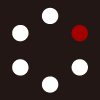
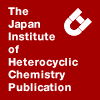
HETEROCYCLES
An International Journal for Reviews and Communications in Heterocyclic ChemistryWeb Edition ISSN: 1881-0942
Published online by The Japan Institute of Heterocyclic Chemistry
e-Journal
Full Text HTML
Received, 16th August, 2010, Accepted, 30th September, 2010, Published online, 1st October, 2010.
DOI: 10.3987/REV-10-678
■ Selenium-Containing Bicyclic β-Lactams
Dinesh R. Garud, Masayuki Ninomiya, and Mamoru Koketsu*
Department of Materials Science and Technology, Faculty of Engineering, Gifu University, 1-1 Yanagido, Gifu, Gifu 501-1193, Japan
Abstract
The first β-lactam ring system was synthesized by H. Staudinger in 1907, but β-lactams as a class of compounds became attractive only after it was established that penicillin contained a β-lactam unit as the structural feature. Over the years, countless numbers of penicillin derivatives and a variety of new β-lactam ring systems have been prepared, tested, and introduced. As a class of β-lactam derivatives, selenium-containing bicyclic β-lactams have received only a limited attention. In this review the advances in the development of synthesis methods for selenium-containing bicyclic β-lactams are presented and discussed.CONTENTS
1. Introduction
2. Synthesis of Selenapenem
3. Synthesis of Selenapenam Using Staudinger Reaction
4. Synthesis of Selenapenams Using Azomethine Ylide Strategy
5. Synthesis of Selenapenams and Selenacephems Using Free-radical Homolytic Substitution Chemistry
6. Synthesis of Selenapenams and Selenacephems Using TSE-Protection Approach
7. Synthesis of Selenium-containing Bicyclic β-Lactams via RCM and RCEYM
8. Synthesis of Isodethiaselenapenam and Isodethiaselenacephem
9. Synthesis of 3-Selena-1-dethiacephams and Selenazepines via Iodocyclization
10. Synthesis of Spirocyclic Selenium-containing β-Lactams
11. Conclusions
12. Acknowledgements
13. References
1. INTRODUCTION
The first β-lactam ring system was synthesized by H. Staudinger in 1907,1 but β-lactams as a class of compounds became attractive only after it was established that penicillin contained a β-lactam unit as the structural feature.2 In early 1900’s the discovery of penicillin began the antibiotic era. The β-lactam (2-azetidinone) skeleton becomes the key structural element of the most widely employed class of antibacterial agents, the β-lactam antibiotics3 and counts for about half of all prescribed antibacterial drugs (cephalosporins: 30%; penicillins: 16%; penams: 5%; macrolides: 18%; quinolones: 19%; others: 12%).4 β-Lactam derivatives with various functional groups are important compounds that attract research interests from both synthetic and pharmaceutical fields.3,5 Interestingly, all known classes of β-lactam antibiotics (with the exception of the monocyclic and spirocyclic β-lactams) share a common structural feature in that the lactam nitrogen is at the ring fusion (Figure 1). The extensive use of common β-lactam antibiotics such as penicillins and cephalosporins in medicine has resulted in an increasing number of resistant strains of bacteria6 and efforts have been made to meet this challenge by exploring new β-lactam chemistry by the skeletal modification of naturally occurring β-lactam antibiotics (Figure 1).7 The nuclear sulphur of penicillins and cephalosporins has been replaced by different atoms (O, N, C) and the chemophysical and microbiological effects of these substitutions have become object of investigation. Also, there is a considerable interest in the modification of the ring system of β-lactams by placing a heteroatom at the 2 or 3 position.
Selenium was discovered in 1817 by J. J. Berzelius8 and the most progress in the area of the synthetic organic chemistry of selenium was accomplished more than 100 years later, in contrast to the chemistry of oxygen- and sulphur-containing organic molecules, which are much better developed. Although the chemistry of selenium-containing compounds is often similar to that of the corresponding sulphur analogues, some significant differences are also known, and because of the toxicity and instability of many selenium compounds, the synthesis of selenium-containing heterocycles is much less developed. In recent years, interest in synthesis of selenium-containing compounds has increased because of their interesting reactivities9 and their potential biological activities. The biological and medicinal properties of selenium and organoselenium compounds are increasingly appreciated, mainly due to their anticancer,10 and for other medicinal applications,11 as well as biologically active substances exhibiting antiviral,12 antibacterial,13 antihypertensive,14 and fungicidal properties.15 It was considered that substitution of the sulphur atom of azetidin-2-ones with selenium preserves its shape and electronic properties but may differ in reactivity and thus potency. Whereas, as a class of β-lactam derivatives, selenium-containing bicyclic β-lactams such as selenapenams and selenacephems have received only a limited attention. In this review the advances in the development of synthesis methods for selenium-containing bicyclic β-lactams are presented and discussed.
2. SYNTHESIS OF SELENAPENEM
Pioneering research contributions in synthesis of selenium-containing bicyclic β-lactam i.e. selenapenem have been made by E. Perrone et al.16 The first synthesis of selenapenem (1) was achieved in two steps from azetidinone 2, and reported together with an evaluation of the antibacterial activity of this novel ring system, which resembled the corresponding penem (Scheme 1).16 As shown in Scheme 1, the treatment of 3,4-cis azetidinone 2 with sodium selenide to afford the (5R)-selenapenem 3, albeit in poor yield (14%). The removal of the PNB-group of 3 was achieved by reduction with iron powder in a buffer medium and the product required for microbiological evaluation was isolated as sodium salt 1 after ion exchange and reverse-phase chromatography. The selenapenem 1 was four-fold less active than the corresponding penem. The selenapenem 1 was prepared in total 8-steps reaction sequence with 0.7% overall yield.
