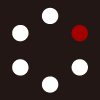
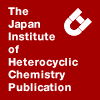
HETEROCYCLES
An International Journal for Reviews and Communications in Heterocyclic ChemistryWeb Edition ISSN: 1881-0942
Published online by The Japan Institute of Heterocyclic Chemistry
e-Journal
Full Text HTML
Received, 7th October, 2010, Accepted, 8th November, 2010, Published online, 19th November, 2010.
DOI: 10.3987/REV-10-684
■ Development of Samarium Diiodide-Promoted Reductive Carbon-Nitrogen Bond Cleavage Reaction of α-Amino Carbonyl Compounds: Application to the Synthesis of Biologically Active Alkaloids
Toshio Honda*
Faculty of Pharmaceutical Sciences, Hoshi University, 2-4-41 Ebara, Shinagawa-ku, Tokyo 142-8501, Japan
Abstract
Samarium diiodide (SmI2) is a mild and selective one electron transfer reagent, and has become an important tool for developing a variety of useful and unique transformations. SmI2 has been utilized in a wide range of synthetic transformations ranging from interconversion of functional groups to carbon-carbon bond forming reactions. Among the various reactions developed for SmI2, we focused our attention on its use for fragmentation reactions. We have already established a regioselective carbon-carbon bond cleavage reaction of γ-halo carbonyl compounds, and its utilization in the synthesis of various types of biologically active natural products. However, a SmI2-promoted reductive carbon-nitrogen bond cleavage reaction has received relatively little attention. In this review article, we would like to describe a general carbon-nitrogen bond cleavage reaction of α-amino carbonyl compounds and the utilization of this methodology in the synthesis of a number of bioactive alkaloids, since this reaction proceeds in relatively high yield under mild reaction conditions.CONTENTS
1. INTRODUCTION
2. GENERAL CARBON-NITROGEN BOND CLEAVAGE REACTION
3. FIRST GENERATION SYNTHESIS OF (-)-ADALININE
4. SYNTHESIS OF (+)-FRBRIFUGINE AND (-)-ISOFRBRIFUGINE
5. NOVEL CHIRAL SYNTHESIS OF INDOLIZIDINE AND QUINOLIZIDINE ALKALOIDS:
SYNTHESIS OF (+)-(8R, 8aR)-PERHYDRO-8-INDOLIZIDINOL AND (-)-DEOXYNUPHARI- DINE
6. SYNTHESIS OF DIPIPERIDINE TYPE ALKALOIDS, (+)-CYTISINE, (-)-KURARAMINE, (-)-ISO KURARAMINE, AND (-)-JUSSIAEIINE A
7. SYNTHESIS OF (+)-APHANORPHINE
8. SYNTHESIS OF BULGARANINE
9. SECOND GENERATION SYNTHESIS OF (-)-ADALININE
10. CONCLUSION
1. INTRODUCTION
Samarium diiodide (SmI2), probably the most versatile lanthanide compound, was firstly introduced as a useful synthetic tool in organic synthesis by Kagan and co-workers,1 thereafter, this reagent rapidly became an established reagent for developing a variety of useful and unique transformations.2 Due to the characteristic feature of samarium(II) to revert to the more stable samarium(III) oxidation state, SmI2 is recognized to be a mild and selective one-electron transfer reagent and has been utilized in a wide range of synthetic transformations ranging from interconversion of functional groups to carbon-carbon bond forming reactions.
Among the various reactions developed for SmI2, we focused our attention on its use for fragmentation reactions. Regarding the fragmentation reactions employing SmI2 as a powerful one-electron reducing reagent, much effort has been devoted to studying the reductive deoxygenation of α-hydroxy or α−alkoxy carbonyl compounds, however, its application to a regioselective carbon-carbon or carbon-nitrogen bond cleavage reaction has received relatively little attention. We have already established a regioselective carbon-carbon bond cleavage reaction of γ-halo carbonyl compounds, and this strategy was utilized in the synthesis of various types of natural products3 (Scheme 1).
In continuation of our work on the synthesis of biologically active natural products using SmI2, we were interested in researching a general carbon-nitrogen bond cleavage reaction of α-amino carbonyl compounds (Scheme 2), and are able to obtain successful results concerning systematic investigation of SmI2-promoted reductive carbon-nitrogen bond cleavage reaction.4
2. GENERAL CARBON-NITROGEN BOND CLEAVAGE REACTION4
Attractive examples of SmI2-promoted carbon-nitrogen bond cleavage reaction were reported by Molander and Stengel using 2-acylaziridines and 4-acylazetidin-2-one as starting materials (Figure 1),5 in which the leaving amino groups (nitrogen-containing groups) were involved in highly strained three- or four-membered rings. Similar fragmentation reactions were also employed in the reductive removal of an N-substituted benzotriazolyl group,6 and in the isonitrile–nitrile rearrangement.7
Initially, we applied the SmI2-promoted carbon-nitrogen bond cleavage reaction to phenylalanine derivatives,8 and the results obtained are summarized in Table 1.
Based on the above results, it was concluded that reductive carbon-nitrogen bond cleavage reaction with SmI2 has a generality and is applicable to the wide variety of amino functions including primary, secondary and tertiary amines, and also amide groups as leaving groups. The fragmentation usually took place within 30 min in the presence of a proton source, such as MeOH or pivalic acid, to provide methyl dihydrocinnamate in high yields, although a relatively prolonged reaction time was required in the case of benzyl-substituted amines as leaving groups. The reactions of proline derivatives9 (Table 2) and ethyl pipecolinate derivatives10 (Table 3), in which the leaving amino groups were involved in the cyclic systems, under the same reaction conditions as above gave the corresponding fragmentation products in good yields. The fragmentation could be applied not only to α-amino esters but also to α-amino ketones. Thus, treatment of α-acetylpiperidine derivatives11 with SmI2 also furnished the desired compounds, in high yields, in which both alkyl and acyl derivatives of amines could be used as leaving groups (Table 3). Interestingly, the reaction of N-(2-oxopropyl)phthalimide with SmI2 in THF–HMPA in the presence of MeOH yielded phthalimide in 72% yield (Table 3). This result suggested that 2-oxopropyl group might be used as a protecting group for a nitrogen atom of amino-, amide, or imide-functions.
We next investigated the effect of proton sources, and found that N,N-dimethylaminoethanol (DMAE) was also effective for this reaction as well as MeOH and pivalic acid (Table 4). It should be noted that this reaction can be carried out under neutral reaction conditions in the presence of other functional groups, such as alkyl ester, alkyl ether, imide and amide groups. Moreover, this reaction proceeded in the presence or absence of HMPA, however, the presence of HMPA proved desirable in terms of yields and reaction times (Tables 2, 3 and 4). These results were in agreement with those observed in the reductive deoxygenation reactions, since HMPA was recognized to increase the rate of the reaction of SmI2.12 As can be seen in Table 2, the fragmentation product bearing a primary amino function sometimes afforded the cyclization compound. This type of conversion will provide a useful route for the synthesis of naturally occurring or biologically interesting piperidine derivatives in optically active forms. Indeed, heating of isopropyl δ-amino-γ-tert-butyldimethylsiloxyvalerate in THF for 2 days gave 5-tert-butyl- dimethylsiloxy-2-piperidone in 75% yield.
Thus, we were able to develop a general reductive carbon-nitrogen bond cleavage reaction employing SmI2 in THF–HMPA. The proposed reaction mechanism was shown in Scheme 3.
