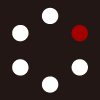
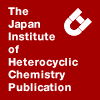
HETEROCYCLES
An International Journal for Reviews and Communications in Heterocyclic ChemistryWeb Edition ISSN: 1881-0942
Published online by The Japan Institute of Heterocyclic Chemistry
e-Journal
Full Text HTML
Received, 7th October, 2010, Accepted, 8th November, 2010, Published online, 17th November, 2010.
DOI: 10.3987/REV-10-683
■ Development of Samarium Diiodide-Promoted Regioselective Carbon-Carbon Bond Cleavage Reaction of γ-Halo- and ε-Halo-α,β-unsaturated Carbonyl Compounds: Application to the Synthesis of Biologically Active Natural Products
Toshio Honda*
Faculty of Pharmaceutical Sciences, Hoshi University, 2-4-41 Ebara, Shinagawa-ku, Tokyo 142-8501, Japan
Abstract
Samarium diiodide (SmI2), a mild and selective one electron transfer reagent, has been utilized in a wide range of synthetic transformations. Among the various reactions developed for SmI2, we focused on its use for fragmentation reactions, and established a regioselective carbon-carbon bond cleavage reaction of γ-halo carbonyl compounds. Utilization of this strategy in the synthesis of various types of biologically active natural products is discussed in this review article.CONTENTS
1. INTRODUCTION
2. DEVELOPMENT OF SAMARIUM DIIODIDE-PROMOTED CARBON-CARBON BOND CLEAVAGE REACTION OF γ-HALO CARBONYL COMPOUNDS
3. SYNTHESIS OF (+)-ELDANOLIDE AND (-)-cis-WHISKY LACTONE
4. NOVEL CHIRAL SYNTHESIS OF NUPHAR PIPERIDINE ALKALOIDS: SYNTHESIS OF (-)-ANHYDRONUPHARAMINE, (-)-NUPHARAMINE, (-)-NUPHENINE, AND (+)-3-EPINUPHARAMINE
5. SYNTHESIS OF A PYRROLIZIDINE ALKALOID, (-)-HELIOTRIDANE
6. SYNTHESIS OF (-)-INVICTOLIDE
7. CHIRAL SYNTHESIS OF NESIC ACID COMPONENTS: SYNTHESIS OF CROBARBATIC ACID AND INTEGERRINECIC ACID LACTONE
8. SYNTHESIS OF A NESIC ACID COMPONENT: SYNTHESIS OF (+)-NEMORENSIC ACID VIA
A CARBON-CARBON BOND CLEAVAGE REACTION OF ε-HALO-α,β-UNSATURATED ESTER
9. CONCLUSION
1. INTRODUCTION
Development of the chemistry of samarium diiodide (SmI2) has been of continuing interest in organic synthesis1 because of its unique and useful properties, such as powerful reducing ability in addition to the chemoselectivity. Although various dehalogenation and deoxygenation reactions of α-hydroxy, α-alkoxy, or α -acyloxycarbonyl compounds are widely investigated to date in the variety of useful transformation utilizing SmI2, little attention has focused on the carbon-carbon bond cleavage reaction.
In 1971, Hey reported that treatment of cis-1,2-divinylcyclobutane derivative with sodium metal afforded the reductive ring-opened product2 (Figure 1). Moreover, Gassman and Creary published that cis-dimethyl bicyclo[2.2.1]heptane-2,3-dicarboxylate was reduced with sodium metal to afford cis-dimethyl 2,2’-cyclopentane-1,3-diyldiacetate3 (Figure 1).
These reports suggested that a treatment of γ,δ-unsaturated carbonyl compound with an appropriate reducing agent might bring about a carbon-carbon bond cleavage reaction between α and β-positions of the carbonyl group, regioselectively.
In fact, a reaction of the γ,δ-unsaturated carbonyl compound (1) with sodium metal in hexamethylphosphoric triamide (HMPA),4 followed by hydrolysis of the crude product by addition of water, gave the hydroxy-acid (2), which was successfully converted to the natural products, (+)-eldanolide and (-)-cis-whisky lactone.5 These results obviously indicated that the expected reductive carbon-carbon bond cleavage reaction took place during the above transformation (Scheme 1), and prompted us to investigate the more effective strategy for this type of fragmentation.5
2. DEVELOPMENT OF SAMARIUM DIIODIDE-PROMOTED CARBON-CARBON BOND CLEAVAGE REACTION OF γ-HALO CARBONYL COMPOUNDS6
In 1982, Magnus and co-workers published a SmI2-promoted reductive cleavage of a 2-chloroethyl carbamate to the corresponding amine and also a reductive fragmentation reaction of a 11α-steroidal xanthate7 (Scheme 2). This strategy was successfully extended to deprotection of a (2,2,2-trichloroethoxy) methyl ether by Evans and Hoveyda.8 Crombie and Rainbow also reported reductive 1,2-eliminations of 3-chloro-tetrahydrofurans and -tetrahydropyrans using SmI2 as a one electron transfer reagent to give the corresponding alkenes9 (Scheme 3).
As mentioned in Scheme 1, we have already established a regioselective carbon-carbon bond cleavage reaction of a γ, δ-unsaturated carbonyl compound by employing sodium metal as a reducing agent. As an extension of this work, we wished to develop an efficient method for the reductive carbon-carbon bond cleavage reaction, regioselectively, at the β-position to the carbonyl function. Since SmI2 is able to interact with both the halogen atom and the carbonyl functional group of γ-halo carbonyl compounds, presumably forming a seven-membered transition state (A), a regioselective carbon-carbon bond cleavage reaction as depicted in Scheme 4 would reasonably be expected, providing the acyclic alkene (B) with retention of the chiral centers as presented in the starting material, during the fragmentation reaction (Scheme 4).
Initially, we applied the SmI2-promoted regioselective carbon-carbon bond cleavage reaction to cyclopentane derivatives,6 readily accessible from (+)- or (-)-carvone as chiral starting materials,10 and the results obtained are summarized in Table 1.
Thus, the γ-bromo ester (3), prepared from the cyclopentane (18) by addition of hydrogen bromide in acetic acid, was treated with 2.5-4.0 equimolar amounts of SmI2 in THF and HMPA (20:1, v/v) at ambient temperature to furnish the desired alkene (16) in 48% yield.
When this reaction was carried out in the absence of HMPA as a co-solvent, reductive dehalogenation only occurred yielding the ester (17) in moderate yield. Reaction of γ-chloro compound (4), derived from 18 by treatment with hydrogen chloride in Et2O, with SmI2 under the same reaction conditions as above provided 16 in 77% yield. Treatment of 4 with tri-n-butyltin hydride and azoisobutyronitrile (AIBN) in refluxing benzene under a radical initiating reaction condition gave none of the fragmentation product, but the dehalogenation compound (18). Reduction of 4 with zinc powder in acetic acid also failed to give the desired compound. Similar reactions for the hydroxy derivatives (5 and 6) induced a lactonization providing the γ-lactone (19). Although the detailed reaction mechanism still remained unclear for the observed fragmentation reaction, these results suggest that this reaction would proceed via a two-electron reduction process involving further reduction of the initially generated radical prior to either proton abstraction of a radical-induced fragmentation.
