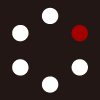
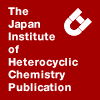
HETEROCYCLES
An International Journal for Reviews and Communications in Heterocyclic ChemistryWeb Edition ISSN: 1881-0942
Published online by The Japan Institute of Heterocyclic Chemistry
e-Journal
Full Text HTML
Received, 22nd June, 2010, Accepted, 15th July, 2010, Published online, 16th July, 2010.
DOI: 10.3987/COM-10-S(E)65
■ Screening the Structural Space of Bicyclo-DNA: Synthesis and Properties of Bicyclo-DNA Functionalized at C(6’)
Samuel Luisier and Christian J. Leumann*
Department of Chemistry and Biochemistry, University of Bern, Freiestrasse, CH-3012 Bern, Switzerland
Abstract
The synthesis of a novel bicyclo-thymidine nucleoside bearing an ester functionality at C(6’) (bcα-alk-nucleosides) is reported. This nucleoside was incorporated into oligodeoxynucleotides via solid phase phosphoramidite chemistry, and the ester moiety was post-synthetically converted to an amide or a carboxy group, or was left unchanged. Thermal melting data (Tm) with complementary DNA and RNA were collected and compared to natural DNA and to bc- and bcox-DNA. It was found that single incorporations of bcα-alk-nucleosides in DNA duplexes were destabilizing by 0.5 to 2.5 °C/mod, whereas two consecutive bcα-alk-residues were less destabilizing, and in some cases even stabilizing by 0.5 °C/mod. In duplexes with complementary RNA, isolated bcα-alk-residues destabilized the duplex by -1.0 to -4.0 °C/mod, depending on the chemical nature of the substituent, whereas two consecutive modifications were only destabilizing by 0.3-1.0 °C/mod. The pairing selectivity was similar to that of unmodified or bc-DNA.INTRODUCTION
The concept of conformational restriction has successfully been applied to nucleic acids and resulted in several nucleic acid analogues, such as locked-nucleic acids (LNA),1,2 hexitol nucleic acids (HNA),3 and tricyclo-DNA (tc-DNA, Figure 1).4,5 These analogues typically exhibit increased affinity to RNA and feature higher nuclease resistance. Due to their properties, such modified nucleic acids are considered as next generation therapeutic drugs.6,7 While the challenges linked to target affinity and nuclease resistance have largely been met in the past, several problems, the most prominent ones being cellular uptake and distribution, largely remained unsolved.
Bicyclo-DNA (bc-DNA),8 was developed as a first generation conformationally constrained oligonucleotide to enhance duplex stability with natural nucleic acids. It turned out, however, that bc-DNA base-pairs to natural nucleic acids only with about equal stability as DNA itself.9 Extensive structural and synthetic investigations suggested that the lack of increased affinity may be due to the structural misalignment of torsion angle γ (C(4’)-C(5’)) with respect to that occurring in duplexes of the A- and B-conformational type.10,11 One of the unique structural features of bc-DNA is the ethylene bridge between the centers C(3’) and C(5’) of the ribose unit which lends itself for further chemical substitution. We reasoned that additional substituents at position C(6’) could be interesting e.g. for controlling the conformation of the carbocyclic ring, eventually correcting the misaligned torsion angle γ. In addition, incorporation of a post-synthetically transformable group could be used either to attach reporter groups or to improve the lipophilic character of bc-DNA, thus improving its cellular uptake properties. Earlier work on C(6’)-oxime modified bc-DNA (bcox-DNA) was encouraging in this context.12
One candidate of interest to be synthesized and tested is bcα-alk-DNA (Figure 1). Here we report on the synthesis of the bcα-alk-T nucleoside carrying a C2-carboxyl substituent in α-position at C(6’), on its conformational preference as explored by molecular modeling, on its incorporation into oligonucleotides and on the base-pairing properties with DNA and RNA.
RESULTS AND DISCUSSION
Modeling of nucleosides and oligonucleotides. A conformational search of bcα-alk-T 9 using Hyperchem yielded two low energy conformers (Table 1) with virtually identical furanose pucker (C1’-exo) but with different cyclopentane ring conformations. In the lowest energy conformer, both the C(5’) and C(6’) substituents were in a pseudo-equatorial arrangement giving rise to a 6’-endo-conformation of the cyclopentane unit (Figure 2a, left). In the second conformer (Figure 2a, right), which is higher in energy by 2.36 kcal/mol, both the C(5’) and C(6’) substituents were in a pseudo-axial arrangement resulting in a C(6’)-exo arrangement of the carbocyclic ring. The relevant backbone torsion angles γ and δ of the two conformers are summarized in Table 1.
Modeling of a DNA-duplex containing one bcα-alk-T nucleotide in the C(1’)-exo/C(6’)-exo conformation resulted in a structure with only minor distortions from an ideal B-conformation at the site of modification (Figure 2b). The C(6’) substituent is pointing away from the minor and major groove, directly into the solvent. Thus no interference of this substituent with base-pairing is to be expected which makes C(6’) a perfect position for the attachment of additional functional groups.