Since this reaction proceeds in relatively high yield under mild reaction conditions and seems to be applicable to the wide variety of α-amino carbonyl compounds, we planned its utilization for the synthesis of (-)-adalinine.13
3. FIRST GENERATION SYNTHESIS OF (-)-ADALININE14
(-)-Adalinine 1, isolated from the secretion of the European two-spotted ladybird beetle, Adalia bipunctata, as a minor component, together with major alkaloid (-)- adaline 2, is a simple piperidine alkaloid with a chiral quaternary carbon center, and the structure of 1 was determined by spectroscopic methods.15 This alkaloid was shown to be present in all of the life cycle stages of Adalia bipunctata, as well as in the adults of a related species, A. decempunctata.15 Adalinine 1 has also been proposed to be biosynthetically derived from the major alkaloid adaline (2) via a retro-Mannich reaction15 (Figure 2).
The relatively simple alkaloid 1 seems to be the target molecule for the application of newly developed synthetic methods and strategies. Consequently, one chiral synthesis16 of 1 leading to the determination of its absolute configuration and three syntheses of its racemate17 have been reported.
Our devised retrosynthetic route to (-)-adalinine (1) is depicted in Figure 3, in which we envisaged that a chiral quaternary carbon center could be constructed with the desired stereochemistry via Michael addition of a pentyl group to enaminone A, readily accessible from (S)-(-)-pyroglutamic acid, by the control of the stereochemistry of the ester function on the pyrrolidine ring. Moreover, a ring enlargement of pyrrolidine derivative B to δ-lactam would easily be achieved by application of a samarium-promoted fragmentation reaction, followed by recyclization of the resulting amino ester C.
Thus, the requisite optically active pyrrolidine derivative bearing a chiral quaternary carbon center was prepared starting from thiolactam (3).18 Treatment of 3 with bromoacetone and subsequent desulfurization of thioether (4) with triphenylphosphine19 gave (Z)-enaminone (5). After protection of the amino group of 5, the resulting (E)-Boc-enaminone (6) was subjected to Michael addition with pentylmagnesium bromide in the presence of a copper sulfide-dimethyl sulfide complex20 to provide the desired pyrrolidine (7).
Although the stereochemistry of the newly generated chiral center could not be determined at this stage, it was assumed that the major product (7) should have the correct stereochemistry for natural product synthesis, since Michael addition of a pentyl group would be expected to take place from the less hindered side of enaminone (6). With the desired pyrrolidine derivative available, we first investigated a samarium-promoted carbon-nitrogen bond cleavage reaction for keto ester (7) or its de-Boc derivative (8); however, none of the desired fragmentation product could be obtained. We thought that this result might be caused by the presence of another reactive site, such as a ketone carbonyl function (Scheme 4).
Ketone (7) was, therefore, reduced with NaBH4 to give alcohol (9) as an inseparable diastereoisomeric mixture (ca. 1:1). After deprotection of the Boc group on treatment with TFA, the resulting alcohol (10) was further converted to silyl ether (11) in the usual manner (Scheme 5). Attempted fragmentation reaction of 11 with 5 equiv of samarium iodide in THF-HMPA (7:1) in the presence of pivalic acid as a proton source took place the carbon-nitrogen bond cleavage smoothly, and simultaneous cyclization of the resulting δ-amino ester to give the desired δ-lactam (12) in 70% yield.21 In our previous study on this fragmentation reaction,4 a co-solvent HMPA usually required only 5 equiv; however, it was found that the use of a smaller amount of HMPA or its absence in the conversion of 11 to 12 decreased the yield, remarkably. Finally, desilylation of 12 on acid hydrolysis with hydrochloric acid, followed by oxidation of the resulting alcohol (13) with TPAP and NMO according to the reported procedure16 gave (-)-adalinine 1 (Scheme 5). Spectroscopic data (1H and 13C NMR, MS, IR) of 1 including specific optical rotation {[α]D -30.4 (c 0.8, CH2Cl2); lit.,16 [α]D -28.3 (c 1.6, CH2Cl2)} were identical with those reported.16
Thus, we were able to apply a samarium diiodide-promoted reductive carbon-nitrogen bond cleavage reaction to the chiral synthesis of 1 successfully.
4. SYNTHESIS OF (+)-FRBRIFUGINE AND (-)-ISOFRBRIFUGINE 22
Febrifugine (14) and isofebrifugine (15) were isolated from the roots of Dichroa febrifuga Lour. (Chinese Name: Cháng Shan) in 1947,23 and are recognized as active principles against malaria.24 Structures including the absolute stereochemistries of those alkaloids were unambiguously established by their syntheses in 1999 (Figure 4).25
Since these alkaloids exhibit the attractive biological activity, a number of their racemic and chiral syntheses have been established.26 Applying SmI2-promoted carbon-nitrogen bond cleavage reaction of α-amino carbonyl compounds, we attempted the synthesis of potential anti-malarial piperidine alkaloids, febrifugine (14) and isofebrifugine (15).
Our synthesis of (+)-febrifugine starts from the stereoselective preparation of 4-hydroxy-5-alkyl-L- prolinate, as follows.
Oxidation of methyl N-Boc-(4S)-tert-butyldimethylsilyloxy-L-prolinate (16) with ruthenium tetroxide according to the literature gave lactam (17).27 In order to synthesize (+)-febrifugine, a stereoselective introduction of an alkyl side chain at the 5-position of 17 with the 2,5-trans-stereochemistry would be required. Although a number of strategies have been developed for stereoselective introduction of an alkyl side chain at the 5-position of pyroglutamic acid ester,28 some of them were found to have a limitation, in terms of nucleophilic species, conversion yield, reaction conditions, and stereoselectivity. Among the various reaction conditions attempted, we could find that a tandem Horner-Emmons-Michael reaction29 gave the best results for introducing the desired alkyl side chain, in terms of yield and stereoselectivity. Thus, lactam (17) was reduced with lithium triethylborohydride to give aminal (18), which without purification, was treated with triethylphosphonoacetate in the presence of sodium hydride to afford diester (19) in 62% from 17. Although diester (19) was obtained as a mixture of the amide rotamers, the stereochemistry at the 5-position was assumed to be R-configuration based on the analysis of the NMR spectral data. To determine the stereoselectivity of this reaction, the N-Boc group of 19 was removed by treatment with trifluoroacetic acid to give amine (20) as the sole product. The spectroscopic data of 20 clearly indicated that a tandem Horner-Emmons-Michael reaction proceeded, stereoselectively, and the stereochemistry at the 5-position of 19 would be controlled during the intramolecular Michael addition of the nitrogen to the α,β-unsaturated ester, generated by the Horner-Emmons reaction, where the addition of the nitrogen might occur from the sterically less hindered side of the substrate.29b As the difficulties were mentioned for the selective reduction of the ethyl ester to manipulate the side chain prior to the reduction of the methyl ester, we attempted to prepare a more versatile precursor for further modification of the side chain.
Treatment of 18 with diethyl (N-methoxy-N-methylcarbamoylmethyl)phosphonate in the presence of sodium hydride provided amide-ester (21) in 83% from 17. The configuration at the 5-position of 21 was assumed to be R based on the analyses of NOE experiments, and was unambiguously determined by the X-Ray crystallographic analysis of the corresponding NH compound (22) (Scheme 6).
Since we could achieve the introduction of the side chain at the 5-position with the desired stereochemistry, we focused our attention on further utilization of amide-ester (21) in the synthesis of (+)-febrifugine. Treatment of 21 with methylmagnesium bromide gave ketone (23), as the sole product, which was further converted into olefin (24) by methylenation with the Wittig reagent in 43% yield.