This fragmentation was also effective to the secondary γ-halo esters (12-15) where the stereoselectivity could not be observed providing a mixture of the alkenic isomers (27) in the same ratio (E:Z = 5:2 or vice versa). Interestingly, reaction of γ-halo ketone with SmI2 under similar reaction conditions as above, usually yielding a cyclobutanol derivative via a ketyl radical,11 afforded the bond cleaved product, probably owing to the biased structure inherent in a trans-bicyclo[3.2.0]heptane ring system.
Thus, we were able to develop a SmI2-promoted regioselective carbon-carbon bond cleavage reaction. Since the newly developed strategy seems to have a generality and wide applicability, we decided to exploit this reaction in the synthesis of biologically active natural products as follows.
3. SYNTHESIS OF (+)-ELDANOLIDE AND (-)-cis-WHISKY LACTONE12
As mentioned above, we have established a novel regioselective fragmentation reaction of γ-halo esters, readily accessible from a monoterpene, carvone, by exploitation of SmI2. As an application of this strategy to natural product synthesis, we became interested in the chiral synthesis of (+)-eldanolide (29),13,14 isolated from the male wing glands of the African sugar cane borer Eldana sacharina (Wlk.) as a sex pheromone, and also in the synthesis of (-)-cis-whisky lactone (30),15,16 one of the important flavors of alcoholic beverages such as whisky, wine, and brandy. It has also been known that cis-whisky lactone has superior scent to the trans isomer17 and a mixture of cis- and trans-whisky lactones exhibits a repellent activity against mosquitos and flies18 (Figure 2).
(+)-Eldanolide (29) and (-)-cis-whisky lactone (30) have a 3,4-disubstituted γ-lactone structure with the same configuration at the 3-position. We sought that this configuration can be transferred from (+)-carvone (31) by exploiting its stereochemical feature.19 Thus, (+)-carvone (31) was converted into the cyclopentane derivative (32) according to the procedure previously developed by us.19 Addition of hydrogen chloride to 32, and subsequent protection of the hydroxy group of the resulting chloride with triethylsilyl chloride afforded the silyl ether (33) in 99% yield from 31. A regioselective bond cleavage reaction of the chloride (33) by treatment with samarium diiodide in THF-HMPA (20:1, v/v) provided the acyclic ester (34) in 85.5% yield, which on exposure to 10% hydrochloric acid brought about deprotection of the silyl group and lactone formation simultaneously to furnish the γ-lactone (35) in 92% yield. The spectroscopic data including its specific optical rotation of 35 were identical with the authentic specimen.19h Since we have already established the conversion of 35 into (-)-cis-whisky lactone (30),19h this synthesis constitutes its formal synthesis (Scheme 5).
(+)-Eldanolide (29) is the diastereoisomer of the intermediate (35) for (-)-cis-whisky lactone with the epimeric dimethylallyl group at the 4-position. Therefore, inversion of the configuration of the hydroxy group in the hydroxy ester (36) was required for completion of the synthesis.
The Mitsunohu reaction of 32 with benzoic acid in the presence of diethyl azodicarboxylate and triphenylphosphine provided the benzoate, which was hydrolyzed with sodium methoxide to give the alcohol (36) in quantitative yield from 32. Addition of hydrogen chloride to 36 and subsequent protection of the hydroxyl group as the triethylsilyl ether afforded the chloride (37) was subjected to the regioselective fragmentation reaction with samarium diiodide providing the ester (38) in 66% yield. Deprotection of the silyl group of 38 with 10% hydrochloric acid afforded (+)-eldanolide (29) in one-step as above in 91% yield. Again the spectroscopic data including the specific optical rotation of the synthetic (+)-eldanolide, [α]D +53.7 (EtOH), were identical to those of the natural material recorded in the literature.13,19h When this fragmentation reaction was applied to the hydroxy ester (36), (+)-eldanolide was also formed in one-step, although the yield (22%) was rather poor (Scheme 6).
Thus, we developed a short synthesis of (+)-eldanolide (29) and (-)-cis-whisky lactone (30) (+)-carvone (31) by utilizing a regioselective fragmentation reaction as a key step.
4. NOVEL CHIRAL SYNTHESIS OF NUPHAR PIPERIDINE ALKALOIDS: SYNTHESIS OF (-)-ANHYDRONUPHARAMINE, (-)-NUPHARAMINE, (-)-NUPHENINE, AND (+)-3-EPINUPHARAMINE20
(-)-Anhydronupharamine (39)21 and (-)-nupharamine (40),22 isolated from the dried rhizome of Nuphar japonicum DC., are naturally occurring sesquiterpenoid alkaloids with three chiral centers on the piperidine ring (Figure 3).
Their structures including absolute stereochemistry have been confirmed by chemical and spectroscopical studies23,24 and also by syntheses.25-27 (-)-Nuphenine (41)28 and (+)-3-epinupharamine (42),29,30 isolated from Nuphar variegatum, are other members of the family of nuphar piperidine alkaloids with the epimeric methyl group at the C-3 position and the absolute configuration of the latter alkaloid (42) was determined to be 2S, 3S, 6S by its chiral synthesis.25 The ring systems and the stereochemical features presented in this group of alkaloids continue to challenge chemists interested in these alkaloids synthesis.31
As mentioned above, we developed a SmI2-promoted carbon-carbon bond cleavage reaction of γ-halo carbonyl compounds, and this strategy was successfully utilized in the synthesis of γ-lactonic natural products.
In continuation of our work on the synthesis of biologically active natural products using a SmI2-promoted fragmentation reaction as a key step, we are interested in developing a novel synthetic route to nuphar piperidine alkaloids. The basic features of our strategy towards these alkaloids involved the formation of an acyclic azide via a regioselective fragmentation reaction of a γ-halo carbonyl compound, followed by an intramolecular aza-Wittig reaction to provide a piperidine ring, as key reactions.
We initially investigated the synthesis of (-)-anhydronupharamine and (-)-nupharamine as follows.
