activity against the nicotinic acetylcholine receptor (Figure 6).25 Aggarwal and co-workers have utilized the enyne RCM approach in their total synthesis of 69.26
The protected aminal (71) was prepared from L-pyroglutamic acid (54) by the known four-step procedure (Scheme 17). Treatment of 71 with allyltrimethylsilane in the presence of BF3·OEt2 gave the required cis-2,5-disubstituted pyrrolidine (72) as the major product (cis/trans = 80:20). Reduction of 72 followed by oxidation provided aldehyde (73), which was converted into terminal enyne (74) with the Ohira–Bestmann reagent.
The enyne RCM reaction of 74 with 2 in refluxing dichloromethane proceeded under an inert atmosphere, providing a nitrogen-bridged cycloheptene (75) in 86% yield (Scheme 18). In contrast, the use of 3 resulted in reduction of the yield of 75, presumably due to the occurrence of homodimerization of 75 during the reaction with the aid of the more active metathesis catalyst.
Wacker oxidation of 75 gave methyl ketone (76) (Scheme 19). Deprotection of the Boc group in 76, followed by reductive N-methylation of the resultant amine, completed the synthesis of (+)-ferruginine (69).
3. SYNTHESIS OF OXYGEN-BRIDGED SEVEN- TO TEN-MEMBERED CYCLOALKENES
3.1. Synthesis of Oxabicyclo[4.2.1]nonenes
Oxygen-bridged carbocycles are versatile synthetic intermediates, serving not only for the synthesis of natural product and medicinally important compounds, but also as templates and building blocks in organic synthesis.27 The Hanna group has demonstrated the utility of RCM in the formation of 9-oxabicyclo[4.2.1]nonenes.28 The addition of the vinyl lithium generated from 1,4-dioxene (77) to cyclohexanone gave allylic alcohol (78), which was reacted with 1-(trimethylsilyloxy)cyclopentene in the presence of trimethylsilyl triflate (TMSOTf) to furnish 2,3-disubstituted 1,4-dioxene (79) (Scheme 20).29 Oxidation of 79 with m-chloroperbenzoic aicd (m-CPBA) in methanol afforded methyl acetal–hemiacetal (80). Treatment of 80 with excess allyltrimethylsilane in the presence of TiCl4 led to the cis-2,5-diallylated tetrahydrofuran (82) via the monoallylated intermediate (81). By the same reaction sequence, a variety of diallyl compounds were also prepared.
The RCM reaction of 82 was carried out by use of 2 in dichloromethane at room temperature to provide 9-oxabicyclo[4.2.1]nonene (83) in 95% yield (Scheme 21). The bicyclic conformational constraint in 82 might facilitate the desired RCM reaction. Also other substrates smoothly underwent RCM, leading to the expected products in excellent yield.
3.2. Synthesis of Oxabicyclo[n.2.1]alkenes
The de Armas/Marrero-Tellado group has reported the synthesis of oxabicyclo[n.2.1]alkenes (n = 4, 5, 6) in enantiomerically pure forms starting from carbohydrate by using RCM reactions.30 The known epoxide (84, PMB = p-methoxybenzyl), prepared from D-mannose, was treated with vinylmagnesium chloride in the presence of copper iodide (Scheme 22). Subsequent acetylation of the resultant homoallyl alcohol gave acetate (85). Hydroboration of 85 followed by oxidative treatment provided primary alcohol (86). Hydrolysis and regioselective protection with pivaloyl chloride (PivCl), followed by removal of the PMB group at the anomeric position, gave hemiacetal (87). Treatment of 87 with iodosylbenzene and iodine resulted in oxidative β-fragmentation of the anomeric oxygen-centered radical,31 affording the formyl derivative (88). Partial hydrolysis of 88 and protection with a TBS group provided 89, which was subjected to the diastereoselective allylation with allyltrimethylsilane in the presence of BF3·OEt2 to give cis-2,5-disubstituted tetrahydrofuran (90). Protection of 90 as a TBS ether followed by DIBALH reduction gave alcohol (91). Conversion of 91 into 2,5-diallylated tetrahydrofuran (92) was performed by Grieco’s procedure. On the other hand, cis-2-allyl-5-(3-butenyl)tetrahydrofuran (93) was prepared from 91 by Swern oxidation followed by Wittig reaction. Another diene (94) was also synthesized from 91 in four steps.