3. SYNTHESIS OF SELENAPENAM USING STAUDINGER REACTION
The Staudinger reaction, involving the [2 + 2] cycloaddition reaction of imines and ketenes, is regarded as one of the most versatile procedures for the stereo-controlled synthesis of substituted 2-azetidinones (β-lactams).17 The next report on the synthesis of selenium-containing bicyclic β-lactams i.e. selenapenam (but lacking a C(2) substituent) was appeared in a Japanese patent based on the use of Staudinger reaction (Scheme 2).18 The synthetic method was started with the diselenide 4, which on treatment with sodium borohydride afforded selenocystine methyl ester followed by in situ reaction with orthoformic acid in 1M HCl/methanol solution resulted in the formation of methyl 1,3-selenazoline-4-carboxylate 5 in 24% yield (Scheme 2). Further, [2+2] cycloaddition of 5 with methoxyacetyl chloride afforded selenapenam 6 in 41% yield, which was converted to free acid by treatment with esterase enzyme to afford selenapenam 7 in 88% yield. Finally, the sodium salt 8 was prepared by the treatment of 7 with 5% NaHCO3/THF solution (Scheme 2).18
4. SYNTHESIS OF SELENAPENAMS USING AZOMETHINE YLIDE STRATEGY
Recently, T. Gallagher et al. described a novel synthetic strategy for the assembly of a variety of bicyclic β-lactam skeletons based on the generation and reactivity of azomethine ylides.19 β-Lactam based N-acyloxazolidinones, such as 9, are critical to this process and thermolysis of 9 in the presence of alkenes or thioketones provides direct access to carbapenams and penams, respectively.19 To date, stable (isolable) 2π dipolarophiles have served as viable traps for 11 (Scheme 3). The equilibrium process shown in Scheme 3 makes it clear that the reactive azomethine ylide 11 is “always present” and, as a consequence, constantly available for the reaction. This unusual mechanistic property has been exploited by capturing a range of less conventional dipolarophiles. By this device, T. Gallagher et al. have extended the scope of β-lactam-containing cycloadducts available. In the similar fashion, the treatment of oxazolidinone 9 with variety of 2π dipolarophiles such as selenoketones, selenothio ester and selenoesters in acetonitrile at reflux resulted in the formation of C(2) substituted selenapenams 12a–12h in moderate yields (Scheme 3).20
5. SYNTHESIS OF SELENAPENAMS AND SELENACEPHEMS USING FREE-RADICAL HOMOLYTIC SUBSTITUTION CHEMISTRY
Free-radical homolytic substitution chemistry is rapidly gaining acceptance as a versatile synthetic method.21 Over the past few years, C. H. Schiesser et al. and his group have demonstrated the effectiveness of this chemistry for the preparation of selenium and tellurium-containing higher membered-ring heterocycles, also have established that the benzyl selenide moiety acts as a “masked” selenolate ion in intramolecular nucleophilic chemistry and this observation has been used to good effect to provide further methods for the construction of selenium-containing rings that include the benzoselenazine-2,4-dione system.22 To that end, selenacephems 17 and 18 were efficiently prepared through homolytic substitution chemistry under standard conditions (Scheme 4).23,24 As shown in Scheme 4, the synthetic route was started with commercially available 4-acetoxy-2-azetidinone 13 which was reacted with sodium benzylselenolate, prepared by treatment of dibenzyl diselenide with sodium borohydride, to give 4-(benzylseleno)-2-azetidinone 14 in quantitative yield. Subsequent treatment of 14 with sodium hydride in DMF at 0 °C followed by an activated electrophile afforded the N-alkylated products 15 or 16 in 30 or 53% yield, respectively (Scheme 4). Finally, the treatment of iodides 15 and 16 with tributyltin hydride in benzene reflux afforded selenacephems 17 and 18 in 84% and 85% yields, respectively (Scheme 4). The compounds 17 and 18 were formed by homolytic attack of the first-formed aryl and vinyl radicals respectively at the selenium moiety, with expulsion of the benzyl leaving group.23,24 This chemistry is analogous to that reported by Beckwith and Boate for the preparation of penicillin analogue (19).25
C. H. Schiesser et al. and his group expanded this chemistry to include the synthesis of the selenapenam 25 through the use of a Kim thiohydroxamate ester 2426 (Scheme 5).24,27 The commercially available, optically pure, (3R,4R)-4-acetoxy-3-[(R)-(tert-butyldimethylsilyloxyethyl]-2-azetidinone (20) was reacted with sodium benzylselenolate, prepared by treatment of dibenzyl diselenide with sodium borohydride, to afford the benzylseleno derivative 21 with complete retention of configuration (de > 98%) (Scheme 5). The treatment of selenide 21 with t-butyl bromoacetate under basic conditions (LHMDS) afforded the ester 22 in 94% yield. Subsequent reaction with ethyl bromoacetate and LHMDS, followed by hydrolysis using barium hydroxide provided the acid 23 as a 7:1 mixture of diastereoisomers. Further conversion of 23 into the thiohydroximate ester 24, which on photolysis afforded the required selenapenam 25 in 51% yield (based on 22) (Scheme 5). In similar fashion, the selenium analogue 26 of the β-lactamase inhibitor, Sulbactam (27) was also prepared (Scheme 5).24,27 Initially the diastereometric ration for the selenapenam 26 was observed to be 1:1, however, upon standing, epimerization to a single diastereoisomer (26) was observed within 72 hours (Scheme 5).24
In an alternative approach by C. H. Schiesser et al. and his group to these classes of compounds, 3,3-dichloro-2,2-dimethylpropionyl chloride 28 was firstly converted into the corresponding amide 29 by reaction with 2-benzylselenoaniline and then into the chloroazetidinone 30 (Scheme 6). The treatment of chloride 30 with one equivalent of sodium iodide in acetone did not provide the iodide 31, rather, the ring-closed selenapenem nucleus, 2,2a-dihydro-2,2-dimethyl-1H-azeto[2,1-b]benzo[1,3]selenazol-1-one 32 was obtained in 46% yields (Scheme 6).23 Presumably the corresponding iodide 31 is formed in situ, but undergoes rapid intramolecular attack by the nucleophilic benzylseleno moiety to provide selenapenem 32 (Scheme 6).