Treatment of the cyclopentane derivative (43) having a γ-halo ester system, derived from (-)-carvone based on our earlier work,19 with SmI2 took place a regioselective fragmentation reaction to give the olefinic ester (44) in 86% yield. Deprotection of the silyl group of 44 with tetra-n-butylammonium fluoride gave the γ-lactone (45), which was further converted into the δ-lactone (48) by three steps involving a reduction of 45 with diisobutylaluminum hydride giving the lactol (46), treatment of the lactol (46) with 2-lithio-2-trimethylsilyl-1,3-dithiane,32 prepared from 2-trimethylsilyl-1,3-dithiane and n-butyllithium, and hydrolysis of the resulting thioacetal (47) with a catalytic amount of p-TsOH in dichloromethane, in 64% yield from 44. Introduction of a furan ring was easily achieved by treatment of 48 with 3-lithiofuran, derived from 3-bromofuran and n-butyllithium, to give the adduct (49), in 92% yield, having all the carbon framework for the natural products. In order to accomplish the synthesis, the replacement of the hydroxy group by an amino function with inversion of the stereochemistry, and a formation of piperidine ring were required. Thus, the alcohol (49) was subjected to Lal’s procedure33 using diethyl azodicarboxylate (DEAD), triphenylphosphine, and diphenylphosphoryl azide (DPPA) to give the azide (50) with the desired stereochemistry, in 88% yield. Finally, construction of a piperidine ring was achieved by employing an aza-Wittig reaction of 50 with triphenylphosphine in refluxing THF to give the imine (51), which, on reduction with sodium borohydride in ethanol provided (-)-anhydronupharamine (39) as a single stereoisomer, in 77% yield from 50. The stereoselectivity exhibited in the formation of 39 can be rationalized by assuming a stable conformation of the imine (51) as depicted in Figure 4, where the bulky substituent (3-methyl-2-butenyl group) occupies quasi-equatorial position and the hydride attack occurs from axial orientation.
The spectroscopic data of 39 including its optical rotation, [α]D -64.9 (c=0.1, CHCl3) {(lit.,27 - 62.5 (c=0.1, CHCl3)}, were identical with those reported in the literature.27 Since anhydronupharamine (39) was already converted into nupharamine (40) by treatment with hydrochloric acid,27 this synthesis constitutes its formal chiral synthesis (Scheme 7).
We next investigated the synthesis of (-)-nuphenine (41) and (+)-3-epinupharamine (42), both having the same configurations at the 2, 3, and 6-positions, by following the above synthetic scheme.
(+)-Eldanolide (29),5,6 easily derived from (+)-carvone by using the essentially same route as for the preparation of 45, was subjected to ring expansion reaction with 2-lithio-2-trimethylsilyl-1,3-dithiane as above via the lactol (53) to furnish the δ-lactone (54), which on introduction of a furan ring gave the alcohol (55) in 89% yield from 53. Introduction of an azido group with the inversion of the stereochemistry employing the Lal's procedure,33 followed by an intramolecular aza-Wittig reaction of 56 afforded the imine (52). Finally the reduction of the imine (52) with sodium borohydride furnished (-)-nuphenine (41), [α]Hg(365 nm) -23.4 (MeOH) {lit.,28 [α]Hg -23 (MeOH)}, as the sole stereoisomer in 73% yield (Scheme 8).
Since the hydration of (-)-nuphenine (41) giving (+)-3-epinupharamine (42) has already been reported by Forrest and Ray,29 this synthesis also constitutes its formal chiral synthesis.
Thus, efficient stereoselective syntheses of nuphar piperidine alkaloids starting from (-)- or (+)-carvone as chiral sources were established by using SmI2-promoted regioselective carbon-carbon bond cleavage reaction of γ-halo ester as the key reaction. This synthetic strategy should be applicable to the chiral synthesis of other naturally occurring nuphar alkaloids.
5. SYNTHESIS OF A PYRROLIZIDINE ALKALOID, (-)-HELIOTRIDANE34
(-)-Heliotridane (57), a saturated degradation product of typical pyrrolizidine alkaloids, such as platynecine and heliotrine, is the simplest necine base with a pyrrolizidine ring, and is the target compound for the application of newly developed synthetic strategies or methods. In deed, this alkaloid has been synthesized in both racemic and optically active forms by a number of groups to date.35 We also planned the chiral synthesis heliotridane (57), in natural enantiomeric form, together with a stereoselective synthesis of pseudo-heliotridane (58) (Figure 5).
We first investigated the synthesis of (-)-pseudo-heliotridane (58) starting from the ester (59), readily obtained from (-)-carvone, via the γ-halo ester utilizing a SmI2-promoted regioselective fragmentation reaction as a key step.12 Removal of triethylsilyl group of 59 in acetic acid-water-THF (3:l:l) afforded the alcohol (60) in quantitative yield, which on treatment with diphenylphosphoryl azide according to the Lal's procedure33 provided the azide (61) with the desired stereochemistry.
Reduction of 61 with magnesium powder in methanol36 for 3 h at 0 °C proceeded smoothly to give the lactam (62). In order to synthesize a bicyclic ring system, the olefinic lactam (62) was subjected to ozonolysis to afford the aldehyde (63) in quantitative yield. Wittig reaction of the aldehyde (63) using methoxymethyltriphenylphosphonium chloride and n-butyllithium provided the eno1 ether (64) as a mixture of E:Z (ca. 1: 1 ratio) stereoisomers, which on treatment with aqueous acetic acid gave the cyclized product (65) as a single stereoisomer, in 24.3% yield from 63. Although the stereochemistry of the hydroxy group of 65 could not be determined at this stage, we used this compound for further conversion into 66, since this stereogenic center would be removed in the next step of this synthesis. Reduction of 65 with triethylsilane in trifluoroacetic acid furnished the known bicyclic lactam (66),35g in 56.9% yield, whose spectroscopic data including specific optical rotation were similar to those reported.35g Since this lactam (66) was already converted into pseudo-heliohidane,37,38 this synthesis constitutes its chiral synthesis (Scheme 9).
Establishing the synthetic procedure for pyrrolizidine ring system from carvone as above, we next attempted the synthesis of (-)-heliotridane in natural enantiomeric form. The acid (67),12 easily prepared from (+)-carvone, was convened into the isopropyl ester (68), which was converted to the alcohol (69) with the inversion of the configuration under the Mitsunobu reaction conditions employing benzoic acid, triphenylphosphine and diethyl azodicarboxylate, followed by alkaline hydrolysis of the resulting benzoate in 84% overall yield from 69.
Addition of hydrogen chloride to the ester (69) and subsequent protection of the hydroxy group with triethylsilyl chloride afforded the chloride (70). SmI2-promoted fragmentation of 70 provided the olefinic ester (71). Removal of triethylsilyl group of 71 in acetic acid-water-THF (3:l:l) gave the alcohol, which on treatment with diphenylphosphoryl aide using the Lal's reaction conditions furnished the azide (72). When the methyl ester was employed in the above reaction, the lactone formation occurred very fast to give the corresponding γ-lactone. Transformation of the azide (72) into (-)-heliohidane was achieved using the similar reaction conditions as described for the synthesis of (-)-pseudo-heliotridane as above. Thus, the azide (72) was reduced with magnesium powder in methanol to give the lactam (73), whose ozonolysis, and subsequent Wittig reaction of the aldehyde (74) afforded the enol ether (75)(E/Z=1:1). Acid treatment of 75, followed by reduction of the resulting aminal (76), a single stereoisomer, with triethylsilane in trifluoroacetic acid yielded the lactam (77), whose spectroscopic data including specific optical rotation supported its structure. Since this lactam (77) was already convened into (-)-heliotridane (57)37,38 this synthesis also constitutes its formal chiral synthesis (Scheme 10).