Olefin (24) was also synthesized by treatment of 23 with Tebbe’s reagent30 in an improved yield. After selective removal of the Boc group of 24 with zinc bromide, the resulting amine (25) was subjected to the key reductive fragmentation reaction by using SmI2 in the presence of MeOH as the proton source to give δ-lactam (26) arising from a recyclization of the carbon-nitrogen bond cleavage product, in 90% yield. Lithium aluminum hydride reduction of 26, followed by treatment of the resulting hydroxy-amine (27) with CbzCl afforded carbamate (28), where deprotection of the silyl group was observed during the reduction of the amide function. Protection of the secondary hydroxy group of 28 with benzyl bromide in the presence of sodium hydride gave benzyl ether (29), which, on ozonolysis, followed by the usual reductive work-up with methyl sulfide, furnished methyl ketone (30) in 83% yield from 28 (Scheme 7). Bromination of 30 was achieved by treatment with trimethylsilyl triflate and subsequently with N-bromosuccinimide to give bromo-ketone (31), which, without purification, was further coupled with 4-hydroxyquinazoline in the presence of potassium hydride in DMF to provide the protected febrifugine (32) (Scheme 8).
Finally, deprotection of 32 with 6N hydrochloric acid furnished (+)-febrifugine (14) in 92% yield. The spectroscopic data of the synthesized compound including the specific optical rotation were comparable to those reported in the literatures, mp 139-140 °C; [α]D +16.0 (c 0.4, MeOH) {lit.,23b mp 139-140 °C; lit.,26b mp 138-139 °C; lit.,23c [α]D +13.0 (c 0.65, MeOH)}. Thus, we could develop a novel synthetic pathway to (+)-febrifugine by employing SmI2-promoted reductive carbon-nitrogen bond cleavage, as a key reaction. Since this strategy is applicable to the construction of functionalized piperidine rings in optically active forms, we next attempt the stereoselective synthesis of (-)-isofebrifugine, an antipodal form of the natural product, due to the accessibility of a starting material.
For the synthesis of (-)-isofebrifugine, an introduction of an alkyl side chain at the 5-position of 4-hydroxypyroglutamate would be required with the relative stereochemistry of 2,5-trans- and 4,5-cis-relationships (Scheme 9).
Thus, a commercially available (4R)-hydroxy-L-proline was converted into the known N-Boc methyl ester (33),27 which on oxidation with ruthenium tetroxide, gave lactam (34) in 83% yield. Reduction of the lactam (34) with lithium triethylborohydride as described above, afforded aminal (35). A tandem Horner-Emmons-Michael reaction of 35 with triethyl phosphonoacetate and sodium hydride in THF gave an inseparable mixture of diastereomers (36 and 37), which, on deprotection of the N-Boc group with trifluoroacetic acid gave separable two compounds (38 and 39) in 77% yield, in a ratio of ca. 1 : 2. The structures of these compounds (38 and 39) were determined by treatment with tetrabutylammonium fluoride to furnish alcohol (40) and lactone (41), respectively (Scheme 10).
When a tandem Horner-Emmons-Michael reaction of 35 was carried out by using diethyl (N-methoxy-N-methylcarbamoylmethyl)phosphonate as the Wittig reagent, the ratio of the products (42 and 43) was improved to ca. 1 : 11, where the desired compound (43) was found to be the major product. After separation by silica gel column chromatography, those amides (42 and 43) were converted into the secondary amines, respectively, as follows. Diisobutylaluminum hydride reduction of amides (42 and 43), followed by methylenation of the resulting aldehydes (44 and 45) with methyltriphenylphosphonium bromide and n-butyllithium, provided olefins (46 and 47).
Selective removal of the N-Boc groups of 46 and 47 was achieved by treatment with zinc bromide as described above to give amines (48 and 49), respectively. With the desired compound (49) in hands, we attempted its conversion into the corresponding δ-lactam by means of samarium diiodide-promoted reductive fragmentation reaction. Treatment of 49 with samarium diiodide in the presence of methanol as the proton source in THF-HMPA afforded the desired δ-lactam (50) in 71% yield. Again, spectroscopic data of 50 were identical with those reported in the literature26m except for the sign of optical rotation, {[α]D +4.6 (c 0.2, CHCl3); lit.,26m [α]D -4.5 (c 1.05, CHCl3)}. Since ent-50 was already transformed into (+)-isofebrifugine (15),26h this synthesis constitutes the formal synthesis of (-)-isofebrifugine (Scheme 11). Again, we were able to establish novel chiral syntheses of (+)-febrifugine and (-)-isofebrifugine. Although the synthesis of isofebrifugine gave the antipodal form of the natural product, we believe that the strategy developed here should provide a useful tool for finding new anti-malarial drugs.
5. NOVEL CHIRAL SYNTHESIS OF INDOLIZIDINE AND QUINOLIZIDINE ALKALOIDS: SYNTHESIS OF (+)-(8R, 8aR)-PERHYDRO-8-INDOLIZIDINOL AND (-)-DEOXYNUPHARI- DINE31
Indolizidine and quinolizidine alkaloids, with their wide range of structural and stereochemical features, are large class of natural products that have, over the year, provoked an extraordinary amount of activity by synthetic organic chemists.32 As mentioned above, we have developed a SmI2-promoted reductive carbon-nitrogen bond cleavage reaction of α-amino esters, where proline derivatives were successfully transformed to the corresponding δ-lactams in good yields. The effectiveness of this reaction for the construction of ring-enlarged lactams leads us to consider its further application toward the indolizidine and quinolizidine alkaloids in optically active forms.
Our synthesis of indolizidine alkaloid commenced with the synthesis of the known proline derivative (47), which was readily accessible via known procedures from (4R)-hydroxy-L-proline. Hydroboration of 47 with 9-BBN in THF, followed by oxidative treatment with alkaline hydrogen peroxide gave the primary alcohol (51). Removal of the Boc group of 51 on treatment with zinc bromide33 in CH2Cl2 afforded the desired amino-alcohol (52) together with tert-butyl ether (53), in 71 and 15% yields, respectively (Scheme 12).
The structure of 53 was confirmed by its alternative preparation from 51 involving an etherification34 with (Boc)2O and Mg(ClO4)2, followed by deprotection of the N-Boc group of the resulting ether (54) with zinc bromide in CH2Cl2 (Scheme 13). In order to synthesize the bicyclic compound, the precursor for a reductive carbon-nitrogen bond cleavage reaction, the primary alcohol (52) was reacted with carbon tetrabromide in the presence of triphenylphosphine in CH2Cl2 to give the alkoxyphosphonium salt, which on treatment with triethylamine (TEA) furnished the bicyclic compound (56) in 74% yield from 52.35 Compound (56) was alternatively prepared from 51 by mesylation with methanesulfonyl chloride in CH2Cl2, followed by deprotection of the N-Boc group of mesylate (55) with zinc bromide in CH2Cl2, in 86% overall yield.
Since the desired bicyclic ester (56) in hands, we focused our attention on its conversion to the corresponding δ-lactam by means of a SmI2-promoted reductive carbon-nitrogen bond cleavage reaction. Treatment of 56 with SmI2 in THF-HMPA in the presence of MeOH as the proton source at 0 °C to room temperature for 2 h provided indolizidinone (57) in 86% yield, where a reductive carbon-nitrogen bond cleavage, followed by recyclization of the resulting amino-ester occurred, simultaneously. Deprotection of the silyl group of 57 with tetrabutylammonium fluoride (TBAF) in THF afforded hydroxy-lactam (58),36 which was further converted to (+)-(8R, 6aR)-perhydro-8-indolizidinol (59) by reduction with borane-methylsulfide complex according to the known procedure36 The spectroscopic data of 10 were identical with those reported in the literature36 except for the sign of optical rotation {[α]D +19.9 (c=1.2, CHCl3); lit.,36 [α]D –17.4 (c=1.15, CHCl3)} (Scheme 14).