6. SYNTHESIS OF SELENAPENAMS AND SELENACEPHEMS USING TSE-PROTECTION APPROACH
2-(Trimethylsilyl)ethyl (TSE) protection for alcohol28 and thiol29 is well known but the concept of TSE-protection in selenium chemistry was first introduced by T. Murai et al.30 Further, the 2-(trimethylsilyl)ethyl (TSE) protection approach was used for the synthesis of the selenium-containing bicyclic β-lactams.31 A novel selenating reagents, 2-(trimethylsilyl)ethyl p-methylselenobenzoate (33), prepared from potassium p-methylselenobenzoate,32 was used for the incorporation of the TSE-protected seleno moiety onto β-lactam skeleton (Scheme 7). This selenating reagent, 33 has two latent reactive sites, that is, carbonyl carbon and tetraalkylated silicon. The former is susceptible to the nucleophilic attack by amine, thereby producing a 2-(trimethylsilyl)ethaneselenolate30 anion. This anion is expected to react with electrophilic sites, allowing the incorporation of the 2-(trimethylsilyl)ethyl (TSE)-protected seleno moiety on to the β-lactam skeleton. As expected, the reaction of the selenating reagent with 4-acetoxyazetidinone 20 afforded the key intermediate TSE-protected seleno derivative 34, with complete retention of configuration in 92% yield (Scheme 7). Subsequent treatment of 34 with either sodium hydride in THF at 0 °C or lithium hexamethyldisilazide (LHMDS) in THF at -78 °C followed by an activated electrophile afforded the corresponding compounds (35a–h) having electrophilic segments (Scheme 7).
The TSE-selenyl precursors were next applied to TBAF-initiated key annulation to deliver selenacephems, selenapenams, or selenazepane frames (Scheme 8).31 Thus, the treatment of alkyne 35a with excess of TBAF at rt led to formation of the selenium anion, and subsequent attack at the alkynyl carbon afforded the required selenacephem 36 by intramolecular cyclization reaction (Scheme 8). Allenes are a versatile class of organic compounds that feature numerous patterns of reactivity.33 Allenamides are a subclass of allenes that have recently received much attention in the synthetic community.34 The treatment of allenamide 35b with excess of TBAF also resulted in the formation of corresponding selenacephem 37 by the intramolecular cyclization reaction. Further, the reactions of 35c and 35d with TBAF afforded the corresponding selenapenam 38 and selenacepham 39 in high yields, respectively. The treatment of 35e with excess of TBAF resulted in the formation of seven-membered selenium-containing heterocycles i.e. selenazepane 40. Further the TSE-deprotection of 35f with excess of TBAF resulted in the formation of corresponding selenapenam 41 as a single diastereomer. With the aim of exploring other biologically important selenium heterocycles, a benzo-fused selenacephem 42 was obtained by the treatment of 35g with TBAF. The nitro group in the selenacephem 42 is advantageous for the functionalization of selenacephem for structure-activity relationship studies. The selective TSE-deprotection of 35h promoted by the minimized amount of TBAF (1.0 equiv) has yielded a TBS-protected pyridine fused ring system (i.e., selenacephem 43). Further, the TBS-group within selenacephem 43 was cleaved by the action of TFA to afford selenacephem 44 (Scheme 8). This chemoselectivity between silyl groups was first reported. The versatility in the TSE-protection approach was shown by the change in the size of the second ring of the bicyclic structure (5, 6, and 7 membered-rings) and the functional groups for further modifications (Scheme 8).
7. SYNTHESIS OF SELENIUM-CONTAINING BICYCLIC β-LACTAMS VIA RCM AND RCEYM
Ring-closing metathesis (RCM)35 and ring-closing enyne metathesis (RCEYM)36 have recently emerged as a powerful tool for the formation of a variety of ring systems (Figure 2). The generation of β-lactam arrays by RCM and RCEYM has received considerable attention over the past few years. RCM37 and RCEYM38 approaches were first time used for the synthesis of higher ring selenium-containing bicyclic β-lactams.
The difficult task in the synthesis of selenium-containing bicyclic β-lactams by ring-closing metathesis (RCM) was the insertion of the alkene-seleno moieties at the C(4) position of the azetidinones. For this, new selenating reagents, Se-allyl p-methylselenobenzoate (45a) and Se-3-butenyl p-methylselenobenzoate (45b) were prepared. These reagents allowed the insertion of the allyl-seleno or butenyl-seleno moieties onto β-lactam skeleton i.e. compounds 46a–46b under basic conditions (Scheme 9). Subsequent treatment of 46a or 46b with either sodium hydride in THF at 0 ºC or lithium hexamethyldisilazide (LHMDS) in THF at -78 ºC followed by an activated electrophile afforded the corresponding previously unknown key intermediates (47a–47e) in 24–82% yields, respectively (Scheme 9).37
The TSE-protection approach was also used for the insertion of the allyl-seleno or butenyl-seleno moieties onto β-lactam skeleton. The treatment of 34 with either sodium hydride in THF at 0 ºC or lithium hexamethyldisilazide (LHMDS) in THF at -78 ºC followed by an activated electrophile afforded the corresponding compounds 35i–35k (Scheme 9). The deprotection of the TSE-group of 35i–35k by TBAF followed by in situ alkylation of the selenolate anion with alkyl bromide afforded the corresponding substrates (47a–47d and 47f–47h) for the RCM reaction (Scheme 9).37
Among the catalysts screened for the ring-closing metathesis (Figure 2), Grubbs 2nd generation catalyst was found to be most effective for the synthesis of selenium-containing bicyclic β-lactams 48a–48j (Scheme 10).37 This approach is useful for the synthesis of 7-, 8-, or 9-membered selenium-containing bicyclic β-lactams.