Synthesis of C(6’)-alkyl bicyclonucleosides. We intended to synthesize nucleoside 9 starting from the already known ketone 1 via cyclopropanation of the corresponding silylenol ether 2 or 3 and subsequent ring opening (Scheme 1). Previous experiments showed that cyclopropanation of silylenol ethers derived from ketone 1 under Simmons-Smith conditions13 yielded cyclopropanes in good yields and excellent selectivity.14 We thus prepared the triethylsilyl (TES)-enol ether by treatment of the anomeric mixture of 1 (α:β 4:1) with LDA followed by TES-Cl. Besides the desired silylenol ether 2, some bis-silylated products could be identified in minor quantities. Preliminary experiments towards cyclopropanation of 2 with ethyl diazoacetate (EDA) and Cu(II) catalysts15 showed that carbene insertion into the tertiary OH-function occurred to a non-negligible extent. To suppress this side reaction enol ether 2 was silylated to bis-silylether 3 by treatment with bis(trimethylsilyl) acetamide in pyridine. Compound 3 was then cyclopropanated with EDA, which lead to three isomeric cyclopropanes 4a-c, whose relative configuration at C(5’) and C(6’) could not unambiguously be assigned. Desilylation of the major isomer, 4a, induced cyclopropane ring opening leading to the mixture of isomers of ketone 5. Thus, during desilylation keto-enol tautomerisation takes place leading to epimerization at C(6’). In later experiments we used 4a-c as a mixture of isomers for this reaction, thus reducing the complexity of the mixture from 3 to 2 isomers on the level of ketone 5. Reduction of the isomeric ketones 5 in the presence of excess CeCl3 yielded 6 and 7 in acceptable yields that are separable by column chromatography.
To unambiguously assign the relative configuration at the centers C(6’) and C(7’) we performed 1H-NMR difference-NOE experiments (Figure 3). The consistently large, mutual NOE-effects between the protons at C(4’) and C(5’) indicated their cis-relationship in both isomers 6 and 7. The relatively small NOE between C(5’) and C(6’) in the case of 6 allowed the assignment of a trans relationship for these protons which is corroborated by the relatively large NOE between these protons in isomer 7 indicating a cis relationship. We rationalize the stereoselectivity of the reduction step in 5 with the bicyclic nature of the carbon scaffold which favors hydride transfer to occur on the convex side of the system.
The synthesis of nucleoside 9 and its building block for DNA synthesis 11 continued by treatment of 6 with 2,6-lutidine and trimethylslilyl triflate (TMS-OTf) to produce the glycal 8 in quantitative yields (Scheme 2). We envisaged to perform a β-selective nucleosidation via a two step procedure that had proven successful for tricyclo-nucleoside synthesis before.16 Thus we converted 8 to the corresponding 2’-iodinated nucleoside upon treatment with in situ silylated thymine and N-iodosuccinimide (NIS). The intermediate iodo-nucleoside was not isolated but directly reduced with Bu3SnH. Desilylation of the crude product of this reaction finally yielded nucleoside 9 with only minor amounts <10% of the undesired α-anomer. The β-configuration of the anomeric center in 9 was confirmed by a strong NOE between the C(1’)- and C(4’)-protons in the 1H-NMR NOE difference spectrum (Scheme 2). Likewise, the relative configurations of C(5’) and C(6’) could be confirmed again, as in the case of 6. The relatively large coupling constant of 9.8 Hz together with the relatively low NOE between HC(5’) and HC(6’) speaks for a trans-diaxial arrangement which is in full agreement with the C(6’)-endo conformation of the carbocyclic ring. Likewise. The coupling pattern of the HC(1’) to the two HC(2’) together with the relatively large NOE between HC(4’) and HC(1’) of 4.9% are very similar to that of the unmodified bicyclothymidine, for which the furanose ring was found to prefer the C(1’)-exo conformation in solution and in the solid state.17 Thus the 1H-NMR data are completely conform with a (C(1’)-exo/C(6’)-endo conformation which was also predicted to be the most stable one by modeling (Table 1). Selective tritylation of the sterically less hindered secondary OH in nucleoside 9 with 4,4’-dimethoxytrityl triflate (DMT-OTf) and subsequent phosphitylation of the tertiary alcohol of nucleoside 10 lead to the phosphoramidite building block 11, ready for oligonucleotide synthesis.
Oligonucleotide synthesis. Two oligonucleotides, containing single or double, consecutive bcα-alk-T nucleotides were synthesized on a 0.5 µmol scale by standard automated phosphoramidite chemistry. For incorporation of the modified nucleotides the standard coupling step was extended to 6 min. No further changes were necessary, and the coupling yields for the modified nucleosides were similar to those of unmodified nucleosides. After synthesis the solid supported crude oligonucleotides were split into 4 portions each, followed by separate deprotection and detachment from the solid support using different conditions. Standard ammonolysis with aqueous ammonia yielded oligonucleotides in which the ester function of the bcα-alk-T residues were converted to the unsubstituted amides. Deprotection with 40% methylamine in an ethanol-water mixture (1:2) yielded N-methylamides. Hydrolysis with 1M KOH in water yielded the free carboxylic acid while treatment with the less nucleophilic benzylamine left the ester function unchanged. All oligonucleotides were purified and desalted by standard HPLC methods and analyzed by ESI-MS. Table 2 gives an overview over the isolated oligonucleotides and their determined masses. All were in agreement with the proposed structures. For comparison we also prepared the two reference oligonucleotides Ref1 and Ref2, carrying unsubstituted bicyclo-T units.
Tm measurements. UV-melting curve analysis was performed at 260 nm with a cooling-heating-cooling cycle at a rate of 0.5 °C/min in standard saline buffer (10mM NaH2PO4, 150 mM NaCl, pH 7.0). All curves within a cycle were superimposable thus ruling out non-equilibrium states. Tm data are summarized in Table 3. Incorporation of single bcα-alk-T nucleotides induce a destabilization of -2.5 to -0.6 °C per modification with complementary DNA and -0.7 to -3.9 °C with complementary RNA depending on the chemical nature of the substituent at C(6’). Increased lipophilicity of this substituent, as for the methylamide or ester function lowers the Tm slightly more than a less hydrophobic primary amide function. The carboxylate not unexpectedly destabilizes significantly due to increased charge repulsion in the duplex. However, incorporation of two consecutive bcα-alk-T units slightly stabilize duplexes with complementary DNA and lead to less destabilization with complementary RNA, compared to the mono-substituted oligonucleotides. A comparison with oligonucleotides Ref 1 and Ref 2 shows that the substituents at C(6’) are slightly depressing the Tms with complementary DNA while they have negligible effects if RNA is the pairing complement. Taking into account that there is no major change in conformation between bc-T and bcα-alk-T we believe that the slight variations in Tm are not of structural origin but may reflect the altered hydration sphere between the phosphodiester functions as a consequence of the chemical nature of the substituent. Nevertheless, it appears that an α-substituent in position C(6’) is generally well tolerated on the bicyclo-DNA skeleton.