Since we were able to establish a new synthetic procedure for indolizidine alkaloids by employing a SmI2-promoted reductive carbon-nitrogen bond cleavage reaction as a key step, we planned to extend the utilization of this strategy to the synthesis of quinolizidine alkaloids.
As a model study, a synthesis of 1-hydroxy-4-quinolizidinone was investigated as follows.
Removal of the N-Boc group of 47 with zinc bromide in CH2Cl2, followed by allylation of the resulting amine with allyl bromide in the presence of sodium hydride in DMF gave the N-allyl compound (60) in 80% from 47. Ring closing metathesis (RCM)37 of 60 with 2 mol% of Grubbs’ 2nd generation catalyst in refluxing benzene provided the olefinic bicyclic compound (61), which on catalytic reduction over palladium on carbon in AcOEt gave the saturated bicyclic compound (62). Application of a SmI2-promoted reductive carbon-nitrogen bond cleavage reaction to 62 under the same reaction conditions as described for the synthesis of 59 furnished the desired quinolizidinone (63) in 86% yield. Deprotection of the silyl group of 63 with tetrabutylammonium fluoride in THF gave hydroxy-lactam (64). Although the synthesis of lactam (64) has already been achieved, none of its spectroscopic data were reported in the literature,38 unfortunately (Scheme 15).
Applying this strategy, we started the synthesis of biologically active quinolizidine alkaloids.
(-)-Deoxynupharidine (65) and (-)-7-epi-deoxynupharidine (66), isolated from plants of the genus Nuphar,39 are sesquiterpene alkaloids based on a quinolizidine core with a furan ring. The dried rhizomes of Nuphar japonicum DC. and Nuphar pumilum (TIMM.) DC., are used in folk medicine for tonic, haemostatic and diuretic purposes in China and Japan. It has also been recognized that (-)-deoxynupharidine exhibited an immunosuppressive effect,40 a central paralysis effect41 and weak anti-metastitic activity,42 and (-)-7-epi-deoxynupharidine showed insecticidal activity39 (Figure 5).
Although several syntheses of deoxynupharidine (65) have been achieved, most of them led to the synthesis of the target compound in racemic form.43 Very recently, Harrity and co-workers published a stereocontrolled chiral synthesis of 65 by employing a formal [3+3] cycloaddition reaction to obtain a piperidine nucleus.44
Our synthesis starts from a stereoselective construction of the 1-methyl-4-quinolizidinone ring system in an optically active form.
Thus, methyl N-tert-butoxycarbonylpyroglutamate (67), readily accessible from commercially available (R)-pyroglutamic acid, was reacted with Bredreck’s reagent45 in toluene at 100 °C to give enaminone (68). Catalytic reduction of 68 over 10% palladium on carbon in iPrOH-AcOEt afforded the methylated pyroglutamates (69 and 70), in 3 and 91% yields, respectively. Reduction of the major product (70) with lithium triethylborohydride in THF at -78 °C gave aminal (71), which, on treatment with diethyl (N-methoxy-N-methylcarbamoylmethyl)phosphonate46 in THF in the presence of sodium hydride furnished amide (72) in 96% yield from 70. The stereochemistry at the 5-position of 72 was assumed to be S, by comparison of the NMR data of 73 with those of the related compounds prepared by us previously,31 and finally determined by its conversion to the natural product (Scheme 16).
Chemoselective reduction of 72 with DIBAL provided the corresponding aldehyde (74), which was treated with methyltriphenylphosphonium bromide in the presence of sodium hexamethyldisilazide to afford alkene (75). After removal of the N-Boc group of 75 with trifluoroacetic acid in CH2Cl2, the resulting amine (76) was subjected to a SmI2-promoted reductive carbon-nitrogen bond cleavage reaction as described before to provide the ring enlarged lactam (77) in 90% yield from 75. Alkylation of 77 with 3-bromo-2-methylpropene in the presence of sodium hydride in DMF, followed by RCM of the resulting olefin (78) with 2 mol% of Grubbs’ 2nd generation catalyst in refluxing benzene for 2 h afforded the bicyclic lactam (79). Lactam (79) was alternatively synthesized from 75, in which the construction of bicyclo-system was carried out prior to a samarium diiodide-promoted fragmentation, as follows (Scheme 17).
Removal of the N-Boc group of 75 with trifluoroacetic acid in CH2Cl2, followed by alkylation with 3-bromo-2-methylpropene as described before gave the alkene (80). RCM of 80 with 2 mol% of Grubbs’ 2nd generation catalyst in refluxing benzene afforded the bicyclic ester (81). Again, application of a SmI2-promoted carbon-nitrogen bond cleavage reaction to 81 furnished the desired lactam (79) in 82% yield. Thus, this reaction was found to be able to apply to both secondary amine and tertiary amine, successfully. Catalytic reduction of 79 over 10% palladium on carbon in MeOH gave lactam (82) as a mixture of the diastereomers at the 7-position, in a ration of 7S:7R=3.4:1.0. Although lactam (82) has already been converted to (-)-deoxynupharidine by Harrity and his co-workers,44 we decided to adopt an alternative route to (-)-deoxynupharidine, in which we planned to introduce a furyl moiety to the lactam function prior to a reduction of the carbon-carbon double bond.
Treatment of 79 with 3-lithiofuran in THF at -78 °C, followed by treatment with sodium borohydride in methanol afforded a mixture of the furyl compounds (84 and 85). In this conversion, we first attempted DIBAL reduction for the intermediate (83) after installation of a furan ring according to the literature reported by Harrity,44 in which, they proposed a carbinolamine as the intermediate in their synthesis. However, we mentioned that DIBAL was not effective for this reduction, unfortunately, and we could confirm the presence of an enamine as an intermediate by the analysis of its NMR spectra. Our experimental results using sodium borohydride as the reducing agent for the intermediate might support the presence of an enamine (83) as the intermediate in our synthesis (Scheme 18).
Finally, a catalytic reduction of 84 over palladium hydroxide in methanol gave (-)-deoxynupharidine (65) and its 7-epimer (66) in 79% yield, in a ratio of 68:11.
Thus, we were able to synthesize both indolizidine and quinolizidine alkaloids by using a samarium diiodide-promoted reductive carbon-nitrogen bond cleavage, as a key step.
6. SYNTHESIS OF DIPIPERIDINE TYPE ALKALOIDS, (+)-CYTISINE, (-)-KURARAMINE, (-)-ISO KURARAMINE, AND (-)-JUSSIAEIINE A47
Naturally occurring lupine alkaloids having a wide range of structural and stereochemical features, continue to provide challenging synthetic targets.48 Among them, (-)-cytisine (86)49 has received special attention50 and several synthetic methods have been developed,51 since it has been shown to be an important probe in nicotinic acetylcholine receptor research52 and shows high affinity at neuronal nicotinic receptors.53
(+)-Kuraramine (87) and (+)-jussiaeiine A (88), isolated from Sophora flavescens54 and Ulex jussiaei,55 respectively, are dipiperidine-type alkaloids with two chiral centers at the 3 and 5 positions of the piperidine ring. Those alkaloids are known to be oxidative metabolites of N-methylcytisine. Another dipiperidine-type alkaloid, (+)-isokuraramine (89)56 was also isolated from the fresh flowers of Sophora flavescens as a minor constituent, and its structure was determined to be the diastereoisomer of (+)-kuraramine by spectroscopic methods.56 However, little attention has been focused on the chiral synthesis of these alkaloids (Figure 6).
Thus, we planned to establish a novel synthetic route to these alkaloids, including cytisine, via a common synthetic intermediate. The key feature of our synthesis is based on a samarium diiodide-promoted reductive carbon-nitrogen bond cleavage reaction of an α-amino ester, as described above.