For the synthesis of selenium-containing bicyclic β-lactams through RCEYM reactions, the compound 46b having 3-butenyl-seleno moiety at the C(4) position in the azetidinones was treated with sodium hydride in THF at 0 °C and subsequent addition of substituted propargyl bromides afforded the corresponding key intermediates 49a–49d for the RCEYM reaction in 42–74% yields (Scheme 11). Further the RCEYM of 3-butenyl-seleno compounds 49a–49d using 10 mol% of Grubbs 2nd gen. catalyst resulted in the formation of 8-membered selenium-containing bicyclic β-lactams 50b–50d in moderate yields (Scheme 11).38
Next, as shown in Scheme 12, the selective removal of the TSE-group of 35i and subsequent in situ alkylation of the selenolate anion afforded the compounds 51a–51d having propargyl-seleno moieties at their C(4) positions in the azetidinones (Scheme 12).38 Further, the treatment of the intermediates 51b–51d with Grubbs 2nd gen. catalyst (10 mol%) afforded the corresponding 1,3-selenazepine compounds 52b–52d, respectively, in good yields, however, an attempt to bring about the cyclization of 51a in the presence of the Grubbs 2nd gen. catalyst (10 mol%) was failed (Scheme 12).38 This RCEYM approach allows the synthesis of previously inaccessible selenium-containing bicyclic β-lactams.
8. SYNTHESIS OF ISODETHIASELENAPENAM AND ISODETHIASELENACEPHEM
The synthesis of selenium-containing bicyclic β-lactams having selenium atom at the 2 or 3 position i.e. isodethiaselenapenam 56 and isodethiaselenacephem 59 were reported by G. H. Hakimelahi et al. with their biological activity.39 The synthetic approach for isodethiaselenapenam 56 was started with the mesyl derivative 53 (Scheme 13). The treatment of 53 with tBuOK and selenium powder in THF:DMF (9:1) afforded the cis-substituted bicyclic β-lactam 54 as a mixture of two diastereomers in 75% overall yield (Scheme 13). Removal of the tBu group of 54 with TFA followed by decarboxylation by use of NaHCO3 gave phosphonate 55 (55%) upon acidic work up. Treatment of phosphonate 55 with Me3SiBr in DCM produced isodethiaselenapenam 56 in 30% yield (Scheme 13).39
Further, the synthetic approach for isodethiaselenacephem 59 was started with the sodium salt 57 of the cis-epoxysuccinic acid (Scheme 14). After several reaction sequences, the sodium salt 57 was converted into a mesyl derivative 58, which on treatment with tBuOK and selenium powder in THF:DMF (9:1) subsequent treatment of the corresponding isodethiaselenacepham intermediate with DBU in situ at reflux temperature gave racemic bicyclic β-lactam which on hydrogenolysis in the presence of PdCl2 in AcOEt at 50 psi H2 afforded the cis-substituted bicyclic β-lactam isodethiaselenacephem 59 (Scheme 14).39 Next, the biological activity of the isodethiaselenapenam 56 and isodethiaselenacephem 59 was investigated. The isodethiaselenapenam 56 and isodethiaselenacephem 59 were exhibited antimicrobial activity.
9. SYNTHESIS OF 3-SELENA-1-DETHIACEPHAMS AND SELENAZEPINES VIA IODOCYCLIZATION
Iodocyclization of an unsaturated C-C bond with a wide variety of nucleophiles, including N, O, and S nucleophiles, has been extensively studied and has become a powerful tool for the construction of various heterocycles.40 In contrast, only a few examples for the synthesis of selenium heterocycles via electrophilic cyclization have been reported in the literature.41 The retrosynthetic disconnection approach as shown in Figure 3 suggests that a variety of selenium-containing β-lactams having selenium other than at C4-position can be easily prepared from the allene- or alkyne-selenoureas via an iodocyclization reaction.42 Further, the key intermediates alkyne-selenoureas 60 (Scheme 15) or allene-selenoureas 64 (Scheme 17) for the iodocyclization reaction can be readily prepared by the N-alkylation reaction of previously known propargyl-azetidinones43 or allenyl-azetidinones,43 prepared from 20 via indium chemistry, with a wide variety of isoselenocyanates44 under basic conditions in good to excellent yields, respectively (Figure 3).