To get information on the selectivity of base recognition with bcα-alk-DNA, Tm data of duplexes containing a mismatched base opposite to the modification were recorded (Table 4). All mismatched duplexes were destabilized compared to the matched duplexes. However, the degree of destabilization was lower than that observed for bc-DNA. The order of stability of mismatched base pairs is as follows: bcα-alk-T∙dT < bcα-alk-T∙dC < bcα-alk-T∙dG. Interestingly bc-T∙dT and bcα-alk-T∙dT are particularly destabilizing, in contrast to the natural dT∙dT mismatch. The influence of the nature of the substituent at C(6’) was negligible on mismatch destabilization with the exception of the carboxylate substituent that showed less discriminative power.
CONCLUSIONS
We have synthesized a bicyclic nucleoside having a post-synthetically transformable ester function attached to its carbocyclic core. This bicyclic nucleoside was successfully incorporated into oligonucleotides by standard phosphoramidite chemistry. Depending on the deprotection conditions, the original ester function could be transformed in amides and carboxylates or left unchanged. Single incorporations of this modification in oligodeoxynucleotides lead to a slight drop in duplex stability. However, duplexes containing two consecutive modifications exhibited similar or slightly enhanced stabilities compared with duplexes containing parent bc-DNA units or natural deoxynucleotides. No critical differences in stability caused by the different chemical nature of the substituents could be observed. This novel convertible building block 11 may be of interest in the future for attaching fluorescent reporter groups onto oligonucleotides or for post-synthetic derivatization with chemical entities that enhance cellular uptake.
EXPERIMENTAL
All reactions were performed under Ar in dried glassware. Anhydrous solvents for reactions were obtained by filtration through activated aluminium oxide, or by storage over 4 Å molecular sieves. Column chromatography was performed on silica gel (Fluka) with an average particle size of 40 μm. All solvents for CC were of technical grade and distilled prior to use. Thin-layer chromatography (TLC) was performed on silica gel plates (Macherey-Nagel, 0.25 mm, UV254). Visualization was performed either by UV or by staining in dip solution (10.5 g Cer(IV)-sulfate, 21 g phophormolybdenic acid, 60 mL conc. sulfuric acid, 900 mL H2O) followed by heating with a heat gun. NMR spectra were recorded on a Bruker DRX-400 or a Bruker AC-300 spectrometer at 400 MHz or 300 MHz (1H-NMR) or 100 MHz (13C-NMR) in either CDCl3 or CD3OD. δ are given in ppm relative to residual undeuterated solvent (CHCl3: 7.26 ppm (1H) and 77.0 ppm (13C); CHD2OD: 3.35 ppm (1H) and 49.3 ppm (13C)), J in Hz. 13C-multiplicities were determined from DEPT-spectra and signal assignments are based on 13C/1H-HMBC spectra. Proton signal assignments were based on COSY and HMBC. 1H-NMR difference-NOE spectra were recorded on a Bruker DRX-500 instrument at 500 MHz. High resolution electrospray ionization (ESI) mass spectra (MS, m/z) were recorded on an Applied Biosystems Sciex QSTAR Pulsar instrument.
Molecular modeling
Conformational search of the nucleosides were performed using Hyperchem software, using the Amber 2 force field and a Polak-Riebere gradient. All torsion angles on the bicyclic core were modulated and the bicyclic ring system was doubly defined: first as 8-membered ring, then as two five membered rings. A geometry optimization preceded the conformational search, and the 1000 lowest energy conformations were screened for relevant structures. Oligdeoxyonucleotide duplexes were setup in B-conformation using the standard Hyperchem dataset. Modified nucleosides were constructed from the parent natural nucleosides. Geometry optimization was run using the Amber 2 force field with a Polak-Riebere gradient.
Oligonucleotides synthesis
Oligonucleotide syntheses were performed with solid-phase phosphoramidite methodology on a Polygen DNA-synthesizer (1−μmol-slider). Ethyl thiotetrazole (0.25 M in MeCN) was used as activator in the coupling step. Commercial dA-CPG (50 μmol/mg) solid support was used. After deprotection, the crude oligonucleotide solutions were evaporated and dried on a Savant Speed-Vac SC 110.
HPLC
All oligonucleotides were purified by ion-exchange HPLC using an ÄktaTMbasic 10/100 system (Amersham Pharmacia Biotech) on a DNAPAC PA200 column (4 x250 mm, Dionex). All oligonucleotides were desalted after chromatography using Sep-Pak Classic C18 Cartridges (Waters) and were routinely analyzed by ESI mass spectrometry (see Table 3).
UV-Melting curves
UV-melting curves were carried out on a Varian Cary 100Bio UV/Vis spectrophotometer. Absorbances were monitored at 260 nm and the heating rate was set to 0.5 °C/min. A heating-cooling-heating cycle in the temperature range 15-80 °C was applied. The absorbance melting curves were smoothed and the first derivative curves obtained using the Varian WinUV software was used to determine the Tm. To avoid evaporation of the solution, the samples in the cells were covered with a layer of dimethylpolysiloxane. All measurements were carried out in phosphate buffered saline (150 mM NaCl, 10 mM NaH2PO4, pH 7.0). Measurements were carried out at a total strand concentration of 2 µM.