After extensive experiments, treatment of diene (92) with 2 in refluxing benzene was found to be the best conditions, affording oxygen-bridged cyclooctene (95) in 64% yield (Scheme 23). Under the same conditions, oxygen-bridged cyclononene (96) and cyclodecene (97) were obtained from dienes (93) and (94), respectively. When each reaction was examined at room temperature or in refluxing dichloromethane, no formation of the corresponding cyclized product was observed.
3.3. Synthesis of Calystegine Analogue
Tamayo and co-workers have applied RCM reaction to construct an oxygen-bridged cycloheptene structure in the context of the synthesis of a calystegine analogue (for structures of calystegines, see Figure 4).32 The L-sorbose derivative (98) was subjected to Wittig reaction, affording the methylenated product (99) (Scheme 24). Acid hydrolysis of the 5,7-O-isopropylidene group in 99, followed by selective 7-O-protection with a TBDPS group, gave secondary alcohol (100). For the stereochemical inversion at C-5, 100 was oxidized to ketone by Ley oxidation and then reduced with NaBH4 to afford 7-O-silyl ether (101) as a single diastereomer. By a protection–deprotection sequence, 101 was converted into 5-O-benzyl ether (102). Dess–Martin oxidation of 102, followed by condensation of the resultant aldehyde with benzylhydroxylamine hydrochloride, provided nitrone (103). Treatment of 103 with vinylmagnesium bromide gave hydroxylamine (104) stereoselectively. Reduction of 104 with indium afforded amine (105), which was protected as carbamate (106).
The RCM reaction of 106 proceeded under microwave conditions (Scheme 25). A solution of 106 and a catalytic amount of 3 in dichloromethane was heated by irradiated microwave under argon in a sealed tube at 100 °C. After 2 h, the cyclized product (107) was obtained in 79% yield. On the other hand, the C-5 epimer of 106, i.e. 108, was prepared from 100 by the similar reaction sequence used for the conversion of 101 to 106. However, the RCM reaction of 108 did not provide the expected product (109), suggesting that the configuration at C-5 must be responsible to the failure of the RCM.
By treatment of 107 with palladium on carbon under an atmosphere of hydrogen, reduction of the carbon–carbon double bond and removal of the N-protecting groups took place to provide amine (110) (Scheme 26). When the hydrogenation of 107 was performed on a gram scale, the epimer (111) at the carbon bearing the amino group was also obtained in 14% yield. Exposure of 111 to hydrochloric acid resulted in hydrolysis of the acetonide, ring-opening of the bicyclic hemiketal (112), and intramolecular attack of the amino group to the carbonyl group in the resultant cycloheptanone (113) took place. Eventually, the triepimer of calystegine B2 as its O-benzyl derivative (114) was obtained. This is a rare example of a polyhydroxylated nortropanic ring with two hydroxy groups both in axial disposition. Unfortunately, attempts to remove the final benzyl group from 114 failed. On the other hand, acid hydrolysis of 110 gave a complex mixture.
4. SYNTHESIS OF OXYGEN-BRIDGED CYCLOOCTADIENE DERIVATIVES
4.1. Total Synthesis of (±)-Mycoepoxydiene
(+)-Mycoepoxydiene ((+)-115) is the first natural product that contains a 9-oxabicyclo[4.2.1]nona-2,4- diene structure (Figure 7).33 This unprecedented structure is thought to be of polyketide origin. Our group has accomplished the first total synthesis of (±)-115 by using a RCM strategy for constructing the oxygen-bridged cyclooctadiene core skeleton.34
The total synthesis started from the known tricyclic compound (116), the Diels–Alder adduct of furan and maleic anhydride (Scheme 27). Hydride reduction of the anhydride ring in 116, followed by monosilylation of the resultant meso-diol, gave mono-silyl ether (117). Conversion of 117 into the xanthate ester and subsequent dihydroxylation afforded diol (118) stereoselectively, which was subjected to the Barton–McCombie radical deoxygenation to provide highly functionalized oxanorbornane (119). Oxidative cleavage of the cis-diol in 119, followed by reduction of the resultant dialdehyde hydrate, provided a tetrasubstituted tetrahydrofuran derivative (120). The diol (120) was transformed into diiodide (121) via the corresponding ditosylate. Simultaneous substitution of the two iodo groups in 121 with each vinyl group was achieved by an excess amount of vinylmagnesium bromide, affording cis-2,5-diallylated tetrahydrofuran (122).