After screening several reaction conditions it was found that, the iodocyclization reaction of β-alkyne-selenourea 60 using 1.25 equiv. of iodine in dichloromethane at rt resulted in the formation of corresponding 3-selena-1-dethiacepham 61a–61h in excellent yields (Scheme 15).42 The nature of the R2 group on the selenourea had very little effect on the reaction rate or product yields. Aryl-substituted selenoureas (60a–60d) gave slightly higher yield than alkyl-substituted selenoureas (60e–60f). The aryl-substitution at alkynes (i.e. R1 = C6H5) were also well accommodated and afforded the cyclized products 61g and 61h in excellent yields (Scheme 15). The reaction shows high regioselectivity for six-membered ring 3-selena-1-dethiacephams 61. Seven-membered ring products were never detected under these reaction conditions.42
The structure of the 3-selena-1-dethiacephams 61a was also confirmed by chemically. Thus the palladium-catalysed triethylammonium formate reduction of the iodide 61a45 provided only compound 62 but not compound 63 (Scheme 16).42 It was relatively easy to distinguish the isomers 62 and 63 by NMR study. Recently, M. Koketsu et al. and the group have reported that selenium shows strong coupling with trans proton of exocyclic double bond, whereas similar coupling with cis proton was not observed.46 The same observation was found in the compound 62 i.e. selenium shows coupling with trans proton H6b 3J(77Se-1H) = 34.3 Hz exclusively (Scheme 16). Thus, this spectral feature may become an important tool for confirming the structure of selenium-containing compounds. The 3-selena-1-dethiacepham 62 was alternatively obtained by direct CuI mediated intramolecular cyclization of 60a (Scheme 16) in 26% yield.42 Further, the synthesized compounds were screened for their biological activity. The compounds, 3-selena-1-dethiacepham 61e and 3-selena-1-dethiacepham 61f, are capable of attenuating oxidative stress and inhibiting cell growth in hormone-sensitive prostate cancer LNCaP cells.47 Thus, these compounds possess the potential as pharmacological agents for chemoprevention of prostate cancer.
Next, the iodocyclization reaction of unsubstituted β-allene-selenoureas 64 using 1.25 equiv. of iodine resulted in the formation of 3-selena-1-dethiacephems 65a–65e as the major product with traces of the corresponding five-membered product isodethiaselenapenams (Scheme 17).42 Good yields were obtained in all cases, irrespective of the nature of substituent present on the selenourea group (65a–65e). Seven-membered ring products, i.e. selenazepines 66, were not detected. Thus, these reaction conditions show high regioselectivity towards six-membered ring products, 3-selena-1-dethiacephem 65. Next, the iodocyclization reaction of β-allene-selenourea bearing alkyl or aryl groups at the allenyl position was examined with iodine (Scheme 17). It was found that the regiochemistry in the iodocyclization reaction is affected by the nature of the R1 group at the allenyl position. The reaction of alkyl-substituted β-allene-selenoureas 64f–64k with 1.25 equiv. of iodine afforded selenazepines 66f–66k, respectively. The traces of five-membered isodethiaselenapenam were observed as side products.
A plausible mechanism was proposed for the formation of 65 and 66 as shown in Figure 4. The reaction of 64 with iodine gave iodonium A and released an iodine anion at the same time. With the assistance of iodine anion, intramolecular nucleophilic attack of selenium in the selenourea group on the center carbon of allene (when R1 = H) in the favored 6-exo mode affords the corresponding cyclization product 3-selena-1-dethiacephem 65, whereas attack of selenium in selenourea group on the terminal carbon of allene (when R1 = Me, Et, or n-C5H11) in the favored 7-endo mode affords the corresponding cyclization product, selenazepine 66, accompanied by the simultaneous elimination of hydrogen iodide. The iodocyclization reaction is highly regioselective.
Further, the presence of iodine in the 3-selena-1-dethiacepham 61a allowed the further structural elaboration, most notably using palladium-catalyzed coupling reaction (Scheme 18).42 When compound 61a was exposed to Sonogashira coupling conditions48 with phenylacetylene, the corresponding coupling product 67 was isolated in excellent yield (Scheme 18).42 The imine group in the 3-selena-1-dethiacepham 67 is advantageous for further functionalization.
10. SYNTHESIS OF SPIROCYCLIC SELENIUM-CONTAINING β-LACTAMS
The ever-increasing applications of azetidin-2-ones have triggered a renewed interest in the spiro-β-lactams, as they behave as β-turn mimetics49 and β-turn nucleators.50 In this regard, S. S. Bari et al. reported the first synthesis of spirocyclic seleno-β-lactams.51 The synthetic approach was started with 2-benzylselenoethanoic acid (68). Benzyl-selenoazetidin-2-one (70) was synthesized in good yield by treating 2-benzylselenoethanoic acid (68) with the Schiff’s base 69 in the presence of triethylamine as the base and phosphorus oxychloride (POCl3) as the condensing reagent in refluxing benzene (Scheme 19). This cycloaddition reaction resulted in an exclusive formation of trans-β-lactam 70. The β-lactam carbocation equivalent 71 was prepared by chlorination of azetidin-2-one 70, using N-chlorosuccinimide (NCS) with catalytic amount of AIBN in carbon tetrachloride (Scheme 19). The reaction is highly stereoselective. The chlorination at benzylic carbon atom was not observed. Further, the treatment of 71 with propargyl alcohol in silica gel mediated by Lewis acid such as ZnCl2 in refluxing chloroform afforded cis-3-alkoxy-3-benzylseleno-β-lactam 72 in 38% yield (Scheme 19). Finally, the synthesis of spiro seleno-β-lactams by halogen mediated intraselenyl cyclization of 72 was carried out. The treatment of compound 72 with 1 equiv of iodine in dry dichloromethane at room temperature afforded spiro seleno-β- lactam 73a in 85% yield (Scheme 19). Furthermore, when the reaction was carried out with bromine as the halogenating reagent, spiro seleno-β-lactam 73b was obtained but with comparatively lower yields (Scheme 19). The halocyclization reactions resulted in the exclusive formation of five-membered ring spiro seleno-β-lactams via a 5-exo closure process, instead of 6-endo closure (Scheme 19).
11. CONCLUSION
In summary, this review provides advances in the development of synthesis methods for selenium-containing bicyclic β-lactams. However, the synthesis of selenium-containing bicyclic β-lactams will surely have a magnificent future and we have no doubt that many further applications will appear in the future.