2-Methoxy-6-triethylsilanyloxy-2,3,4,6a-tetrahydrocyclopenta[b]furan-3a-ol (2)
A solution of ketone 1 (α,β ca. 4:1, 494 mg, 2.87 mmol) in THF (5 mL) was added at -78 °C in 5 min to a solution of BuLi (3.6 mL 1.56 M, hexane, 6 mmol, 2 eq) and diiospropylamine (0.83 mL, 6 mmol, 2 eq) in THF (5 mL), followed by triethylchlorosilane (1.05 mL, 6.4 mmol, 2 eq) and TEA (0.5 mL) in THF (5 mL) within 10 min. After stirring for 2 h at -78 °C and 30 min at 0 °C, NH4Cl (1 g) was added. After filtration over Celite, removal of the solvents followed by column chromatography (hexane/EtOAc 3:1), enol ether 2 (441 mg, 54%) was obtained as a colorless oil; TLC (hexane/EtOAc 3:1) Rf = 0.58; 1H-NMR (400 MHz, CDCl3) δ 5.06 (1H, d, J = 4.16 Hz), 4.66 (1H, s), 4.59 (1H, t, J = 2.44 Hz), 3.39 (3H, s), 3.19 (1H, s), 2.42 (2H, t, J = 1.84 Hz), 2.20 (1H, d, J = 13.32 Hz), 2.01 (1H, dd, J = 13.32, 4.04 Hz), 0.98 (9H, t, J = 8.08 Hz), 0.74-0.67 (6H, m); 13C-NMR (100 MHz, CDCl3) δ 152.28 (s), 105.79 (d), 103.15 (d), 92.37 (d), 85.14 (s), 54.95 (q), 47.29 (t), 37.99 (t), 6.85 (q), 5.04 (t); HRMS (ESI+) m/z 309.1492 (M+Na+, C14H26O4NaSi requires 309.1498).
2-Methoxy-6-triethylsilanyloxy-3a-trimethylsilanyloxy-,3a,4,6a-tetrahydro-2H-cyclopenta[b]furan (3)
BSA (0.56 mL, 2.3 mmol, 1eq) was added at rt to a solution of 2 (655 mg, 2.3 mmol) in pyridine (5 mL). After stirring for 12h at rt, a second portion of BSA (0.56 mL, 2.3 mmol, 1 eq) was added. After stirring for another 5h at rt, the solvents were removed by evaporation, yielding 3 (707 mg, 86%) as a slightly brownish oil; TLC (hexane/EtOAc 9:1) Rf = 0.9; 1H-NMR (CDCl3, 400MHz) δ 5.01 (1H, dd, J = 4.40, 0.88 Hz); 4.73 (1H, s), 4.60 (1H, t, J = 2.44, Hz), 3.37 (3H, s), 2.49 (1H, dt, J =15.61, 2.09 Hz), 2.41 (1H, dd, J = 15.50, 1.45 Hz), 2.29 (1H, dd, J = 13.58, 0.71 Hz); 2.03 (1H, dd, J = 13.52, 4.57 Hz), 0.98 (9H, t, J = 7.77 Hz), 0.70 (6H, q, J = 7.96 Hz); 0.14 (9H, s); 13C-NMR (CDCl3, 100MHz) δ 152.09 (s), 105.77 (d), 102.89 (d), 91.53 (d), 86.29 (s), 54.97 (q), 48.59 (t), 42.53 (t), 6.55 (q), 4.77 (t), 1.76 (q); HRMS (ESI+) m/z 381.1897 (M+Na+, C17H34O4NaSi2 requires 381.1893.
3-Methoxy-1a-triethylsilanyloxy-4a-trimethylsilanyloxyoctahydro-2-oxacyclopropa[a]pentalene-1-carboxylic acid ethyl ester (4)
A solution of ethyl diazoacetate (0.6 mL, 5.9 mmol, 3 eq) in CH2Cl2 (100 mL) was dropped at 90 °C over a period of 9h to a solution of 3 (0.7 g, 1.95 mmol) and Cu(acac)2 (19 mg, 0.08 mmol, 0.04 eq) in CH2Cl2 (50 mL). During the addition the solvent was partly evaporated. Filtration over Celite and evaporation of the solvents followed by column chromatography (hexane/EtOAc 15:1 + 0.1 % TEA) yielded a 1.3 : 1 : 1 mixture of isomers 4a-c (4a, 286 mg, 33%; 4b, 217 mg, 25%; 4c, 192 mg, 22%), all as colorless oils.