6. SYNTHESIS OF (-)-INVICTOLIDE39
δ-Lactonic compounds with a wide range of structural feature are often observed in nature and also are of biologically significance. Invictolide (78), isolated from the red imported fire ant queens, Solenopsis invicta (Buren)40a as a queen recognition pheromone, is one of δ-lactonic natural products, and its relative stereochemistry was proposed by Tumlinson and co-workers40b based on spectroscopic analysis and synthesis. Although the absolute stereostructure of natural invictolide (78) was established to have (3R,5R,6S,1’R)-configuration by Mori and Nakazono in 1986,41 both the levorotatory and the racemic forms of invictolide exhibit pheromone activity.40b Whereas its dextrorotatory form, in admixture with its related pheromone, was inactive in surrogate queen field tests.42 Owing to the interesting structural feature having the δ-lactonic moiety with four chiral centers and also to the biological activity, several syntheses for both racemic and optically active invictolide (78) have been appeared in the literature43 (Figure 6).
In searching the structure of 78 for retrosynthetic disconnection, we thought that the most straightforward route to achieve this goal was an exploitation of the ester C, easily available from (-)-carvone,10 as a starting material, since the adjacent methyl and hydroxy groups of natural product were already incorporated in C with correct stereochemistry and the 5R-methyl group could be constructed by chelation-controlled alkylation of the corresponding β-hydroxy ester D (Scheme 11).
Thus, SmI2-promoted regioselective carbon-carbon bond cleavage reaction of 8 in THF-HMPA was carried out to give the ester 22 in 89% yield. Ozonolysis of the ester (22) afforded the aldehyde (79). Oxidative treatment of 79 with m-chloroperbenzoic acid (MCPBA)44 gave the acid, which was further transformed to its isopropyl ester (80) to differentiate the reactivity from the methyl ester presented in the aldehyde (79). Deprotection of the silyl group of 80 with 5% HCl brought about a lactonization to provide the γ-lactone (81). Selective reduction of the lactone (81) with DIBAL, followed by Wittig reaction of the resulting lactol furnished the olefin (82) in reasonable yield. Thus, the all the carbon framework for the side chain of invictolide was constructed stereoselectively. In order to complete the synthesis of 78, a stereoselective installation of the 5R-methyl group of (-)-invictolide was carried out at this stage by using the chelation-controlled alkylation45 providing the desired dimethyl compound (83) after silylation of the resulting secondary alcohol. Based on the consideration of the previous report,43h the stereoselective construction of 3R-methyl group of (-)-invictolide would be achieved without difficulties by a catalytic hydrogenation of the α,β-unsaturated δ-lactone (84). We therefore focused on the conversion of 83 to 84 as follows. Reduction of the ester (83) with DIBAL, followed by a (Z)-selective olefination developed by Still46 afforded the unsaturated ester (84). Finally, removal of the silyl group of 84 by acid treatment took place a lactonization, and subsequent stereoselective hydrogenation of δ-lactone over 10% palladium on carbon furnished invictolide (78) and its 3-epimer in quantitative yield in a ratio of 6:1 (Scheme 12).
Thus, we were able to establish the stereoselective synthesis of a δ-lactonic natural product, invictolide, by using SmI2-promoted carbon-carbon bond cleavage reaction as the key reaction, and were able to prove that this strategy is obviously applicable to the synthesis of other structurally related δ-lactonic natural products, such as Prelog-Djerassi lactone.
7. CHIRAL SYNTHESIS OF NESIC ACID COMPONENTS: SYNTHESIS OF CROBARBATIC ACID AND INTEGERRINECIC ACID LACTONE47
Pyrrolizidine alkaloids,48 a large class of natural products, exhibit interesting biological properties such as hepatotoxic and carcinogenic activities.49 Therefore, much effort has been devoted to developing new methods and strategies for their synthesis. Some of these alkaloids occur in nature as macrocyclic compounds having 11-, 12-, 13-, and 14-membered rings through the diester linkage between the necine base and necic acid (Figure 7).
In our continuing work on the synthesis of pyrrolizidine alkaloids,50 we were interested in the chiral synthesis of (+)-crobarbatic acid and (+)-integerrinecic acid lactone, the necic acid components of the 11- and 12-membered pyrrolizidine alkaloids, crobarbatine and integerrimine, respectively. A challenging feature in the synthesis of the necic acid components is the construction of two stereogenic vicinal methyls and a tertiary hydroxy group. The desired stereochemical control will be able to achieve by the use of an optically active cyclic ketone as a starting material.
The synthesis of crobarbatic acid was established as follows.
The acid (85), prepared from (-)-carvone,19a was converted into the methyl γ-chloro ester (86) or benzyl γ-chloro ester (87), respectively. Addition of methyllithium to 86 gave the tertiary alcohols (88 and 89) in 62 and 20% yield, respectively. When the methylation with methyllithium was applied to the corresponding benzyl esters (87), improved stereoselectivity was observed. After conversion of the adducts (90 and 91) into the methyl esters in two steps, the ratio of the alcohols (88 and 89) increased to ~5:1 in 69% yield. The stereochemistry of the products was determined on the basis of their NMR spectra. The signals for the secondary methyl group (2R-methyl) of compound 88 appeared at δ 0.97 and at δ 10.8 in the 1H and 13C NMR spectra, respectively, whereas the signals for the corresponding methyl group (3S-methyl) of compound 89 were shifted downfield to δ 1.04 in 1H and at δ 16.4 in 13C NMR spectra due to the presence of the cis-hydroxy group. These results supported the view that the major adduct 88 has two methyl groups in a cis configuration. The increased stereoselectivity for the benzyl ester was rationalized by assuming that the relatively bulky benzyl group would prevent the attack of a methyl anion from β-side as depicted in Scheme 13.
For the synthesis of crobarbatic acid, the major tertiary alcohol (88) was converted to its triethylsilyl ether (92). SmI2-promoted fragmentation reaction of 92 afforded the expected olefin (93) in 94% yield. Desilylation of 93 with TBAF resulted in the formation of γ-lactone, which was subjected to ozonolysis and subsequent reductive treatment with NaBH4 to furnish the primary alcohol (94). Difficulties were initially encountered in the conversion of 94 to the olefin (95), however, Grieco’s procedure51 could successfully applied to provide the olefin (95) in good yield. Finally, treatment of the olefin (95) with ruthenium tetroxide under Sharpless’s condition52 yielded crobarbatic acid (96) (Scheme 14).
We next examined the synthesis of integerrinecic acid lactone starting from the tertiary alcohol (89) by adopting the above synthetic route.