Treatment of 122 with 2 (four-time addition of each 5 mol% equiv of 2 over a period of 20 h) in refluxing benzene furnished the RCM product (123) in 83% yield (Scheme 28). When 20 mol% of 2 was added in one portion, the product (123) was obtained in a less-satisfactory yield of 41%. The use of 3 enabled the reduction of the catalyst-loading to 3 mol% and also the reduction of the reaction time to 6 h, with the formation of 123 in a slightly higher yield of 86%. The oxygen-bridged cyclooctadiene core skeleton in the target natural product was introduced into the oxygen-bridged cyclooctene (123). Thus, the addition of bromine to 123, followed by β-elimination of two-molar equivalents of hydrogen bromide from the resultant dibromide (124), provided the 9-oxabicyclo[4.2.1]nona-2,4-diene derivative (125), which was deprotected, providing the key synthetic intermediate (126).
The attachment of the δ-lactone moiety to the main scaffold was achieved as shown in Scheme 29. Dess–Martin oxidation of 126 gave the aldehyde, which was allowed to react with 2-lithiated furan to provide an inseparable mixture (ca. 3:2) of furfuryl alcohols (127 and 128). The desired diastereomer (127) could be obtained as a sole product by an oxidation–reduction of the diastereomeric mixture. Reduction of ketone (129) with L-Selectride proceeded presumably through the Li-chelation-assisted transition state occurring between the carbonyl group and the bridge-oxygen. The oxidative rearrangement of the furfuryl alcohol was realized by treatment of 127 with t-butyl hydroperoxide in the presence of a catalytic amount of vanadyl acetylacetonate (VO(acac)2), providing 2-pyranone (130). Protection of the hemiacetal hydroxy group in 130 as the TBS ether gave a mixture of the α-isomer (131) and the β-isomer (132). The major isomer (131) was subjected to Luche reduction and subsequent acetylation to afford allylic acetate (133) with a high level of diastereoselectivity. Finally, acid hydrolysis of the TBS group in 133, followed by oxidation of the resultant lactol, provided (±)-mycoepoxydiene ((±)-115). Through the same reaction steps used for the α-isomer (131), the β-isomer (132) was also converted into (±)-115.
4.2. Total Synthesis of Natural (+)-Mycoepoxydiene
In the progress directed toward the concise synthesis of the core skeleton (126) of mycoepoxydiene (115), we have developed a more concise methodology for the construction of the oxygen-bridged cyclooctadiene structure using sequential metathesis.35 Our second-generation approach to enantioenriched mycoepoxydiene ((+)-115) involves ring-opening/cross metathesis (ROCM) followed by RCM to construct the oxygen-bridged cyclooctadiene skeleton in a one-pot operation.
The synthesis of (+)-115, the natural enantiomer, began with the enzymatic hydrolysis of meso-diacetate (134) (Scheme 30). By treatment of 134 with lipase PS (Amano) in aqueous THF, enantioselective hydrolysis of one of the acetyl esters occurred effectively to provide monoacetate ((–)-135) with 95% ee. Silylation of (–)-135 followed by deacetylation afforded (–)-117. Tosylation of (–)-117 and subsequent reductive removal of the sulfonate in the resultant (–)-136 with sodium borohydride in N,N’-dimethylpropyleneurea (DMPU) led to an oxanorbornene derivative ((+)-137).