Data of 4a: TLC (EtOAc) Rf = 0.88; 1H-NMR (CDCl3, 400MHz) δ 5.12 (1H, dd, J = 5.72, 2.20 Hz), 4.58 (1H, s), 4.23 - 4.12 (2H, m), 3.40 (3H, s), 2.27 (1H, dd, J = 13.96, 5.52 Hz), 2.22 (1H, d, J = 1.96 Hz), 2.17 (1H, dd, J = 13.56, 7.00 Hz), 2.06 (1H, d, J = 9.68 Hz), 1.95 (1H, dd, J = 13.72, 1.44 Hz), 1.88-1.83 (1H, m), 1.27 (3H, t, J = 7.07 Hz), 1.00 - 0.91 (9H, m), 0.72-0.67 (6H, m), 0.12 (9H, s); 13C-NMR (CDCl3, 100MHz) δ 168.69 (s), 106.26 (d), 91.49 (s), 88.28 (d), 60.10 (t), 55.14 (q), 47.75 (t), 34.96 (t), 33.50 (d), 30.70 (d), 14.37 (q), 6.73 (q), 5.11 (t), 2.01 (q); HRMS (ESI+) m/z 467.2260 (M+Na+, C21H40O6NaSi2 requires 467.2261). Data of 4b: TLC (EtOAc) Rf = 0.83; 1H-NMR (CDCl3, 400MHz) δ 5.03 (1H, dd, J = 5.80, 3.80 Hz), 4.16 - 4.11 (3H, m), 3.39 (3H, s), 2.41 (1H, dd, J = 13.40, 5.80 Hz), 2.23 - 2.20 (2H, m), 2.14 - 2.04 (2H, m), 1.89 (1H, d, J = 13.80 Hz), 1.27 (3H, t, J = 11.23 Hz), 0.96 (9H, t, J = 8.04 Hz), 0.66 (6H, q, J = 7.89 Hz), 0.12 (9H, s); 13C-NMR (CDCl3, 100MHz) δ 165.32 (s), 105.50 (d), 88.18 (d), 86.63 (s), 61.62 (t), 55.74 (q), 48.48 (t), 41.86 (t), 31.33 (d), 29.56 (d), 14.63 (q), 7.15 (q), 5.76 (t), 2.23 (q); HRMS (ESI+) m/z 467.3166, (M+Na+, C21H40O6NaSi2 requires 467.2261. Data of 4c: TLC (EtOAc) Rf = 0.71; 1H-NMR (CDCl3, 400MHz) δ 5.17 (1H, dd, J = 5.38, 0.83 Hz), 4.83 (1H, s), 4.12 (2H, dq, J = 7.21, 1.85 Hz), 3.36 (3H, s), 2.42 - 2.35 (2H, m), 2.08 (1H, dd, J =14.27, 0.87 Hz), 1.90 (1H, d, J = 13.3 Hz), 1.84 (1H, dd, J = 14.15, 4.82 Hz), 1.79 (1H, d, J = 3.99 Hz), 1.27 (3H, t, J = 7.11 Hz), 0.98 (9H, t, J = 7.94 Hz), 0.73-0.67 (6H, m), 0.15 (9H, s); 13C-NMR (CDCl3, 100MHz) δ 107.56 (d), 98.19 (d), 60.51 (t), 54.88 (q), 47.19 (t), 43.80 (t), 35.01 (d), 30.75 (d), 14.31 (q), 7.12 (q), 6.74 (t), 1.83 (q); HRMS (ESI+) m/z 467.2274 (M+Na+, C21H40O6NaSi2 requires 467.2261).
(3a-Hydroxy-2-methoxy-6-oxohexahydrocyclopenta[b]furan-5-yl)acetic acid ethyl ester (5a,b)
HF-py (0.01 mL 70% HF, ca 1 mmol, 10 eq) was added at 0 °C to a solution of of 4a (39 mg, 0.09 mmol) in py (0.5 mL). After stirring for 1h at rt the reaction was quenched by the addition of silica gel (ca 0.5 g), followed by filtration over Celite after 15 min. Evaporation of the solvents and column chromatography (EtOAc) yielded an inseparable 2:1 mixture of isomers 5a,b (18.4 mg, 79%) as a colorless oil; TLC (hexane/EtOAc 3:1) Rf = 0.20; 1H-NMR (CDCl3, 400MHz) δ 5.10-5.09 (0.4H, d, J = 3.60 Hz), 5.08 (0.6H, d, J = 3.48 Hz), 4.50 (0.4H, d, J = 1.16 Hz), 4.22 (0.5H, s), 4.09 - 4.05 (2H, m), 3.35 (3H, s), 3.35 - 3.00 (1H, m), 2.70 - 2.52 (3H, m), 2.46 - 2.31 (1H, dd, J = 13.56, 5.96 Hz); 2.18 (0.6H, d, J = 11.08 Hz), 2.14 (0.4H, d, J = 11.08), 2.04 - 1.97 (1H, m), 1.91 - 1.85 (1H, m), 1.28 - 1.23 (3H, m), 13C-NMR (CDCl3, 100MHz) δ 171.56 (s), 107.40 (d), 107.00 (d), 89.58 (d), 88.50 (d), 82.43 (s), 61.05 (t), 60.82 (t), 55.24 (q), 55.19 (q), 46.66 (t), 45.29 (t), 44.02 (d), 43.53 (d) 34.04 (t), 34.01 (t), 14.14 (q); HRMS (ESI+) m/z 281.0993, (M+Na+, C12H18O6Na requires 281.1001.