SmI2-prompted fragmentation reaction of the triethysilyl ether (97) gave the olefinic ester (98). Removal of the silyl group with TBAF, followed by ozonolysis and reductive treatment with NaBH4 provided the primary alcohol (99). The olefinic γ-lactone (100) was obtained by dehydration of 99 using Grieco’s procedure51 as above. Diisobutylaluminum hydride (DIBAL) reduction of the lactone (100) gave the lactol (101), which on treatment with 2-lithio-2-trimethylsilyl-1,3-dithiane and subsequent acid hydrolysis with p-TsOH furnished the δ-lactone (102). This lactone (102) has previously been converted into (+)-integerrinecic acid lactone (103) by White and co-workers.53 Therefore, the above constitutes a formal synthesis of (+)-integerrinecic acid lactone (Scheme 15). Thus, SmI2-prompted fragmentation reaction of γ-halo carbonyl compound provided a new synthetic pathway to the synthesis of necic acid components.
8. SYNTHESIS OF A NESIC ACID COMPONENT: SYNTHESIS OF (+)-NEMORENSIC ACID VIA A CARBON-CABRON BOND CLEAVAGE REACTION OF ε-HALO-α,β- UNSATURATED ESTER54
As mentioned above, we have developed a new synthetic pathway to the synthesis of necic acid components by application of SmI2-promoted fragmentation reaction of γ-halo carbonyl compounds, where fragmentation occurred between the α and β positions of the carbonyl group regioselectively. Therefore, it would be interesting to investigate the further application of this fragmentation to an ε-halo-α, β-unsaturated ester, where carbon-carbon bond cleavage would be expected to take place between the γ and δ positions of the carbonyl group, regioselectively.
In this section, we would like to report an enantiospecific synthesis of (+)-nemorensic acid (104),55,56 a necic acid component of a macropyrrolizidine alkaloids nemorensine and retroisosenine, isolated from Senecio nemorensis L.,57 in which the regioselective fragmentation reaction of an ε-halo-α,β-unsaturated ester with samarium iodide plays as a key role.
The structure of nemorensic acid, including its absolute configuration, was confirmed to be 104 based on its chiral synthesis starting from (R)-(+)-β-citronellol,56 and also by X-ray analysis of nemorensine (Figure 8). 56 As depicted for the retrosynthetic route in Scheme 16, the basic feature of our strategy for developing (+)-nemorensic acid involved an intramolecular Michael addition58 of the hydroxy function to the α,β-unsaturated ester (E), which could be derived from the ε-halo-α, β-unsaturated ester (F) by a regioselective fragmentation reaction, to construct a tetrahydrofuran ring. The desired ester (F) would be prepared by Wittig olefination of the ketone (G), easily accessible from the known cyclopentanone (H).19a
The requisite starting material was prepared as follows.
An optically active cyclopentanone derivative (105),19a readily derived from (-)-carvone, was treated with methyllithium to give acetyl compounds (106 and 107), in a ratio of ca. 2:1, in 91% yield (Figure 9). The observed stereoselctivity was assumed that the nucleophilic attack of methyllithium to 105 would proceed via the transition state (TS-1) depending upon the stereochemistry of the carboxylic acid.
The minor product was assumed to be 107 based on an NMR study, in which NOE was observed between two methyl groups at the 1 and 2 positions. After the major compound (106) was converted to the corresponding triethylsilyl ether with triethylsilyl triflate, the ketone (108) was subjected to a Horner-Emmons reaction to give the α,β-unsaturated esters (109), as an inseparable mixture of stereoisomers (E/Z = >95 based on NMR analysis), in 96% yield. Since the desired ester was thus prepared, we applied SmI2-promoted fragmentation to 109, and found that the reaction proceeded cleanly at room temperature and carbon-carbon bond cleavage occurred between the γ and δ positions of the carbonyl group, regioselectively, to provide the olefin-esters (110), as a mixture of stereoisomers (E:Z) 2:1 based on NMR analysis), in 91% yield. Treatment of the acyclic α,β-unsaturated esters (110) with TBAF brought about desilylation and subsequent cyclization to give tetrahydrofuran derivatives (111) as a mixture of cis- and trans-isomers in quantitative yield. Ozonolysis of the mixture, followed by reductive treatment with sodium borohydride gave alcohols (112 and 113), which could be separated by HPLC column chromatography on silica gel to give the 2,5-cis-alcohol (112) and the 2,5-trans-alcohol (113) in yields of 17% and 59%, respectively (Scheme 17).
The stereoselectivity observed in the formation of 111 can be rationalized by assuming that the cyclization proceeds via the sterically favorable transition state TS-3 to predominantly give the 2,5-trans-isomer (111-A), whereas, in transition state TS-4, which leads to the 2,5-cis-isomer (111-B), steric repulsion is present between the methyl groups at the 3 and 6 positions (Figure 10).
A similar cyclization has been previously reported by White and co-workers, who observed a similar stereoselectivity.56 Stereochemical assignment of these alcohols was made based on the analysis of their NMR spectra. In the NMR spectrum of 112, NOE of 1.3% between the methyl group at the 5-position and the 4-β-proton was observed in addition to that between the 4-α-proton and the methylene protons of the acetate unit. On the other hand, the 4-β-proton and 4-α-proton were correlated to the methylene protons of the acetate and methyl group at the 5-position, respectively, in the NMR spectrum of 113, as depicted in Figure 11.
Although the desired tetrahydrofuran derivative having the same stereochemistry as the natural compound was isolated as a minor stereoisomer, we focused our attention on conversion of the hydroxyethyl moiety to the one-carbon-reduced carboxylic acid. To determine the stereochemistry unambiguously, both alcohols (112 and 113) were converted to the olefins (114 and 116) using Grieco’s procedure51via the corresponding 2-nitrophenylselenides, respectively. Ozonolysis of the olefin (112), followed by reductive workup with triphenylphosphine gave the aldehyde (115), which was subjected to further oxidation with sodium chlorite59 and subsequent hydrolysis of the ester group to give the acid (104) in 72% yield from the olefin (115) (Scheme 18). The spectral properties including the specific optical rotation of 104 were identical to those reported in the literature.56 The major isomer (113) was also transformed into the known trans-nemorensic acid (118),60 via the olefin (116) and aldehyde (117), by using the same procedure as described for the preparation of 104 in 80% yield from 117.
Again, we have disclosed a novel stereospecific synthetic route for (+)-nemorensic acid by exploitation of SmI2-promoted regioselective carbon-carbon bond cleavage reaction of an ε-halo-α,β-unsaturated ester. This type of fragmentation with SmI2 is unprecedented, and the strategy developed here should also be applicable to the synthesis of other types of necic acid components of macropyrrolizidine alkaloids.
9. CONCLUSION
Development of the chemistry of SmI2 has been of continuing interest in organic synthesis because of its unique and useful properties, such as powerful reducing ability in addition to the chemoselectivity. As mentioned above, we have developed a novel SmI2-promoted regioselective carbon-carbon bond cleavage reaction, and successfully applied this strategy to the synthesis of various types of biologically active heterocyclic natural products.
Although the target molecules are not heterocyclic compounds, SmI2-promoted fragmentation reaction was employed in the chiral synthesis of a natural antibiotic, (-)-oudemansin A,6 a potent inhibitor of respiration. This strategy was also utilized in the stereoselective synthesis of a steroidal side chain containing a 26-27 cyclopropane ring, which was further transformed to the naturally occurring marine steroid, petrosterol61 (Figure 12). We hope that this methodology is recognized as one of the versatile synthetic tools, and many organic chemists employ this reaction in the synthesis of useful bioactive compounds including natural products.