12. ACKNOWLEDGEMENTS
This work was supported by a Grant-in-Aid for Science Research from the Ministry of Education, Culture, Sports, Science and Technology of Japan (No. 17550099 and 20590005) to MK. DRG is thankful to the Head Department of Chemistry and Principal of Sir Parashurambhau College, Pune for their support.
References
1. H. Staudinger, Liebigs Ann. Chem., 1907, 51, 356.
2. H. T. Clarke, J. R. Johnson, and R. Robinson, The Chemistry of Penicillin, Princeton University Press: Princeton, 1949.
3. For some reviews on β-lactam antibiotics, see: Chemistry and Biology of β-Lactam Antibiotics, ed. by R. B. Morin and M. Gorman, Academic Press: New York, 1982, Vols. 1-3; R. Southgate, C. Branch, S. Coulton, and E. Hunt, In Recent Progress in the Chemical Synthesis of Antibiotics and Related Microbital Products, ed. by G. Luckacs, Springer-Verlag: Berlin, 1993, Vol. 2, p. 621; R. Southgate, Contemp. Org. Synth., 1994, 1, 417; CrossRef W. Durckheimer, J. Blumbatch, R. Lattrell, and K. H. Scheunemann, Angew. Chem. Int. Ed., 1985, 24, 180; CrossRef D. T. W. Chu, J. J. Plattner, and L. Katz, J. Med. Chem., 1996, 39, 3853. CrossRef
4. F. von Nussbaum, M. Brands, B. Hinzen, S. Weigand, and D. Häbich, Angew. Chem. Int. Ed., 2006, 45, 5072. CrossRef
5. For reviews, see: C. Palomo, J. M. Aizpurua, I. Ganboa, and M. Oiarbide, Eur. J. Org. Chem., 1999, 3223; CrossRef C. Palomo, J. M. Aizpurua, I. Ganboa, and M. Oiarbide, Curr. Med. Chem., 2004, 11, 1837.
6. See, for example: V. P. Sandanayaka and A. S. Prashad, Curr. Med. Chem., 2002, 9, 1145; T. K. Ritter and C.-H. Wong, Angew. Chem. Int. Ed., 2001, 40, 3508; CrossRef N. Díaz, D. Suárez, and K. M. M., Jr. Merz, J. Am. Chem. Soc., 2000, 122, 4197; CrossRef M. I. Page, Chem. Commun., 1998, 1609; CrossRef D. Niccolai, L. Tarsi, and R. Thomas, Chem. Commun., 1997, 2333; CrossRef V. Hook, Chem. Brit., 1997, 33, 34; B. G. Spratt, Science, 1994, 264, 388; CrossRef J. Davies, Science, 1994, 264, 375; CrossRef L. P. Kotra and S. Mobashery, Bull. Inst. Pasteur., 1998, 96, 139; CrossRef D. J. F. Fisher, S. O. Meroueh, and S. Mobashery, Chem. Rev., 2005, 105, 395. CrossRef
7. M. S. Manhas, D. R. Wagle, J. Chiang, and A. K. Bose, Heterocycles, 1988, 27, 1755; CrossRef G. I. Georg, The Organic Chemistry of β-Lactams, VCH, New York, 1992; I. Ojima, Adv. Asymmetric Synth., 1995, 1, 95; I. Ojima and F. Delaloge, Chem. Soc. Rev., 1997, 26, 377; CrossRef C. Palomo, J. M. Aizpurua, I. Ganboa, and M. Oiarbide, Amino Acids, 1999, 16, 321; CrossRef B. Alcaide and P. Almendros, Chem. Soc. Rev., 2001, 30, 226; CrossRef B. Alcaide and P. Almendros, Org. Prep. Proced. Int., 2001, 33, 315; CrossRef C. Palomo, J. M. Aizpurua, I. Ganboa, and M. Oiarbide, Synlett, 2001, 1813; CrossRef B. Alcaide and P. Almendros, Synlett, 2002, 381. CrossRef
8. J. J. Berzelius, Afhandl. Fys. Kemi Mineral., 1818, 6, 42.
9. A. R. Katritzky, C. W. Rees, and E. F. V. Scriven, Comprehensive Heterocyclic Chemistry II. A Review of the Literature 1982–1995, Elsevier Science, Oxford, 1996, vol. 1–11; T. Wirth, Organoselenium Chemistry: Modern Development in Organic Synthesis, Springer, Berlin, 2000; A. Krief, In Comprehensive Organometallic Chemistry, ed. by W. W. Abel, F. G. A. Stone, and G. Wilkinson, Pergamon: Oxford, 1995, Vol. 11, p. 515; Organoselenium Chemistry: A Practical Approach, ed. by T. G. Back, Oxford University Press: U. K., 1999; T. Wirth, Angew. Chem. Int. Ed., 2000, 39, 3740; CrossRef A. R. Katritzky, C. A. Ramsden, F. V. Scriven, and J. K. Taylor, Comprehensive Heterocyclic Chemistry III. A Review of the Literature 1995–2007, Elsevier Science, Oxford, 2008.
10. P. C. Srivastava and R. K. Robins, J. Med. Chem., 1983, 26, 445; CrossRef W. Wu, K. Murakami, M. Koketsu, Y. Yamada, and I. Saiki, Anticancer Res., 1999, 19, 5375; M. Koketsu, H. Ishihara, W. Wu, K. Murakami, and I. Saiki, Eur. J. Pharm. Sci., 1999, 9, 157; CrossRef K. El-Bayoumy and R. Sinha, Mutat. Res., 2004, 551, 181; CrossRef L. Patrick, Altern. Med. Chem., 2004, 9, 239; E. Block, Adv. Exp. Med. Biol., 1996, 401, 155; E. Block, S. Bird, J. F. Tyson, P. C. Uden, X. Zhang, and E. Denoyer, Phosphorus Sulfur Silicon Relat. Elem., 1998, 136, 1.