3a-6-Dihydroxy-2-methoxyhexahydrocyclopenta[b]furan-5-yl)acetic acid ethyl ester 6 and 7
A solution of CeCl3 (638 mg) and 5a,b (133 mg, 0.52 mmol ca 1:1 mixture) in MeOH was stirred 15 min at rt. Then NaBH4 (85 mg) was added at 0 °C and the reaction was quenched after 5 min with sat. NaHCO3 (10 mL). After washing with sat. NaHCO3 (2 x10 mL) and extraction with tBuOMe (3 x25 mL) and EtOAc (30 mL), the combined organic phases was dried over MgSO4, filtered and the solvents evaporated. Column chromatography (hexane/EtOAc 1:4) yielded 6 (35 mg, 0.13 mmol, 26%), and 7 (41 mg, 0.16 mmol, 31%) both as colorless oils. Data of 6: TLC (EtOAc) Rf = 0.64; 1H-NMR (CDCl3, 400MHz) δ 5.26 (1H, d, J = 4.28 Hz), 4.30 (1H, d, J = 4.28 Hz), 4.15 - 4.09 (3H, m), 3.39 (3H, s), 2.79 -2.73 (1H, m), 2.62 (1H, dd, J = 15.76, 7.68 Hz), 2.38 (1H, dd, J = 15.76, 6.96 Hz), 2.16 (1H, d, J = 13.60 Hz), 2.03 (1H, dd, J = 13.44, 7.60 Hz), 1.97 (1H, dd, J = 13.68, 4.40 Hz), 1.77 (1H, t, J = 12.32 Hz), 1.26 (3H, t, J = 7.17); 1H-NMR-difference-NOE (CDCl3, 400MHz) δ 5.25 → 3.40 (9.04%), 1.97 (9.32%); 4.34 → 4.09 (6.62%), 2.27 (9.11%); 2.76 → 5.25 (0.63%), 4.34 (5.22%), 4.10 (6.49%), 2.38 (2.34%), 2.15 (1.29%), 2.02 (2.71%); 2.62 → 5.25 (0.93%), 4.33 (2.62%), 4.10 (4.77%), 2.37 (5.27%), 1.76 (1.64%); 2.37 → 5.25 (0.71%), 4.33 (1.85%), 4.10 (1.74%), 2.62 (7.29%); 2.17 → 5.25 (1.69%), 4.33 (2.62%), 4.10 (1.65%), 1.96 (14.68%); 2.05-1.94 → 5.25 (4.11%), 4.33 (2.27%), 4.10 (1.86%), 2.15 (10.52%), 1.77 (9.70%); 1.77 → 4.33 (1.82%), 4.10 (1.14%), 2.60 (2.52%), 2.38 (2.22%), 2.03 (12.36%), 1.99 (1.59%); 13C-NMR (CDCl3, 100MHz) δ 172.93 (s), 109.69 (d), 94.01 (d), 87.48 (s), 72.71 (d), 60.67 (t), 55.15 (q), 48.31 (t), 41.74 (t), 41.23 (d), 34.08 (t), 14.50 (q); HRMS (ESI+) m/z 283.1152, (M+Na+, C12H20O6Na requires 283.1157). Data of 7: TLC (EtOAc) Rf = 0.45; 1H-NMR (CDCl3, 400MHz) δ 5.12 (1H, d, J = 4.25 Hz), 4.17-4.11 (3H, m), 3.72-3.67 (1H, m), 3.38 (3H, s), 2.65 (1H, dd, J = 15.64, 5.11 Hz), 2.33 (1H, dd, J = 15.66, 8.09 Hz), 2.18 (1H, d, J = 13.93 Hz), 2.09-2.00 (3H, m), 1.57 (1H, t, J = 5.35 Hz), 1.26 (3H, t, J = 5.37 Hz); 1H-NMR-NOE (CDCl3, 400MHz) δ 5.14 → 3.40 (5.31%), 2.04 (3.09%); 3.72 → 4.17 (7.48%), 2.69 (12.11%), 2.33 (3.01%) 1.39 (2.24%); 2.66 → 4.16 (2.58%), 3.71 (1.52%), 2.32 (12.56%), 2.19(1.55%), 2.09 (2.41%), 1.38 (0.90%); 2.32 → 4.15 (1.09%), 3.71 (2.58%), 2.66 (), 2.06 (1.65%), 1.38 (2.02 %); 2.19 → 5.12 (0.67%), 2.06 (5.62%); 2.06 → 5.25 (0.60%), 2.67 (1.58%), 2.19 (1.67%), 1.38 (9.16%); 13C-NMR (CDCl3, 100MHz) δ 173.20 (s), 108.32 (d), 87.49 (d), 84.97 (s), 75.94 (d), 60.91 (t), 54.99 (q), 48.91 (t), 41.38 (t), 39.58 (d), 37.02 (t), 14.51 (q); HRMS (ESI+) m/z 283.1151, (M+Na+, C12H20O6Na requires 283.1157).
(3a,6-Bistrimethylsilanyloxy-4,5,6,6a-tetrahydro-3aH-cyclopenta[b]furan-5-yl)acetic acid ethyl ester (8)
Lutidine (0.1 mL, 0.6 mmol, 5 eq) was added at 0 °C to a solution of 6 (29 mg, 0.11 mmol) in CH2Cl2 (0.4 mL), followed by TMS-OTf (0.1 mL, 0.72 mmol, 4 eq) after 5 min. After 15min at 0 °C and 1h at rt, the mixture was diluted with EtOAc (5 mL), washed with sat. NaHCO3 (2 x10 mL), extracted with EtOAc (3 x10 mL) and evaporated to yield crude 8 (50 mg, >100%) as a brown oil, which was used for the next reaction without further purification; TLC (hexane/EtOAc 7:2) Rf = 0.94; 1H-NMR (CDCl3, 400MHz): 6.34 (1H, d, J = 2.72 Hz), 5.12 (1H, d, J = 2.66 Hz), 4.41 (1H, d, J = 4.90 Hz), 4.34 (1H, dd, J = 4.80, 2.92 Hz), 4.14 (2H, q, J = 14.28 Hz), 2.50 - 2.47 (1H, m), 2.45 (1H, m), 2.30 - 2.25 (1H, m), 2.02 (1H, dd, J = 12.63, 6.26), 1.81 (1H, t, J = 12.74 Hz), 1.26 (3H, t, J = 7.09 Hz), 0.09 (9H, s), 0.07 (9H, s); 13C-NMR (CDCl3, 100 MHz) δ 173.03 (s), 148.26 (d), 108.05 (d), 93.99 (d), 90.65 (s), 75.36 (d), 60.21 (t), 44.61 (t), 38.20 (d), 33.24 (t), 14.27 (q), 1.86 (q), 0.13 (q); HRMS (ESI+) m/z 395.1686, (M+Na+, C17H32O5NaSi2 requires 395.1686.