With use of 2 in benzene, the ROCM reaction of (+)-137 in the presence of 1,3-butadiene proceeded smoothly at room temperature to give an inseparable mixture of trienes (138a and 138b) in 60% yield with a favorable formation of the Z-isomers, which were stereochemically indispensable for the next ring-closing step (Scheme 31). In contrast, the catalyst 3 produced preferentially the undesired E-isomers in a diminished yield of 40%. This lower Z-selectivity was also observed when the reaction
was conducted in dihloromethane. The RCM reaction of the mixture of 138a and 138b occurred by using 3 in refluxing benzene, affording the oxygen-bridged cyclooctadiene compound ((+)-125) accompanied by inseparable and unidentified byproducts. By exposure of the mixture to tetrabutylammonium fluoride, homogeneous (+)-126 was obtained in a yield of 29% from 138ab after chromatographic purification on silica gel. On the other hand, the catalyst 2 did not activate the RCM reaction, and solvent did not influence this reaction. To construct the oxygen-bridged cyclooctadiene structure from the oxanorbornene derivative more concisely, the sequential ROCM/RCM reaction was performed in a one-pot process. Treatment of (+)-137 with 1,3-butadiene (2 equiv) in the presence of 2 (2
mol%) in benzene at room temperature initially produced trienes 138a and 138b. After (+)-137 was consumed, the reaction mixture was heated to reflux under bubbling argon to remove excess 1,3-butadiene. Then each 2 mol% of 3 was added in five portions over a period of 4 days. Desilylation of the reaction mixture provided the key intermediate ((+)-126) in a yield of 23% from (+)-137. Although the yield is moderate, this one-pot ROCM/RCM approach enabled us to cut seven steps in the synthetic route and to improve the overall yield of (+)-126.
From compound (+)-126, the similar reaction sequence used for the racemic total synthesis led to (+)-mycoepoxydiene ((+)-115) (Scheme 32). Through this enantioselective total synthesis of (+)-115, the absolute stereochemistry of natural mycoepoxydiene was established.
4.3. Total Syntheses of (–)-1893A and (+)-1893B
Soon after the completion of total synthesis of (±)-mycoepoxydiene ((±)-115),34 (–)-1893A (139) and (+)-1893B (140), which showed cytotoxic and insecticidal activities, were isolated from the fermentation broth of a marine endophytic fungus (Figure 8).36 These natural products (139 and 140) were found to possess the same oxygen-bridged cyclooctadiene core skeleton as in 115. We have synthesized 139 and 140 from the common intermediate ((+)-126).35,37
Oxidation of (+)-126 and subsequent vinylogous aldol reaction of the resulting aldehyde with 2-(trimethylsilyloxy)furan in the presence of triethylsilyl triflate (TESOTf) provided γ-adduct (141) as a mixture of stereoisomers (Scheme 33). The sulfonylation of 141 with mesyl chloride in pyridine followed by heating gave (–)-1893A (139) and its E-isomer (142) in a Z/E ratio of ca. 5:1. In addition, the minor product (142) was subjected to the same reaction conditions to afford additional 139. This result is reasonably attributable to a reversible conjugate addition of a nucleophilic species such as a chloride ion to δ-position followed by β-elimination, leading to 139.
The structure of 1893B was originally assigned to 149 as depicted in Scheme 34 by 1H-NMR spectral analysis.36 However, the stereochemistry of 1893B was envisaged to possess the same relative configuration as that of mycoepoxidiene (115), i.e. hence to be 140. Therefore, the syntheses of both structures (140 and 149) were examined.37 The common intermediate ((+)-126) was oxidized to the aldehyde, which was reacted with vinylmagnesium bromide to provide allylic alcohols (143 and 144) in a ratio of ca. 1:1 (Scheme 34). After separation and stereochemical determination of the two diastereomers, β-isomer (143) was subjected to VO(acac)2-catalyzed oxidation and subsequent protection as the methoxymethyl (MOM) ether, providing epoxides (145 and 146) in a ratio of ca. 1:1.6. The stereochemistry of the major isomer (146) was unambiguously determined to be anti alkoxy–epoxide as depicted. The minor syn-isomer (145) was treated with lithium trimethylsilylacetylide, resulting in epoxy-ring opening to afford acetylene-diol (147). Conversion of 147 into γ-lactone (148) was achieved by intramolecular Wacker-type oxidation. Deprotection of the MOM group in the resultant 148 followed by acetylation provided (+)-1893B (140), which was identical in all respects to those of natural 1893B. On the other hand, the major anti-isomer (146) was transformed into 149, the originally reported structure for 1893B, by the analogous reaction sequence used for 145. The spectroscopic data of 149 did not coincide with those of natural 1893B. Consequently, our total synthesis of 140 verified the stereochemistry of natural (+)-1893B.