11. S. W. May, Exp. Opin. Invest. Drugs, 2002, 11, 1261. CrossRef
12. M. J. Parnham and E. Graf, Prog. Drug. Res., 1991, 36, 9.
13. M. Koketsu, H. Ishihara, and M. Hatsu, Res. Commun. Mol. Pathol. Pharmacol., 1998, 101, 179.
14. S. W. May, L. Wang, M. M. Gill-Woznichak, R. F. Browner, A. A. Ogonowski, J. B. Smith, and S. H. Pollock, J. Pharm. Exp. Ther., 1997, 283, 470.
15. T. Göbel, L. Gsell, O. F. Hüter, P. Maienfisch, R. Naef, A. C. O’Sullivan, T. Pitterna, T. Rapold, G. Seifert, M. Sern, H. Szczepanski, and D. J. Wadsworth, Pestic. Sci., 1999, 55, 355; S. I. El-Desoky, S. B. Bondock, H. A. Etman, A. A. Fadda, and M. A. Metwally, Sulfur Lett., 2003, 26, 127; CrossRef C. Lamberth, J. Sulfur Chem., 2004, 25, 39; CrossRef R. M. Kedar, N. N. Vidhale, and M. M. Chincholkar, Orient. J. Chem., 1996, 12, 301; M. A. Metwally, E. Abdel-Latif, F. A. Amer, and G. Kaupp, J. Sulfur Chem., 2004, 25, 63; CrossRef D. D. Erol, M. D. Aytemir, and N. Yulug, Eur. J. Med. Chem., 1996, 31, 731. CrossRef
16. M. Alpegiani, A. Bedeschi, E. Perrone, and G. Franceschi, Tetrahedron Lett., 1986, 27, 3041. CrossRef
17. For review, see: G. I. Georg and V. T. Ravikumar, In The Organic Chemistry of β-Lactams, ed. by G. I. Georg, Verlag Chemie: New York, 1993, p. 257-293.
18. T. Inayama, K. Izawa, K. Soda, H. Tanaka, and H. Nagao, JP 63,83,091; (Chem. Abstr., 1999, 109, 149216 p).
19. S. R. Martel, R. Wisedale, T. Gallagher, L. D. Hall, M. F. Mahon, R. H. Bradbury, and N. J. Hales, J. Am. Chem. Soc., 1997, 119, 2309; CrossRef D. Planchenault, R. Wisedale, T. Gallagher, and N. J. Hales, J. Org. Chem., 1997, 62, 3438; CrossRef M. D. Andrews, G. A. Brown, J. P. H. Charmant, T. M. Peakman, A. Rebello, K. E. Walsh, T. Gallagher, and N. J. Hales, Chem. Commun., 1999, 249; CrossRef T. Gallagher and N. J. Hales, J. Org. Chem., 1997, 36, 1365.
20. G. A. Brown, K. M. Anderson, M. Murray, T. Gallagher, and N. J. Hales, Tetrahedron, 2000, 56, 5579; CrossRef G. A. Brown, K. M. Anderson, J. M. Large, D. Planchenault, D. Urban, N. J. Hales, and T. Gallagher, J. Chem. Soc., Perkin Trans. 1, 2001, 1897. CrossRef
21. C. H. Schiesser and L. M. Wild, Tetrahedron, 1996, 52, 13265; CrossRef J. C. Walton, Acc. Chem. Res., 1998, 31, 99; CrossRef C. H. Schiesser, Chem. Commun., 2006, 4055. CrossRef
22. M. A. Lucas, O. T. K. Nguyen, C. H. Schiesser, and S.-L. Zheng, Tetrahedron, 2000, 56, 3995; CrossRef N. Al-Maharik, L. Engman, J. Malmström, and C. H. Schiesser, J. Org. Chem., 2001, 66, 6286; CrossRef M. C. Fong, M. J. Laws, and C. H. Schiesser, Aust. J. Chem., 1995, 48, 1221. CrossRef
23. M. W. Carland, R. L. Martin, and C. H. Schiesser, Tetrahedron Lett., 2001, 42, 4737. CrossRef
24. M. W. Carland, R. L. Martin and C. H. Schiesser, Org. Biomol. Chem., 2004, 2, 2612. CrossRef
25. A. L. J. Beckwith and D. R. Boate, J. Org. Chem., 1988, 53, 4339. CrossRef
26. S. Kim, C. J. Lim, S. Song, and H. Kang, Synlett, 2001, 688. CrossRef
27. M. W. Carland and C. H. Schiesser, Molecules, 2004, 9, 466. CrossRef
28. S. D. Burke, G. J. Pacofsky, and A. D. Piscopio, Tetrahedron Lett., 1986, 27, 3345; CrossRef L. A. Paquette, D. Backhaus, and R. Braun, J. Am. Chem. Soc., 1996, 118, 11990; CrossRef G. V. Reddy, R. K. Jain, R. D. Locke, and K. L. Matta, Carbohydr. Res., 1996, 280, 261. CrossRef
29. M. Koreeda and W. Yang, J. Am. Chem. Soc., 1994, 116, 10793; CrossRef M. B. Anderson, M. G. Ranasinghe, J. T. Palmer, and P. L. Fuchs, J. Org. Chem., 1988, 53, 3125. CrossRef
30. K. Tani, T. Murai, and S. Kato, J. Am. Chem. Soc., 2002, 124, 5960. CrossRef
31. D. R. Garud, H. Ando, Y. Kawai, H. Ishihara, and M. Koketsu, Org. Lett., 2007, 9, 4455. CrossRef
32. Y. Kawai, H. Ando, H. Ozeki, M. Koketsu, and H. Ishihara, Org. Lett., 2005, 7, 4653; CrossRef M. Nanami, H. Ando, Y. Kawai, M. Koketsu, and H. Ishihara, Tetrahedron Lett., 2007, 48, 1113; CrossRef H. Ishihara and Y. Hirabayashi, Chem. Lett., 1976, 203; CrossRef H. Ishihara, N. Matsunami, and Y. Yamada, Synthesis, 1987, 371. CrossRef