[3a-6-Dihydroxy-2-(5-methyl-2,4-dioxo-3,4-dihydro-2H-pyrimidin-1-yl)hexahydrocyclopenta[b]furan-5-yl]acetic acid ethyl ester (9)
A suspension of BSA (0.14 mL, 0.48 mmol, 4.2 eq) and thymine (61 mg, 0.48 mmol, 4.2 eq) in CH2Cl2 (0.2 mL) was stirred for 1h at rt. Then a solution of crude 8 (50 mg, ca. 0.11 mmol) in CH2Cl2 (0.2 mL) was added and stirring was continued for 30 min. N-Iodosuccinimide (41 mg, 0.18 mmol, 1.5 eq) was added and the mixture stirred for 30 min at 0 °C and for 3h at rt. The reaction mixture was then diluted with EtOAc (5 mL), washed with sat. Na2CO3 (2 x5 mL) and sat. NaHCO3 (5 mL) and extracted with EtOAc (3 x10 mL) to yield the crude iodonucleoside (54 mg, 0.09 mmol, 79%). This intermediate was dissolved in toluene (0.5 mL) and AIBN (10 mg, 0.06 mmol, 0.5 eq) and Bu3SnH (ca 0.05 mL, 0.16 mmol, 1.5 eq) was added. After 2 h at reflux the solvents were removed yielding the crude deiodinated nucleoside (20.7 mg) that was subsequently redissolved in pyridine (1 mL) and treated with a solution of HF-pyridine (ca 0.01 mL 70% HF, 0.2 mmol, 5 eq) for 4h at rt. The reaction mixture was quenched by the addition of silica gel (ca 0.5 g) filtered over Celite and washed with EtOAc/MeOH 10:1 (5 mL). Evaporation and column chromatography (CH2Cl2/MeOH 10:1) yielded 9 (7 mg, 0.014 mmol, 13% from 8) as a colorless foam; TLC (EtOAc) Rf = 0.27; 1H-NMR (CDCl3, 400MHz): 7.65 (1H, d, J = 1.24 Hz), 6.13 (1H, dd, J = 9.80, 5.28 Hz), 4.13 - 4.04 (2H, m), 3.94 (1H, d, J = 5.96 Hz), 3.64 (1H, dd, J =9.80, 5.96 Hz), 2.67 (1H, d, J = 11.12 Hz), 2.40 (1H, dd, J = 13.72, 5.12 Hz), 2.31 - 2.24 (2H, m), 2.14 (1H, dd, J = 12.84, 5.60 Hz), 1.97 (1H, dd, J = 13.80, 9.80 Hz), 1.86 (3H, d, J = 1.20), 1.32 (1H, t, J = 12.20 Hz), 1.21 (3H, dd, J = 8.80, 7.08 Hz); 1H-NMR difference-NOE (CD3OD, 400MHz) δ 7.64 → 6.13 (3.4%), 2.29 (3.6%), 1.97 (4.6%), 1.86 (7.0%); 6.13 → 7.65 (3.1%), 3.94 (4.9%), 2.40 (5.7%), 1.86 (1.8%) 1.21 (1.8%); 3.94 → 6.13 (5.9%), 3.65 (10.3%), 1.86 (1.3%), 1.21 (1.4%); 3.64 → 3.94 (11.0%), 2.27 (4.6%), 1.31 (3.1%);2.67 → 3.69 (2.5%), 2.26 (35.9%); 2.40 → 6.13 (11.7%), 1.97 (23.4%); 2.26 → 7.64 (2.7%), 3.64 (3.6%), 2.67 (14.7%), 1.96 (2.8%); 2.13 → 1.96 (2.3%), 1.31 (22.6%); 1.96 → 7.64 (7.6%), 6.13 (2.4%), 2.39 (20.0%), 2.27 (5.7%), 2.13 (3.9%), 1.21 (1.4%);1.86 → 2.4 (7.6%); 13C-NMR (MeOD, 100 MHz) δ 136.71 (d), 110.78 (s), 88.04 (d), 84.53 (d), 83.47 (s), 75.44 (d), 60.67 (t), 46.91 (t), 41.52 (t), 40.68 (d), 36.40 (t), 13.52 (q), 11.38 (q); HRMS (ESI+) m/z 377.1323 (M+Na+, C16H22N2O7Na requires 377.1324).
{6-[Bis-(4-methoxyphenyl)phenylmethoxy]-3a-hydroxy-2-(5-methyl-2,4-dioxo-3,4-dihydro-2H-pyrimidin-1-yl)hexahydrocyclopenta[b]furan-5-yl}acetic acid ethyl ester (10)
DMT-OTf (250 mg, 0.53 mmol, 3.8 eq) was added in two portions at rt to a solution of 9 (50 mg, 0.14 mmol) in pyridine (0.3 mL). After 13h at rt, the mixture was diluted with EtOAc (5 mL), washed with NaHCO3 (2 x10 mL) and extracted with EtOAc (3 x15 mL), the combined organic layers were dried over MgSO4, filtered and the solvents. Column chromatography (EtOAc + 1% TEA) yielded 10 (66 mg, 72%) as a yellow foam; TLC (EtOAc) Rf = 0.45; 1H-NMR (CDCl3, 400MHz) δ 8.25 (1H, s), 7.67 (1H, d, J = 1.20 Hz), 7.52 - 7.36 (10H, m), 6.82 (4H, m), 6.20 (1H, dd, J = 9.12, 5.32 Hz), 4.03 (2H, dq, J =14.44, 0.88 Hz), 3.80 (7H, m), 3.62 (1H, d, J = 5.48 Hz), 2.58 (1H, dd, J =13.68, 5.20 Hz), 2.20 - 2.04 (4H, m), 1.96 - 1.89 (2H, m), 1.87 (3H, d, J = 1.20 Hz), 1.54 (1H, dd, J = 15.96, 10.32 Hz), 1.26 (1H, m), 1.20 (1H, t, J = 7.12 Hz); 13C-NMR (CDCl3, 100 MHz) δ 172.94 (s), 163.65 (s), 159.22(s), 159.16 (s), 150.33 (s), 145.60 (s), 136.59 (s), 136.52 (s), 135.45 (d), 131.09 (d), 131.05 (s), 128.96 (d), 128.07 (d), 127.46 (d), 113.50 (s), 113.41 (d), 113.40 (d), 113.28 (s), 111.52 (s), 88.42 (d), 87.77 (s), 84.93 (d), 84.83 (s), 76.87 (d), 60.70 (t), 55.59 (q), 55.57 (q), 48.49 (t), 41.20 (d), 40.99 (t), 35.34 (t), 14.47 (q), 12.78 (q); HRMS (ESI+) m/z 679.2613 (M+Na+, C37H40N2O9Na requires 679.2632.