5. CONCLUSIONS
This review summarizes the utilization of metathesis for synthesizing nitrogen- and oxygen-bridged seven- to ten-membered carbocycles. The successful formation of medium-sized rings by RCM requires an installation of a conformationally properly disposed diene unit. In the case of the formation of nitrogen-bridged cycloalkenes, the N-alkoxycarbonyl, N-acyl or N-sulfonyl group was found to play an important role in the RCM reaction. The enyne RCM as well as the olefin RCM were also developed to serve the construction of medium-sized bridged heterocycles and were applied to natural products synthesis. Furthermore, the sequential metathesis reaction realized the expeditious access to the oxygen-bridged cyclooctadiene skeleton. Depending upon the targeted structures, a variety of valuable protocols have been devised. There is no doubt that the utility of metathesis will contribute remarkably to the development of organic synthesis in future.
References
1. For recent reviews on the synthetic application of metathesis, see: (a) R. H. Grubbs, A. G. Wenzel, and A. K. Chatterjee, ‘Comprehensive Organometallic Chemistry III,’ Vol. 11, ed. by D. M. Mingos and R. H. Crabtree, Elsevier, Amsterdam, 2007, pp. 179-205; CrossRef (b) J. Mulzer, E. Ohler, and T. Gaich, ‘Comprehensive Organometallic Chemistry III,’ Vol. 11, ed. by D. M. Mingos and R. H. Crabtree, Elsevier, Amsterdam, 2007, pp. 207-269; CrossRef (c) M. Mori and T. Kitamura, ‘Comprehensive Organometallic Chemistry III,’ Vol. 11, ed. by D. M. Mingos and R. H. Crabtree, Elsevier, Amsterdam, 2007, pp. 271-310; CrossRef (d) K. C. Nicolaou, P. G. Bulger, and D. Sarlah, Angew. Chem. Int. Ed., 2005, 44, 4490. CrossRef
2. R. R. Schrock, J. S. Murdzek, G. C. Bazan, J. Robbins, M. DiMare, and M. O’Regan, J. Am. Chem. Soc., 1990, 112, 3875. CrossRef
3. (a) P. Schwab, M. B. France, J. W. Ziller, and R. H. Grubbs, Angew. Chem., Int. Ed. Engl., 1995, 34, 2039; CrossRef (b) P. Schwab, R. H. Grubbs, and J. W. Ziller, J. Am. Chem. Soc., 1996, 118, 100. CrossRef
4. M. Scholl, S. Ding, C. W. Lee, and R. H. Grubbs, Org. Lett., 1999, 1, 953. CrossRef
5. S. B. Garber, J. S. Kingsbury, B. L. Gray, and A. H. Hoveyda, J. Am. Chem. Soc., 2000, 122, 8168. CrossRef
6. G. Illuminati and L. Mandolini, Acc. Chem. Res., 1981, 14, 95. CrossRef
7. G. C. Fu and R. H. Grubbs, J. Am. Chem. Soc., 1992, 114, 5426. CrossRef
8. For reviews on the synthesis of heterocycles by metathesis, see: (a) S. K. Chattopadhyay, S. Karmakar, T. Biswas, K. C. Majumdar, H. Rahaman, and B. Roy, Tetrahedron, 2007, 63, 3919; CrossRef (b) A. Deiters and S. F. Martin, Chem. Rev., 2004, 104, 2199. For a review on the synthesis of five- and six-membered heterocycles by metathesis, see; CrossRef (c) K. C. Majumdar, S. Muhuri, R. Ul Islam, and B. Chattopadhyay, Heterocycles, 2009, 78, 1109. CrossRef
9. (a) A. G. King and J. Meinwald, Chem. Rev., 1996, 96, 1105; CrossRef (b) M. Lounasmaa and T. Tamminen, The Alkaloids, 1993, 44, 1.