Based on a retrosynthetic analysis of these alkaloids as depicted in Scheme 19, we decided to exploit a readily available 4R-hydroxy-L-proline derivative as a starting material to synthesize the enantiomers of the aforementioned natural products, since the use of the antipodal starting material, D-hydroxyproline, should lead to the synthesis of natural products if this synthetic strategy can be established.
Thus, methyl 4-oxo-L-prolinate (90) was converted to the corresponding triflate (91) in three steps. Treatment of 91 with 2-tributylstannyl-6-methoxypyridine (92) in the presence of a palladium catalyst57 afforded the coupling product (93). Hydrogenation of 93 over 10% palladium-charcoal in MeOH gave 94, stereoselectively. After removal of the Boc group, samarium diiodide-promoted reductive fragmentation of the resulting amine (95), followed by simultaneous cyclization of the resulting δ-amino ester was carried out in THF-HMPA in the presence of methanol as a proton source to give the desired common intermediate, the δ-lactam (96) (Scheme 20).
N-Methylation of 96 with iodomethane and sodium hydride in THF-HMPA furnished the corresponding N-methylpiperidone (97), which was further converted into hydroxymethyl derivative as follows.
Treatment of amide (97) with ethyl chlorocarbonate in the presence of LDA in THF gave an inseparable mixture of diastereomers (98 and 99), in a ratio of ca. 1:1. Reduction of the amide and ester functions of the mixture with LiAlH4 in THF afforded amino-alcohols (ent-88 and 100) in respective yields of 50% and 46%. The spectroscopic data of ent-88 obtained here were identical to those reported for (+)-jussiaeiine A. Moreover, the sign of the optical rotation of the synthetic compound corresponded to that of the antipode, {ent-88, [α]D –5.2 (c=0.5, CHCl3); lit.,55 [α]D +3.3 (c=0.26, CHCl3)}. Therefore, we were able to establish the first enantioselective synthesis of (-)-jussiaeiine A. Jussiaeiine A ent-3 was converted into (-)-kuraramine ent-2 by treatment with iodotrimethylsilane in refluxing acetonitrile.58
Again, the physicochemical properties of ent-87 were identical to those reported in the literature, except for the sign of optical rotation59 {ent-87: mp 78-80 °C, [α]D -3.6 (c=2.1, EtOH); lit.,54 [α]D +8.4 (c=0.52, EtOH)}.
The same treatment of the diastereoisomeric compound (100) gave isokuraramine ent-89 as an amorphous solid, [α]D –93.0 (c=2.1, EtOH).56
To achieve the total synthesis of (+)-cytisine from the common intermediate (96) through the formation of a carbon-nitrogen bond, as shown in Scheme 21, N-benzylation of 96 and subsequent ethoxycarbonylation of 101 with ethyl chlorocarbonate in the presence of LDA were carried out to provide a mixture of diastereoisomeric β-ketoesters (102 and 103) in a ratio of ca. 1:1.
Although the attempted isomerization of the mixture to the thermodynamically more stable 3,5-cis-compound under various reaction conditions, such as basic treatment of a mixture of 102 and 103 with lithium diisopropylamide in THF, sodium hydride in appropriate solvents, and 1,8-diazabicyclo- [5.4.0]undec-7-ene (DBU) in benzene, did not improve the ratio, reduction of the mixture with LiAlH4 gave the primary alcohols (104 and 105), in respective yields of 48% and 43%. Finally, mesylation of 104 followed by thermal cyclization of the mesylate yielded tricyclic compound (106). Debenzylation of 106 under the hydrogenolysis conditions furnished ent-86, whose spectroscopic data were identical to those reported in the literature60 except for the sign of optical rotation {ent-1: mp 152-153 °C, [α]D +113.5 (c=0.3, EtOH); lit.,61 mp 152-153 °C, lit.,60 [α]D -110 (c=0.5, EtOH)} (Scheme 21).
In summary, we have established novel and facile syntheses of dipiperidine-type lupine alkaloids, including cytisine. Although these syntheses give the antipodal forms of the natural products, we believe that the strategy developed here should be a useful tool for finding new drugs that are biologically related to cytisine.
7. SYNTHESIS OF (+)-APHANORPHINE62
In the above-mentioned syntheses of bioactive alkaloids, we have applied a SmI2-promoted reductive fragmentation reaction of α-amino esters to proline derivatives yielding the corresponding δ-lactams in good yields, where a regioselective carbon-nitrogen bond cleavage reaction, followed by a recyclization of the resulting amino-esters took place, simultaneously. This reaction is obviously a useful synthetic tool for a construction of ring-enlarged lactams. To extend the usefulness of this strategy, we are interested in a conversion of 1,2,3,4-tetrahydroisoquinoline derivatives having an ester group at the 1- or 3-position to the corresponding benzazepinones.
Thus, (3S)-3-methoxycarbonyl-1,2,3,4-tetrahydroisoquinoline (107)63 was treated with SmI2 in THF-HPMA in the presence of MeOH as the proton source to give the corresponding lactam (108) in 66% yield. Similar reaction of 1-methoxycarbonyl-1,2,3,4-tetrahydroisoquinoline (109)63 also provided the desired lactam (110) together with the ring-opened primary amine (111), in 40 and 49% yields, respectively. The later (111) could be converted to the former (110) by heating in benzene or by treatment with sodium methoxide (Scheme 22).
Since transformation of the isoquinoline derivatives having an ester group at the 1- or 3-position to the corresponding seven-membered lactams was found to be successful by application of a SmI2-promoted reductive carbon-nitrogen bond cleavage reaction, we planned to utilize this strategy to the synthesis of aphanorphine and related compound aiming at searching new analgesic candidates. (-)-Aphanorphine (112), isolated from the freshwater bluegreen alga Aphanizomenon flosaquae in 1988,64 is a natural compound with potent analgesic activity. In 1992, we established a novel synthetic route to (±)-aphanorphine via the amylinium intermediate.65 Thereafter, a formal synthesis of (–)-aphanorphine was also achieved by using an enzymatic process.66
Owing to its interesting biological activity, a number of syntheses of 112 have been reported to date.67
Our synthetic plan for aphanorphine was based on an intramolecular cyclization68 of a seven-membered lactam, as depicted in Figure 7.
In this synthesis, we decided to exploit a readily accessible (S)-tyrosine derivative as a starting material to synthesize the enantiomer of natural aphanorphine,66 since the use of the antipodal starting material, (R)-tyrosine, should lead to the synthesis of the natural product, if this synthetic strategy can be established.
The known compound (112)69 was converted to oxazolidinone (113), which on treatment with ethyl glyoxylate and benzotriazole in the presence of p-toluenesulfonic acid in refluxing toluene afforded ester (114). Reaction of 114 with TiCl4 in acetonitrile70 gave the corresponding isoquinoline derivative (115), as a single stereoisomer, in 76% yield. Hydrolysis of 115 with 2 N NaOH, followed by acidification with 3 N HCl gave acid (116), which, without purification was esterified with thionyl chloride in methanol to provide ester (117) as an inseparable diastereoisomeric mixture, in a ratio of ca. 3:1, in 88% yield from 115. In this conversion, partial epimerization at the 1-position obviously occurred, however, this stereogenic center will be removed at the later stage of this synthesis. Therefore, ester (117) was used without separation in the next step (Scheme 23). In this synthesis, it was recognized that the benzotriazole method developed by Katritzky70 was obviously superior to the other procedures for the preparation of the desired 1,2,3,4-tetrahydroisoquinoline.