{6-[Bis-(4-methoxyphenyl)phenylmethoxy]-3a-[2-(cyanoethoxy)diisopropylaminophosphanyloxy)-2-(5-methyl-2,4-dioxo-3,4-dihydro-2H-pyrimidin-1-yl)hexahydrocyclopenta[b]furan-5-yl}acetic acid ethyl ester (11)
To a solution of 10 (63 mg, 0.09 mmol) and diisopropylamine (0.07 mL, 0.4 mmol, 4.5 eq) in MeCN (0.3 mL) was added (iPr2N)P(Cl)OCH2CH2CN (0.06 mL, 0.35 mmol, 4 eq) at rt. After stirring for 90min at rt, the mixture was diluted with EtOAc (5 mL), washed with sat NaHCO3 (2 x5 mL) and extracted with EtOAc (3 x10 mL). The combined organic phases were dried over MgSO4, evaporated and the crude product purified by chromatograpy (hexane/EtOAc 1:1) to give 11 (46 mg, 60%) as a colorless foam; TLC (EtOAc) Rf = 0.83, 0.74; 1H-NMR (CDCl3, 400MHz) δ 7.90 (1H, s), 7.66/7.65 (1H, 2d, J = 1.24/1.20), 7.51 - 7.48 (1H, m), 7.43 - 7.39 (4H, m), 6.84 - 6.80 (4H, m), 6.21 - 6.13 (1H, ), 4.05 - 4.02 (2H, m), 3.79/3.78 (6H, 2s), 3.79 - 3.75 (1H, m), 3.73 - 3.61 (2H, m), 3.59 - 3.53 (3H, m), 3.00 - 2.91 (1H, m), 2.60-2.52 (2H, m), 2.18 - 2.04 (3H, m), 1.93 - 1.81 (3H, m), 1.31 - 1.08 (18H, m); 31P-NMR (CDCl3, 100 MHz) δ 142.25, 141.80; HRMS (ESI+) m/z 879.3692 (M+Na+, C46H57N4O10NaP requires 879.3710).
ACKNOWLEDGEMENTS
Financial support by the Swiss National Science Foundation (grant-No: 200020-115913) is gratefully acknowledged.
References
1. A. A. Koshkin, P. Nielsen, M. Meldgaard, V. K. Rajwanshi, S. K. Singh, and J. Wengel, J. Am. Chem. Soc., 1998, 120, 13252. CrossRef
2. S. Obika, D. Nanbu, Y. Hari, J. I. Andoh, K. I. Morio, T. Doi, and T. Imanishi, Tetrahedron Lett., 1998, 39, 5401. CrossRef
3. C. Hendrix, H. Rosemeyer, I. Verheggen, F. Seela, A. Van Aerschot, and P. Herdewijn, Chem. Eur. J., 1997, 3, 110. CrossRef
4. R. Steffens and C. J. Leumann, J. Am. Chem. Soc., 1997, 119, 11548. CrossRef
5. D. Renneberg and C. J. Leumann, J. Am. Chem. Soc., 2002, 124, 5993. CrossRef
6. C. F. Bennett and E. E. Swayze, Annu. Rev. Pharmacol. Toxicol., 2010, 50, 259. CrossRef
7. K. Tiemann and J. J. Rossi, EMBO Molecular Medicine, 2009, 1, 142.
8. M. Tarköy, M. Bolli, and C. Leumann, Helv. Chim. Acta, 1994, 77, 716. CrossRef
9. M. Bolli, H. U. Trafelet, and C. Leumann, Nucleic Acids Res., 1996, 24, 4660. CrossRef
10. M. Egli, P. Lubini, M. Bolli, M. Dobler, and C. Leumann, J. Am. Chem. Soc., 1993, 115, 5855. CrossRef
11. J. C. Litten and C. Leumann, Helv. Chim. Acta, 1996, 79, 1129. CrossRef
12. S. Luisier and C. J. Leumann, ChemBioChem, 2008, 9, 2244. CrossRef
13. J. M. Conia, Pure Appl. Chem., 1975, 43, 317. CrossRef
14. R. Steffens and C. Leumann, Helv. Chim. Acta, 1997, 80, 2426. CrossRef
15. E. J. Corey and A. G. Myers, Tetrahedron Lett., 1984, 25, 3559. CrossRef
16. S. Luisier, P. Silhar, and C. J. Leumann, Nucleic Acids Symp. Ser., 2008, 581. CrossRef
17. M. Tarköy, M. Bolli, B. Schweizer, and C. Leumann, Helv. Chim. Acta, 1993, 76, 481 CrossRef