ACKNOWLEDGEMENTS
This research was supported financially in part by a Grant-in-Aid from the Ministry of Education, Culture, Sports, Science and Technology of Japan. I would also like to thank the dedicated undergraduate, graduate and postdoctoral student, and research associates in my group who made this work possible. They are listed as co-authors and I sincerely regret any inadvertent omissions.
References
1. For recent reviews of SmI2-mediated reaction, see: (a) J. Inanaga, J. Org. Synth. Chem., 1989, 47, 200; CrossRef (b) J. A. Soderquist, Aldrichim. Acta, 1991, 24, 15; (c) D. P. Curran, T. L. Fevig, C. P. Jasperse, and M. J. Totleben, Synlett, 1992, 943; CrossRef (d) G. A. Molander, Chem. Rev., 1992, 92, 29; CrossRef (e) G. A. Molander and C. R. Harris, Chem. Rev., 1996, 96, 307; CrossRef (f) T. Skrydstrup, Angew. Chem., Int. Ed. Engl., 1997, 36, 345; CrossRef (g) G. A. Molander and C. R. Harris, Tetrahedron, 1998, 54, 3321; CrossRef (h) R. Nomura and T. Endo, Chem. Eur. J., 1998, 4, 1605; CrossRef (i) A. Krief and A.-M. Laval, Chem. Rev., 1999, 99, 745; CrossRef (j) P. G. Steel, J. Chem. Soc., Perkin Trans.1, 2001, 2727; CrossRef (k) S. Agarwal and A. Greiner, J. Chem. Soc., Perkin Trans. 1, 2002, 2033; CrossRef (l) H. B. Kagan, Tetrahedron, 2003, 59, 10351; CrossRef (m) M. Berndt, S. Gross, A. Hölemann, and H.-U. Reissig, Synlett, 2004, 422; CrossRef (n) D. J. Edmonds, D. Johnston, and D. J. Procter, Chem. Rev., 2004, 104, 3371; CrossRef (o) D. Y. Jung and Y. H. Kim, Synlett, 2005, 3019; CrossRef (p) K. Gopalaiah and H. B. Kagan, New J. Chem., 2008, 32, 607; CrossRef (q) I. M. Rudkin, L. C. Miller, and D. J. Procter, Organomet. Chem., 2008, 34, 19; CrossRef (r) K. C. Nicolaou, S. P. Ellery, and J. S. Chen, Angew. Chem. Int. Ed., 2009, 48, 7140; CrossRef (s) D. J. Procter, R. A. Flowers, II, and T. Skrydstrup, Organic Synthesis Using Samarium Diiodide: A Practical Guide; Royal Society of Chemistry Publishing: UK, 2010; p. 204; For the key reports of SmI2-HMPA system, see: (t) J. Inanaga, M. Ishikawa, and M. Yamaguchi, Chem. Lett., 1987, 16, 1485; CrossRef (u) E. Hasegawa and D. P. Curran, Tetrahedron Lett., 1993, 34, 1717; CrossRef (v) F. Machrouhi, B. Hamann, J.-L. Namy, and H. B. Kagan, Synlett, 1996, 633; CrossRef (w) M. Shabangi and R. A. Flowers, Jr., Tetrahedron Lett., 1997, 38, 1137; CrossRef (x) M. Shabangi, J. M. Sealy, J. R. Fuchs, and R. A. Flowers, Jr., Tetrahedron Lett., 1998, 39, 4429. CrossRef
2. H. Hey, Angew. Chem., Int. Ed. Engl., 1971, 10, 132. CrossRef
3. P. G. Gassman and X. Creary, J. Chem. Soc., Chem. Comm., 1972, 1214. CrossRef
4. G. M. Whitesides and W. J. Ehmann, J. Org. Chem., 1970, 35, 3565. CrossRef
5. Y. Suzuki, W. Mori, H. Ishizone, K. Naito, and T. Honda, Tetrahedron Lett., 1992, 33, 4931. CrossRef
6. T. Honda, K. Naito, S. Yamane, and Y. Suzuki, J. Chem. Soc., Chem. Comm., 1992, 1218. CrossRef
7. T. P. Ananthanarayan, T. Gallagher, and P. Magnus, J. Chem. Soc., Chem. Comm., 1982, 709. CrossRef
8. D. A. Evans and A. H. Hoveyda, J. Am. Chem. Soc., 1990, 112, 6447. CrossRef
9. L. Crombie and L. J. Rainbow, Tetrahedron Lett., 1988, 29, 6517. CrossRef
10. (a) T. Kametani, Y. Suzuki, C. Ban, and T. Honda, Heterocycles, 1987, 26, 1491; CrossRef (b) T. Kametani, T. Honda, H. Ishizone, K. Kanada, K. Naito, and Y. Suzuki, J. Chem. Soc., Chem. Commun., 1989, 646; CrossRef (c) T. Honda, H. Ishizone, W. Mori, K. Naito, and Y. Suzuki, J. Chem. Soc., Perkin Trans.1, 1991, 3027. CrossRef
11. (a) J. J. Sosnowski, E. B. Danaher, and R. K. Murray, Jr., J. Org. Chem., 1985, 50, 2759; CrossRef (b) G. A. Molander and J. A. McKie, J. Org. Chem., 1991, 32, 1112.
12. T. Honda, S. Yamane, K. Naito, and Y. Suzuki, Heterocycles, 1994, 37, 515. CrossRef
13. (a) G. Kunesch, P. Zagatti, J. Y. Lallemand, A. Debal, and J. P. Vigneron, Tetrahedron Lett., 1981, 22, 5270; CrossRef (b) J. P. Vigneron, R. Menc, M. Larcheveque, A. Debal, G. Kunesch, P. Zagani, and M. Gallois, Tetrahedron Lett., 1982, 23, 5051. CrossRef
14. Synthesis of optically active eldanolide: (a) T. Uematsu, T. Umemura, and K. Mori, Agric. Biol. Chem., 1983, 47, 597; CrossRef (b) Y. Yokoyama and M. Yunokihara, Chem. Lett., 1983, 1245; CrossRef (c) K. Suzuki, T. Ohkuma, and G. Tsuchihashi, Tetrahedron Lett., 1985, 26, 861; CrossRef (d) R. M. Orutuno, R. Merce, and J. Font, Tetrahedron Lett., 1986, 27, 2519; CrossRef (e) T. Ebata, K. Matsumoto, H. Yoshioshi, K. Koseki, H. Kawakami, K. Okano, and H. Matsushita, Heterocycles, 1993, 36, 1017; CrossRef (f) B. K. Smah and N. C. Barua, Tetrahedron, 1993, 49, 2253. CrossRef
15. (a) H. Tsukasa, Koryo, 1988, 158, 95; (b) M. Masuda and K. Nishimura, Chem. Lett., 1981, 1333. CrossRef
16. Synthesis of optically active cis-whisky lactone: (a) C. Gunther and A. Mosandl, Liebigs Ann. Chem., 1986, 2112; CrossRef (b) R. Bloch and L. Gilbert, J. Org. Chem., 1987, 52, 4603; CrossRef (c) D. Hoppe and O. Zschage, Angew. Chem., Int. Ed. Engl., 1989, 28, 69; CrossRef (d) M. Bechmann, H. Hildebrabdt, and E. Winterfeldt, Tetrahedron:Asymmetry, 1990, 1, 335; CrossRef (e) G. V. Shma, S. R. Vepachdu, and S. Chandrasekher, Synth. Commun., 1990, 20, 3403; CrossRef (f) O. Miyata, T. Shinada, N. Kawakami, K. Taji, I. Ninomiya, T. Naito, T. Date, and K. Okamura, Chem. Pharm. Bull., 1992, 40, 2579; CrossRef (g) T. Ebata, K. Matsumoto, H. Yoshikoshi, K. Koseki, H. Kawakami, K. Okano, and H. Matsushita, Heterocycles, 1993, 36, 1017. CrossRef