After silylation of 117 with tert-butyldimethylsilyl chloride and imidazole in the usual manner, the resulting silyl derivative (118) was subjected to the SmI2-promoted ring expansion reaction in THF-HMPA in the presence of MeOH as the proton source to furnish the desired benzazepinone (119), as the sole product, in 59% yield. Methylation of 119 with iodomethane in the presence of sodium hydride in DMF afforded the N- and C-methylated lactam (120) in 98% yield. Deprotection of the silyl group of 120 with tetrabutylammonium fluoride gave alcohol (121) as a mixture of the diastereomers in a ratio of 3.3:1. The stereochemistry of the C-methyl group of the major product would be assumed to R configuration by comparison of its 1H NMR data with that of the racemic compound.68 The primary alcohol (121) was further converted to mesylate (122) by treatment with methanesulfonyl chloride and triethylamine in 79% yield.
Finally, treatment of 122 with potassium tert-butoxide in refluxing THF gave amide (123), which on reduction with lithium aluminum hydride provided (-)-8-O-methylaphanorphine (124). The spectroscopic data for the synthesized compound (124) including specific optical rotation {[α]D –9.72 (c=0.67, CHCl3); lit.,71 [α]D –7.40 (c=0.35, CHCl3)} were identical with those reported.71 Since (-)-124 was already converted to (+)-aphanorphine (ent-112) by a demethylation,70 this synthesis constitutes its total synthesis (Scheme 24).
We were able to apply this ring expansion reaction to 6,7-dioxygenated 1,2,3,4-tetrahydroisoquinoline derivatives derived from L-dopa, successfully, to provide the corresponding seven-membered lactams. This strategy will provide a useful tool to synthesize aphanorphine-related compounds aiming at searching new analgesic candidates.
8. SYNTHESIS OF BULGARANINE72
Plants of the genus Fumaria have been used in some parts of Asian and eastern European countries as folk medicines for their antipyretic, analgesic, and diuretic properties.73 Because benzindenoazepines are expected to exhibit potential biological activities of medicinal interest, interest in the development of new synthetic methodology for this class of alkaloids continues unabated.74
Bulgaramine (126) was isolated from Herba Fumaria officinalis75 as a member of benzindenoazepines that are a distinct group of alkaloids, probably derived biogenetically from the rearrangement of spirobenzylisoquinolines.76 On the basis of this consideration, a spirobenzylisoquinoline alkaloid, fumaricine (125), has been successfully transformed to bulgaramine, supporting the biogenetic hypothesis77 (Figure 8). Furthermore, benzindenoazepine derivatives have been employed as key intermediates for transformation to rhoeadine and protopine alkaloids.78
Total synthesis of bulgaramine (126) has recently been achieved by Giese and co-workers by using an intramolecular cyclopentannulation of the Fischer aminocarbene complex as a key reaction to give the desired ring system,79 and this is the only report of total synthesis for this alkaloid. The crucial step for synthesis of this class of alkaloids obviously lies in the facile construction of a benzindenoazepine ring system.80 As mentioned above, we developed a SmI2-promoted regioselective carbon-nitrogen bond cleavage reaction of α-amino carbonyl compounds. In relation to a project directed at the synthesis of bioactive alkaloids by application of this methodology, we are interested in establishing an entirely new, perhaps practical and general route for the total synthesis of a unique benzindenoazepine alkaloid, bulgaramine.
Prior to synthesis of the natural product, we investigated efficient and mild reaction conditions for the SmI2-promoted bond cleavage reaction of an ester (128) derived from the known 1-ethoxycarbonyl-2-methyl-6,7-dimethoxy-1,2,3,4-tetrahydroisoquinoline (127)81 by installation of a benzoyl group at the 1-position. Attempted SmI2-promoted reductive carbon-nitrogen cleavage reaction of 128 in THF at ambient temperature for 1 h in the presence of MeOH as a proton source afforded a bond-cleaved compound, which without further purification was subjected to a recyclization in refluxing toluene to give the desired product (129) in 43% yield from 128.82 Thus, we were able to develop a new route for transformation of an isoquinoline skeleton to a functionalized benzazepine ring system by simple reaction sequence (Scheme 25).
By establishing a synthetic route to the basic skeleton of the natural product, we started the synthesis of bulgaramine as follows. Treatment of tetrahydroisoquinoline derivative (127) with 2,3-methylenedioxybenzoyl chloride83 in THF in the presence of NaHMDS in THF afforded the 1-benzoyl derivative (130) in 83% yield. Reductive carbon-nitrogen bond cleavage reaction of 130 with SmI2 (4 equiv) in THF in the presence of MeOH (3 equiv) as the proton source at room temperature for 30 min gave a secondary amine, which on treatment with p-TsOH monohydrate (0.1 equiv) in refluxing toluene furnished the desired benzindenoazepine-type compound (131) directly in 68% yield from 130. It is noteworthy that the bond-cleaved compound was converted to benzindenoazepin-6-one (131) by treatment with p-TsOH in reasonable yield, probably due to the presence of an electron-donating methylenedioxy group on the D-ring, which might facilitate the cyclization of a relatively unstable enamino-ester to the more stable tetracyclic compound (131) (Scheme 26). Actually, when this cyclization was attempted in toluene in the absence of p-TsOH, enamino-ester (133) was isolated in 40% yield as the major product, which could be transformed to 131 in refluxing toluene containing p-TsOH in 81% yield. The plausible reaction mechanism is depicted in Scheme 27.
Finally, reduction of the carbonyl group of 131 at the 6-position was investigated under various reaction conditions. Usually, reduction of enaminones with various hydride donors, such as lithium borohydride, sodium borohydride, zinc borohydride, sodium acetoxyborohydride, or lithium aluminum hydride, provides the corresponding carbon-carbon double bond reduced ketones or corresponding alcohols, but the attempted reduction of 131 under similar reaction conditions did not give any reduced products. Fortunately, we are able to find a practical route for the conversion of 131 to the target compound (126) in which DIBAL was employed as the reducing agent.
Thus, the reduction of ketone (131) with DIBAL (5 equiv) in toluene at -78 °C for 24 h afforded bulgaramine (126), in 24% yield, together with the isomer 13277,79 in 67% yield. Further transformation of 132 to 126 was achieved by treatment with 10% NaOH in EtOH at room temperature for 19 h in 95% yield (Scheme 26).
This synthesis provides a facile and entirely new route for construction of a benzindenoazepine ring skeleton in a small number of steps with reasonably high yield, in which a SmI2-promoted regioselective carbon-nitrogen bond cleavage reaction, followed by acid-catalyzed formation of a benzindenoazepin-6-one ring system, was involved as the key step. By exploiting this strategy, we succeeded in a concise synthesis of a benzindenoazepine alkaloid, bulgaramine, starting from the known tetrahydroisoquinoline derivative (127) in 4 or 5 steps in 50% overall yield.
9. SECOND GENERATION SYNTHESIS OF (-)-ADALININE84
In 1999, we have developed a novel carbon-nitrogen bond cleavage reaction of α-amino carbonyl compounds by using SmI2 as a one-electron transfer reagent.4 Thereafter, this strategy was recognized to be a general and widely applicable synthetic tool for the synthesis of various types of naturally occurring alkaloids, in which proline derivatives have mainly been employed as the chiral starting materials as shown above. The plausible mechanism for the fragmentation was depicted in Figure 9 as exemplified by the reaction of α-amino esters with SmI2, where samarium-containing 5-membered intermediates might be involved as the active species.
To extend the usefulness of this strategy, we decided to apply a SmI2-promoted fragmentation reaction to a 3-oxopyrrolidine derivative with expectation of a bond cleavage between the 1(N) and 2(C) positions, site-selectively, since an obtained product would be a suitable precursor for the synthesis of coccinellid alkaloids adalinine (1) and adaline (2) as shown in Figure 10.