33. H. E. Schuster and G. M. Coppola, Allenes in Organic Synthesis. John Wiley and Sons: New York, 1984.
34. For reviews on allenamides, see: L.-L. Wei, H. Xiong, and R. P. Hsung, Acc. Chem. Res., 2003, 36, 773; CrossRef R. W. Saalfrank and C. J. Lurz, Methoden Der Organischen Chemie (Houben-Weyl), ed. by H. Kropf and E. Schaumann, Georg Thieme Verlag: Stuttgart, 1993, p. 3093.
35. For some recent reviews on olefin metathesis, see: A. Fürstner, Angew. Chem. Int. Ed., 2000, 39, 3012; CrossRef M. E. Maier, Angew. Chem. Int. Ed., 2000, 39, 2073; CrossRef A. Fürstner, Synlett, 1999, 1523; CrossRef D. L. Wright, Curr. Org. Chem., 1999, 3, 211; R. H. Grubbs and S. Chang, Tetrahedron, 1998, 54, 4413; CrossRef S. K. Armstrong, J. Chem. Soc., Perkin Trans. 1, 1998, 371; CrossRef M. Schuster and S. Blechert, Angew. Chem. Int. Ed., 1997, 36, 2036. CrossRef
36. For selected review papers see: a) V. Michelet, P. Y. Toullec, and J.-P. Genêt, Angew. Chem. Int. Ed., 2008, 47, 4268; CrossRef H. Villar, M. Frings, and C. Bolm, Chem. Soc. Rev., 2007, 36, 55; CrossRef S. T. Diver and A. J. Giessert, Chem. Rev., 2004, 104, 1317. CrossRef
37. D. R. Garud, D. D. Garud, and M. Koketsu, Org. Biomol. Chem., 2009, 7, 2591. CrossRef
38. D. B. Bankar and M. Koketsu, Eur. J. Org. Chem., 2010, 2742. CrossRef
39. J. R. Hwu, L.-L. Lai, G. H. Hakimelahi, and H. Davari, Helv. Chim. Acta, 1994, 77, 1037. CrossRef
40. For a two-part review of iodocyclization, see: M. Frederickson and R. Grigg, Org. Prep. Proced. Int., 1997, 2, 33; CrossRef ibid, p. 63; CrossRef F. M. da Silva, J. J. Junior, and M. C. S. de Mattos, Curr. Org. Synth., 2005, 2, 393. CrossRef
41. C. T. Bui and B. L. Flynn, J. Comb. Chem., 2006, 8, 163; CrossRef M. Koketsu, T. Kiyokuni, T. Sakai, H. Ando, and H. Ishihara, Chem. Lett., 2006, 35, 626; CrossRef T. Kesharwani, S. A. Worlikar, and R. C. Larock, J. Org. Chem., 2006, 71, 2307; CrossRef D. Alves, C. Luchese, C. W. Nogueira, and G. Zeni, J. Org. Chem., 2007, 72, 6726; CrossRef D. R. Garud, M. Makimura, H. Ando, H. Ishihara, and M. Koketsu, Tetrahedron Lett., 2007, 48, 7764. CrossRef
42. D. R. Garud and M. Koketsu, Org. Lett., 2008, 10, 3319. CrossRef
43. P. H. Lee, H. Kim, K. Lee, M. Kim, K. Noh, H. Kim, and D. Seomoon, Angew. Chem. Int. Ed., 2005, 44, 1840. CrossRef
44. D. R. Garud, M. Koketsu, and H. Ishihara, Molecules, 2007, 12, 504; CrossRef M. Ninomiya, D. R. Garud, and M. Koketsu, Heterocycles, 2010, 81, 2027. CrossRef
45. S. Cacchi, P. G. Ciattini, E. Morera, and G. Ortar, Tetrahedron Lett., 1986, 27, 5541; CrossRef A.-Y. Peng and Y.-X. Ding, Org. Lett., 2004, 6, 1119. CrossRef
46. M. Koketsu, T. Sakai, T. Kiyokuni, D. R. Garud, H. Ando, and H. Ishihara, Heterocycles, 2006, 68, 1607; CrossRef D. R. Garud, M. Makimura, H. Ando, H. Ishihara, and M. Koketsu, Tetrahedron Lett., 2007, 48, 7764. CrossRef
47. R. Terazawa, D. R. Garud, N. Hamada, Y. Fujita, T. Itoh, Y. Nozawa, K. Nakane, T. Deguchi, M. Koketsu, and M. Ito, Bioorg. Med. Chem., 2010, 18, 7001. CrossRef
48. K. Sonogashira, Y. Tohda, and N. Hagihara, Tetrahedron Lett., 1975, 23, 4467; CrossRef K. Sonogashira, Comprehensive Organic Synthesis, ed. by B. M. Trost and I. Fleming, Pergamon Press: Oxford, 1991, Vol. 3, p. 521.
49. E. Alonso, F. Lopez-Ortiz, C. del-Pozo, E. Peralta, A. Macia, and J. Gonzalez, J. Org. Chem., 2001, 66, 6333. CrossRef
50. H. Bittermann and P. Gmeiner, J. Org. Chem., 2006, 71, 97. CrossRef
51. A. Bhalla, P. Venugopalan, K. K. Bhasin, and S. S. Bari, Tetrahedron, 2007, 63, 3195 CrossRef