17. K. Otsuka, Y. Zenibayashi, M. Itoh, and A. Totsuka, Agric. Biol. Chem., 1974, 38, 485. CrossRef
18. Y. Shono and H. Tsukasa, Nippon Kokai Tokkyo Koho, 63-48203.
19. (a) T. Kametani, Y. Suzuki, C. Ban, and T. Honda, Heterocycles, 1987, 26, 1491; CrossRef (b) T. Kametani, Y. Suzuki, C. Ban, K. Kanada, and T. Honda, Heterocycles, 1987, 26, 1789; CrossRef (c) T. Kametani, T. Honda, H. Ishizone, K. Kanada, K. Naito, and Y. Suzuki, J. Chem. Soc., Chem. Commun., 1989, 646; CrossRef (d) T. Honda, H. Ishizone, K. Naito, and Y. Suzuki, Heterocycles, 1990, 31, 1225; CrossRef (e) T. Honda, H. Ishige, M. Tsubuki, K. Naito, and Y. Suzuki, J. Chem. Soc., Perkin Trans. 1, 1991, 954; CrossRef (f) T. Honda, H. Ishige, M. Tsubuki, K. Naito, and Y. Suzuki, Chem. Pharm. Bull., 1991, 39, 1641; CrossRef (g) T. Honda, H. Ishizone, W. Mori, K. Naito, and Y. Suzuki, J. Chem. Soc., Perkin Trans. 1, 1991, 3027; CrossRef (h) Y. Suzuki, W. Mori, H. Ishizone, K. Naito, and T. Honda, Tetrahedron Lett., 1992, 33, 4931; CrossRef (i) T. Honda, H. Isbizone, K. Naito, W. Mori, and Y. Suzuki, Chem. Pharm. Bull., 1992, 40, 2031; CrossRef (j) T. Honda, N. Haze, H. Ishige, K. Masuda, K. Naito, and Y. Suzuki, J. Chem. Soc., Perkin Trans. I , 1993, 539. CrossRef
20. (a) T. Honda, F. Ishikawa, and S. Yamane, J. Chem. Soc., Chem. Commun., 1994, 499; CrossRef (b) T. Honda, F. Ishikawa, and S. Yamane, Heterocycles, 2000, 52, 313. CrossRef
21. Y. Arata, T. Ohashi, M. Yonemitsu, and S. Yasuda, Yakugaku Zasshi, 1967, 87, 1094.
22. (a) Y. Arata and T. Ohashi, Yakugaku Zasshi, 1957, 77, 792; (b) T. Ohashi, Yakugaku Zasshi., 1959, 79, 729 and 734.
23. I. Kawasaki, S. Matsutani, and T. Kaneko, Bull. Chem. Soc. Jpn., 1963, 36, 1474. CrossRef
24. Y. Itatani, S. Yasuda, M. Hanaoka, and Y. Arata, Chem. Pharm. Bull., 1976, 24, 2521. CrossRef
25. S. Aoyagi, Y. Shishido, and C. Kibayashi, Tetrahedron Lett., 1991, 32, 4325. CrossRef
26. I. Shimizu and H. Yamazaki, Chem. Lett., 1990, 777. CrossRef
27. A. Leniewski and J. Szychowski, Coll. Czech. Chem. Commun., 1991, 56, 1309. CrossRef
28. R. Barchet and T. P. Forrest, Tetrahedron Lett., 1965, 4229. CrossRef
29. T. P. Forrest and S. Ray, Can. J. Chem., 1971, 49, 1774. CrossRef
30. M. Sabat, T. Glowiak, J. Szychowski, J. T. Wrobel, and A. Leniewski, Can. J. Chem., 1977, 55, 3111. CrossRef
31. J. T. Wrobel, in "The Alkaloids", ed. by R. H. F. Manske, New York, 1967, Vol. 9, p. 441; J. T. Wrobel, in "The Alkaloids", ed. by R. H. F. Manske, New York, 1977, Vol. 16, p. 181; J. Cybulski and J. T. Wrobel, in "The Alkaloids", ed. by A. Brossi, New York, 1989, Vol. 35, p. 215 and references cited therein.