Thus, the precursors having a quaternary carbon center at the 5-position for a SmI2-promoted fragmentation reaction were prepared starting from (4R)-hydroxyproline.
First, we synthesized a 3-oxopyrrolidine derivative (133) possessing (1,3-dioxolan-2-yl)propyl group at the 5-position, as the precursor for the synthesis of (-)-adalinine (1). However, the attempted SmI2-promoted carbon-nitrogen bond cleavage reactions of 133 in appropriate solvent in the presence of several kinds of additives produced an inseparable mixture of the reduction products (134) instead of the desired fragmentation product (135) (Scheme 28).
It would be conceivable, based upon examination of proposed reaction mechanism for a regioselective carbon-nitrogen bond fragmentation of α-amino carbonyl compounds with SmI2, that a participation of samarium metal in forming a cyclic intermediate between carbonyl and amino functions might play an important role to accelerate the fragmentation as shown in Figure 10. However, a formation of such samarium-involved 5-membered intermediate seems to be sterically disfavor for 133 (Figure 11).
Given these considerations, we sought to prepare the known 3-oxopyrrolidine derivative (136) having a functional group at the 5-position that can generate a reasonably tight coordination with samarium metal.
SmI2-promoted carbon-nitrogen bond cleavage reaction of 136 was investigated under several reaction conditions, and the results obtained were summarized in Table 5.
Treatment of 136 with 5 equivalents of SmI2 in THF in the presence of HMPA and MeOH as an additive and a proton source, respectively, at 0 °C for 5 min afforded the secondary alcohols (139) in 88% yield (Entry 1). By changing the proton source to N,N-dimethylaminoethanol (DMEA), the similar reaction gave the fragmentation product with further reduction of the carbonyl group to furnish the secondary amine (138), as an inseparable mixture of the diastereoisomers, in 42% yield (Entry 2).
By using MeOH as a proton source in the absence of HMPA, the desired ketone (137) could be isolated from the reaction mixture in 44% yield. When this reaction was carried out at -78 °C for 3 h, the reduction product (139) was again isolated in 63% yield. Similar reaction at room temperature afforded the reduction product (139) as the sole isolable product. It is reasonably assumed that the reaction of 136 with SmI2 would probably proceed through the chelation intermediate to facilitate the desired fragmentation as depicted in Figure 12.
Next, we attempted to find the best proton source for 5,5-disubstituted 3-oxopyrrolidine derivative having a similar substitution pattern to the target natural product.
First, the fragmentation of 140, prepared from (4R)-hydroxyproline, was carried out by using SmI2 (5.0 equivalents) in THF-HMPA at 0 °C for 15 h, however, only decomposition of the starting material was observed (Entry 1). We note in advance that proton sources play an important role in this fragmentation reaction. We, therefore, decided to find the best proton source in THF solution by screening, and the results obtained were summarized in Table 6.
With the presence of MeOH as the proton donor in THF-HMPA, the reaction of 140 with SmI2 (5.0 equivalents) at 0 °C for 5 min gave the secondary alcohols (142) as an inseparable diastereoisomeric mixture in 85% yield in a ratio of ca. 1:1 (Entry 6). As can be seen in Table 6, almost all of proton sources; such as N,N-dimethylethanolamine (DMEA), tert-BuOH and the combination of those proton sources were also found to be ineffective for this fragmentation (Entries 2, 4, 5, 7-9). When MeOH (2.5 equiv) was employed as the proton source in THF solution, formation of the desired product (141) was observed in a trace amount (< 3%) (Entry 3). However, the yield of 141 could not be improved under the variety of reaction conditions attempted by the use of MeOH, unfortunately. We are very pleased to find that the choice of water as a proton source afforded the desired carbon-nitrogen bond cleavage product (26) in moderate yield (Entry 10). Although the pivotal role was still unclear at present, it has been recognized that the use of water as the proton donor sometimes induced profound impact on variety of factors; such as the reaction rate, mechanism, and stereoselectivity for SmI2-mediated one-electron transfer reactions.85
Since a methoxymethyl protecting group of the primary alcohol in 136 and 140 was found to be effective for the expected fragmentation, we further investigated a possibility of other types of protecting groups expecting a formation of chelation intermediate by employing water as the proton donor. Again, the attempted fragmentation of the compounds (140, 143, 144 and 145) with SmI2 provided the corresponding carbon-nitrogen cleavage products in moderate yields, and the results were summarized in Table 7. Although the exact reason was not clear at present, the use of water as a proton source furnished the desired product in reasonable yields.
These results obviously indicated that formation of a chelation intermediate between samarium metal and heteroatoms on the protecting groups seems to play an important role to take place a SmI2-promoted bond cleavage reaction.
Given these considerations, we assumed that an ester might also serve as a suitable functional group to generate a chelation intermediate with samarium metal. Therefore, we decided to synthesize a precursor having an ester function on the side chain for SmI2-promoted carbon-nitrogen bond cleavage reaction in the synthesis of adalinine. Alkylation of the known ester (33) with amyl iodide in the presence of LiHMDS in THF, followed by reduction of a mixture of diastereoisomeric esters with DIBAL gave the primary alcohols (149 and 150) in 51% and 33% yield, respectively. Oxidation of the major alcohol (149) with SO3-Py and subsequent Wittig reaction of the resulting aldehyde (151) with the phosphonium salt afforded (Z)-olefin (152) in 87% yield. After deprotection of the silyl group of 152 on treatment with TBAF, olefinic diol (153) was hydrogenated over platinum oxide to provide the saturated diol (154). Oxidation of both hydroxyl groups of 154, followed by Pinnick oxidation86 of the resulting aldehyde with NaClO2 gave the corresponding acid. Finally, esterification of the acid with methyl iodide in the presence of potassium carbonate furnished the desired ester (155).
With the requisite carbonyl compound (155) in hand, a SmI2-promoted carbon-nitrogen bond cleavage reaction was attempted. The desired precursor thus obtained was subjected to SmI2-mediated reductive carbon-nitrogen bond cleavage reaction exploiting water as the proton donor by two routes (Scheme 29).
Removal of N-Boc group of ester (155) by treatment with ZnBr2 gave amine (156), which was heated in toluene to furnish the bicyclic lactam (157). Treatment of 157 with 5.0 equivalents of SmI2 in THF in the presence of 2.5 equivalents of water at 0 °C for 3 h, however, afforded the reduction product (158) as the major product in 61% yield as a mixture of diastereoisomers, together with 22% of the recovered starting material (157). On the other hand, a similar treatment of amine (156) with 5.0 equivalents of SmI2 in THF in the presence of 2.5 equivalents of water at 0 °C for 30 min generated two products, which, without separation, were heated at reflux in toluene to give (-)-adalinine (1), in 16% yield from 156, together with the bicyclic alcohol (158) in 38% yield.
In summary, we were able to establish an alternative stereoselective chiral synthesis of (-)-adalinine (1) by employing a SmI2-promoted reductive carbon-nitrogen bond cleavage reaction of a 3-oxopyrrolidine derivative as a key reaction. In this synthesis, we assumed that the formation of samarium-involved chelation intermediate would play an important role for the desired fragmentation. It is noteworthy that water was the best proton source for this reaction, although the reasons remained unclear. This methodology seems to be applicable to various types of 3-oxo-pyrrolidine and -piperidine derivatives.