10. (a) C. E. Neipp and S. F. Martin, Tetrahedron Lett., 2002, 43, 1779; CrossRef (b) C. E. Neipp and S. F. Martin, J. Org. Chem., 2003, 68, 8867. CrossRef
11. For a review on A1,3-strain, see: R. W. Hoffmann, Chem. Rev., 1989, 89, 1841. CrossRef
12. N. Yu. Kuznetsov, V. N. Khrustalev, I. A. Godovikov, and Y. N. Bubnov, Eur. J. Org. Chem., 2006, 113. CrossRef
13. B. Tursch, J. C. Braekman, D. Daloze, C. Hootele, D. Losman, R. Karlsson, and J. M. Pasteels, Tetrahedron Lett., 1973, 14, 201. CrossRef
14. T. Itoh, N. Yamazaki, and C. Kibayashi, Org. Lett., 2002, 4, 2469. CrossRef
15. N. Yamazaki, T. Ito, and C. Kibayashi, Tetrahedron Lett., 1999, 40, 739. CrossRef
16. The catalyst 2 is poisoned by free bases; however, it is tolerant to hydrochloride salt of amine: G. C. Fu, S. T. Nguyen, and R. H. Grubbs, J. Am. Chem. Soc., 1993, 115, 9856. CrossRef
17. (a) D. Tepfer, A. Goldmann, N. Pamboukdjian, M. Maille, A. Lepingle, D. Chevalier, J. Dénarié, and C. Rosenberg, J. Bacteriol., 1988, 170, 1153; (b) B. Dräger, Nat. Prod. Rep., 2004, 21, 211. CrossRef
18. K. P. Kaliappan, P. Das, S. T. Chavan, and S. G. Sabharwal, J. Org. Chem., 2009, 74, 6266. CrossRef
19. (a) J. B. Brenneman and S. F. Martin, Org. Lett., 2004, 6, 1329; CrossRef (b) J. B. Brenneman, R. Machauer, and S. F. Martin, Tetrahedron, 2004, 60, 7301. CrossRef
20. J. P. Devlin, O. E. Edwards, P. R. Gorham, N. R. Hunter, R. K. Pike, and B. Stavric, Can. J. Chem., 1977, 55, 1367. CrossRef
21. M. Mori, Adv. Synth. Catal., 2007, 349, 121; see also ref 1c. CrossRef
22. (a) M. Mori, T. Tomita, Y. Kita, and T. Kitamura, Tetrahedron Lett., 2004, 45, 4397; CrossRef (b) T. Tomita, Y. Kita, T. Kitamura, Y. Sato, and M. Mori, Tetrahedron, 2006, 62, 10518. CrossRef
23. M. Mori, N. Sakakibara, and A. Kinoshita, J. Org. Chem., 1998, 63, 6082. CrossRef
24. P. Somfai and J. Åhman, Tetrahedron Lett., 1992, 33, 3791. CrossRef
25. I. R. C. Bick, J. W. Gillard, and H. Leow, Aust. J. Chem., 1979, 32, 2537. CrossRef
26. V. K. Aggarwal, C. J. Astle, and M. Rogers-Evans, Org. Lett., 2004, 6, 1469. CrossRef
27. F. López and J. L. Mascareñas, Chem. Eur. J., 2007, 13, 2172. CrossRef
28. I. Hanna and V. Michaut, Org. Lett., 2000, 2, 1141. CrossRef
29. I. Hanna, Tetrahedron Lett., 1999, 40, 2521. CrossRef
30. P. de Armas, F. García-Tellado, and J. J. Marrero-Tellado, Eur. J. Org. Chem., 2001, 4423. CrossRef
31. P. de Armas, C. G. Francisco, and E. Suárez, J. Am. Chem. Soc., 1993, 115, 8865. CrossRef
32. D. Lo Re, F. Franco, F. Sánchez-Cantalejo, and J. A. Tamayo, Eur. J. Org. Chem., 2009, 1984. CrossRef
33. P. Cai, A. T. McPhail, E. Krainer, B. Katz, C. Pearce, C. Boros, B. Caceres, D. Smith, and D. R. Houck, Tetrahedron Lett., 1999, 40, 1479. CrossRef
34. K. Takao, G. Watanabe, H. Yasui, and K. Tadano, Org. Lett., 2002, 4, 2941. CrossRef
35. K. Takao, H. Yasui, S. Yamamoto, D. Sasaki, S. Kawasaki, G. Watanabe, and K. Tadano, J. Org. Chem., 2004, 69, 8789. CrossRef
36. G. Chen, Y. Lin, L. Wen, L. L. P. Vrijmoed, and E. B. G. Jones, Tetrahedron, 2003, 59, 4907. CrossRef
37. (a) H. Yasui, K. Hirai, S. Yamamoto, K. Takao, and K. Tadano, Heterocycles, 2006, 67, 123; CrossRef (b) H. Yasui, K. Hirai, S. Yamamoto, K. Takao, and K. Tadano, J. Antibiot., 2006, 59, 456. CrossRef