32. F. A. Carey and A. S. Court, J. Org. Chem., 1972, 37, 1926. CrossRef
33. B. Lal, B. N. Pramanik, M. S. Manhas, and A. K. Bose, Tetrahedron Lett., 1977, 1977. CrossRef
34. T. Honda, S. Yamane, K. Naito, and Y. Suzuki, Heterocycles, 1995, 40, 301. CrossRef
35. Recent synthesis of (±)-heliotridane, see: (a) P. G. Andersson and J. E. Bäckvall, J. Am. Chem. Soc., 1992, 114, 8696; CrossRef (b) S.-H. Kim, S.-I. Kim, S. Lai, and J. K. Cha, J. Org. Chem., 1999, 64, 6771; CrossRef (c) J. Robertson, M. A. Peplow, and H. Pillai, Tetrahedron Lett., 1996, 37, 5825; CrossRef (d) R. K. Dieter and R. Watson, Tetrahedron Lett., 2002, 43, 7725; CrossRef (e) X. L. M. Despinoy and H. McNab, Org. Biomol. Chem., 2009, 7, 4502 and references cited therein; CrossRef Recent synthesis of (-)- or (+)-heliotridane, see: (f) J. G. Knight and S. V. Ley, Tetrahedron Lett., 1991, 32, 7119; CrossRef (g) S. Le Coz, A. Mann, F. Thaueau, and M. Taddei, Heterocycles, 1993, 36, 2073; CrossRef (h) P. F. Keusenkothen and M. B. Smith, J. Chem. Soc., Perkin Trans. 1, 1994, 2485; CrossRef (i) M. P. Doyle and A. V. Kalinin, Tetrahedron Lett., 1996, 37, 1371; CrossRef (j) I. L. Lysenko and O. G. Kulinkovich, Russian J. Org. Chem., 2005, 41, 70; CrossRef (k) R. K. Dieter, N. Chen, and R. T. Watson, Tetrahedron, 2005, 61, 3221. CrossRef
36. S. N. Maiti, P. Spevak, and A. V. N. Reddy, Synth. Commun., 1988, 18, 1201. CrossRef
37. N. J. Leonard and D. L. Felley, J. Am. Chem. Soc., 1950, 72, 2537. CrossRef
38. M. Mori, N. Kanda, I. Oda, and Y. Ban, Tetrahedron, 1985, 41, 5465. CrossRef
39. T. Honda, S. Yamane, F. Ishikawa, and M. Katoh, Tetrahedron, 1996, 52, 12177. CrossRef
40. (a) J. R. Rocca, J. H. Tumlinson, B. M. Glancy, and C. S. Lofgren, Tetrahedron Lett., 1983, 24, 1889; CrossRef (b) J. R. Rocca, J. H. Tumlinson, B. M. Glancy, and C. S. Lofgren, Tetrahedron Lett., 1983, 24, 1893. CrossRef
41. K. Mori and Y. Nakazono, Tetrahedron, 1986, 42, 6459. CrossRef
42. F. E. Ziegler and J. K. Thottathil, Tetrahedron Lett., 1982, 23, 3531. CrossRef
43. For the synthesis of racemic invictolide, see: (a) T. R. Hoye, D. R. Peck, and P. K. Thrumper, J. Am. Chem. Soc., 1981, 103, 5618; CrossRef (b) T. R. Hoye, D. R. Peck, and T. A. Swanson, J. Am. Chem. Soc., 1984, 106, 2738; CrossRef (c) S. L. Schreiber and Z. Wang, J. Am. Chem. Soc., 1985, 107, 5303; CrossRef (d) Y. Yamamoto, K. Taniguchi, and K. Maruyama, J. Chem. Soc., Chem. Commun., 1985, 1429; CrossRef For the synthesis of optically active invictolide, see: (e) F. E. Ziegler, E. P. Stirchak, and R. T. Wester, Tetrahedron Lett., 1986, 27, 1229; CrossRef (f) K. Mori and S. Senda, Agric. Biol. Chem., 1987, 51, 1379; CrossRef (g) T. Wakamatsu, Y. Nishikimi, H. Kikuiri, H. Nakamura, and Y. Ban, Heterocycles, 1987, 26, 1761; CrossRef (h) R. W. Hoffman, K. Ditrich, G. Koster, and R. Sturmer, Chem. Ber., 1989, 122, 1783. CrossRef
44. J. W. Coe and W. R. Roush, J. Org. Chem., 1989, 54, 915. CrossRef
45. F. György, Helv. Chim. Acta, 1979, 62, 2825 and references cited therein. CrossRef
46. W. C. Still and C. Gennari, Tetrahedron Lett., 1983, 24, 4405. CrossRef
47. T. Honda, F. Ishikawa, and S. Yamane, J. Chem. Soc., Perkin Trans. 1, 1996, 1125. CrossRef
48. (a) T. Wrobel, The Alkaloids: Pyrrolizidine Alkaloids, ed. by A. Brossi, Academic Press, New York, 1985, vol. 26, pp. 327-384; (b) A. R. mattocks, Chemistry and Toxicology of Pyrrolizidine Alkaloids, Academic Press, London, 1986.
49. E. K. McLean, Pharmacol. Rev., 1970, 22, 429.
50. (a) T. Kametani, K. Higashiyama, H. Otomasu, and T. Honda, Isr. J. Chem., 1986, 27, 57; (b) T. Kametani, H. Yukawa, and T. Honda, J. Chem. Soc., Perkin Trans. 1, 1988, 833; CrossRef (c) T. Kametani, H. Yukawa, and T. Honda, J. Chem. Soc., Perkin Trans. 1, 1990, 571; CrossRef (d) T. Honda, K. Tomitsuka, and M. Tsubuki, J. Org. Chem., 1993, 58, 4274. CrossRef
51. P. A. Grieco, S. Gilman, and M. Nishizawa, J. Org. Chem., 1976, 41, 1485. CrossRef
52. P. H. J. Carlsen, T. Katsuki, V. S. Martin, and K. B. Sharpless, J. Org. Chem., 1981, 46, 3936. CrossRef
53. J. D. White, J. C. Amedio, Jr., S. Gut, S. Ohira, and L. R. Jayasinghe, J. Org. Chem., 1992, 57, 2270. CrossRef
54. T. Honda and F. Ishikawa, J. Org. Chem., 1999, 64, 5542. CrossRef
55. Only one synthesis of racemic nemorensic acid has been reported before our synthesis: L. L. Klein, L. L. J. Am. Chem. Soc., 1985, 107, 2573; CrossRef Recent syntheses of racemic and (+)-nemorensic acid, see: (a) J. R. Rodriguez, A. Rumbo, L. Castedo, and J. L. Mascarenas, J. Org. Chem., 1999, 64, 4560; CrossRef (b) T. J. Donohoe, J.-B. Guillermin, C. Frampton, and D. S. Walter, Chem. Commun., 2000, 465; CrossRef (c) F. Lopez, L. Castedo, and J. L Mascarenas, Chem. Eur. J., 2002, 8, 884; CrossRef (d) T. J. Donohoe, J.-B. Guillermin, C. Frampton, and D. S. Walter, J. Chem. Soc., Perkin Trans. 1, 2002, 1369; CrossRef (e) D. M. Hodgson, T. D. Avery, and A. C. Donohue, Org. Lett., 2002, 4, 1809; CrossRef (f) D. M. Hodgson, F. Le Strat, T. D. Avery, A. C. Donohue, and T. Brueckl, J. Org. Chem., 2004, 69, 8796; CrossRef (g) J. Y. Sim, G.-S. Hwang, K. H. Kim, E. M. Ko, and D. H. Ryu, Chem. Commun., 2007, 5064. CrossRef
56. M. P. Dillon, N. C. Lee, F. Stappenbeck, and J. D. White, J. Chem. Soc., Chem. Commun., 1995, 1645 and references therein. CrossRef
57. A. Klasek, P. Sedmera, A. Boeva, and F. Santavy, Collect. Czech. Chem. Commun., 1973, 38, 2504.
58. H. Ohrui, G. H. Jones, J. G. Moffatt, M. L. Maddox, A. T. Christensen, and S. K. Byram, J. Am. Chem. Soc., 1975, 97, 4602. CrossRef
59. B. S. Bal, W. E. Childers, Jr., and H. W. Pinnick, Tetrahedron, 1981, 37, 2091. CrossRef
60. N. T. Nghia, P. Sedmera, A. Klasek, A. Boeva, S. Dvorakova, and F. Santavy, Collect. Czech. Chem. Commun., 1976, 41, 2952.
61. T. Honda, M. Katoh, and S. Yamane, J. Chem. Soc., Perkin Trans. 1, 1996, 2291. CrossRef