10. CONCLUSION
The use of samarium diiodide as a mild and selective one-electron transfer agent has become an important tool in organic synthesis. This reagent proved to be an outstanding versatile reagent in stereoselective reactions to synthesize highly functionalized, structurally complex organic compounds inaccessible by established conventional methods. We have already established a regioselective carbon-carbon bond cleavage reaction of γ−halo carbonyl compounds, and its utilization in the synthesis of various types of biologically active natural products. However, a SmI2-promoted reductive carbon-nitrogen bond cleavage reaction has received relatively little attention. In this review article, we would like to discuss a general carbon-nitrogen bond cleavage reaction of α-amino carbonyl compounds newly developed in our laboratory, and utilization of this methodology in the synthesis of a number of bioactive alkaloids, since this reaction proceeds in relatively high yield under mild reaction conditions. Although we did not mention in this manuscript, enantioselective synthesis of (R)-deoxydysibetaine and (-)-4-epi-dysibetaine was also achieved by employing this methodology as a key reaction.87
We hope that this methodology is recognized as one of the versatile synthetic tools, and many organic chemists employ this reaction in the synthesis of useful bioactive compounds including natural products.
ACKNOWLEDGEMENTS
This research was supported financially in part by a grant for the Open Research Center Project of Hoshi University and a Grant-in-Aid from the Ministry of Education, Culture, Sports, Science and Technology of Japan. I would also like to thank the dedicated undergraduate, graduate and postdoctoral student, and research associates in my group who made this work possible. They are listed as co-authors and I sincerely regret any inadvertent omissions.
References
1. P. Girard, J. L. Namy, and H. B. Kagan, J. Am. Chem. Soc., 1980, 102, 2693. CrossRef
2. For recent reviews of SmI2-mediated reaction, see: (a) J. Inanaga, J. Org. Synth. Chem., 1989, 47, 200; (b) J. A. Soderquist, Aldrichim. Acta, 1991, 24, 15; (c) D. P. Curran, T. L. Fevig, C. P. Jasperse, and M. J. Totleben, Synlett, 1992, 943; CrossRef (d) G. A. Molander, Chem. Rev., 1992, 92, 29; CrossRef (e) G. A. Molander and C. R. Harris, Chem. Rev., 1996, 96, 307; CrossRef (f) T. Skrydstrup, Angew. Chem., Int. Ed. Engl., 1997, 36, 345; CrossRef (g) G. A. Molander and C. R. Harris, Tetrahedron, 1998, 54, 3321; CrossRef (h) R. Nomura and T. Endo, Chem. Eur. J., 1998, 4, 1605; CrossRef (i) A. Krief and A.-M. Laval, Chem. Rev., 1999, 99, 745; CrossRef (j) P. G. Steel, J. Chem. Soc., Perkin Trans. 1, 2001, 2727; CrossRef (k) S. Agarwal and A. Greiner, J. Chem. Soc., Perkin Trans. 1, 2002, 2033; CrossRef (l) H. B. Kagan, Tetrahedron, 2003, 59, 10351; CrossRef (m) M. Berndt, S. Gross, A. Hölemann, and H.-U. Reissig, Synlett, 2004, 422; CrossRef (n) D. J. Edmonds, D. Johnston, and D. J. Procter, Chem. Rev., 2004, 104, 3371; CrossRef (o) D. Y. Jung and Y. H. Kim, Synlett, 2005, 3019; CrossRef (p) K. Gopalaiah and H. B. Kagan, New J. Chem., 2008, 32, 607; CrossRef (q) I. M. Rudkin, L. C. Miller, and D. J. Procter, Organomet. Chem., 2008, 34, 19; CrossRef (r) K. C. Nicolaou, S. P. Ellery, and J. S. Chen, Angew. Chem. Int. Ed., 2009, 48, 7140; CrossRef (s) D. J. Procter, R. A. Flowers, II, and T. Skrydstrup, Organic Synthesis Using Samarium Diiodide: A Practical Guide; Royal Society of Chemistry Publishing: UK, 2010; p. 204.
3. (a) T. Honda, S. Yamane, K. Naito, and Y. Suzuki, Heterocycles, 1994, 37, 515; CrossRef (b) T. Honda, F. Ishikawa, and S. Yamane, J. Chem. Soc., Chem. Commun., 1994, 499; CrossRef (c) T. Honda, S. Yamane, K. Naito, and Y. Suzuki, Heterocycles, 1995, 40, 301; CrossRef (d) T. Honda, F. Ishikawa, and S. Yamane, J. Chem. Soc., Perkin Trans. 1, 1996, 1125; CrossRef (e) T. Honda, S. Yamane, F. Ishikawa, and M. Katoh, Tetrahedron, 1996, 37, 12177; CrossRef (f) T. Honda, M. Katoh, and S. Yamane, J. Chem. Soc., Perkin Trans. 1, 1996, 2219; (g) T. Honda and M. Katoh, Chem. Commun., 1997, 369. CrossRef
4. T. Honda and F. Ishikawa, Chem. Commun., 1999, 1065. CrossRef
5. (a) G. A. Molander and P. J. Stengel, J. Org. Chem., 1995, 60, 6660; CrossRef (b) G. A. Molander and P. J. Sangel, Tetrahedron, 1997, 53, 8887; CrossRef (c) N. H. Kawahara and M. Goodman, Tetrahedron Lett., 1999, 40, 2271. CrossRef
6. (a) J. M. Aurrecoechea and A. Fernandez-Acebes, Tetrahedron Lett., 1993, 34, 549; CrossRef (b) J. M. Aurrecoechea and A. Fernandez-Acebes, Synlett, 1996, 39; CrossRef (c) A. R. Katritzky, M. Qi, D. Feng, and D. A. Nichols, J. Org. Chem., 1997, 62, 4121. CrossRef
7. H.-Y. Kang, A. N. Pae, Y. S. Cho, H. Y. Koh, and B. Y. Chung, Chem. Commun., 1997, 821. CrossRef
8. The starting phenylalanine derivatives were prepared from the methyl ester by the known procedures; N-methyl derivative: M. C. Allen, W. Fuhrer, B. Tuck, R. Wade, and J. M. Wood, J. Med. Chem., 1989, 32, 1652; CrossRef N,N-dimethyl derivative: T. Hayashi, M. Konishi, M. Fukushima, K. Kanehira, T. Hioki, and M. Kumada, J. Org. Chem., 1983, 48, 2195; CrossRef N-benzyl derivative: F. Effenberger, U. Burkard, and J. Willfahrt, Liebigs Ann. Chem., 1986, 314; CrossRef N,N-dibenzyl derivative: B. D. Gray and P. W. Jeffs, J. Chem. Soc., Chem. Commun., 1987, 1329; CrossRef N-acetyl derivative: M. J. Burk, J. E. Feaster, E. H. Harlow, and L. Richard, Tetrahedron: Asymmetry, 1991, 2, 569; CrossRef N-Boc derivative: M. Sakaitani and Y. Ohfune, J. Org. Chem., 1990, 55, 870. CrossRef
9. N-Benzylproline methyl ester was prepared from methyl prolinate: V. Ferey, P. Vedrenne, L. Toupet, T. L. Gall, and C. Mioskowski, J. Org. Chem., 1996, 61, 7244; CrossRef isopropyl 4-tert-butyldimethyl- siloxyprolinate was prepared by silylation of the corresponding ester, which on N-benzylation with BnBr and NaH gave the N-benzyl derivative.
10. N-Substituted pipecolinates were prepared from ethyl pipecolinate by alkylation with the suitable alkyl halide and iPr2NEt or by acetylation with Ac2O.
11. 2-Acetyl-N-alkylpiperidine derivatives were prepared from ethyl pipecolinate via four steps involving N-alkylation with alkyl halide, hydrolysis of the ester, conversion of the acid to the Weinreb’s amide (S. Nahm and S. M. Weinreb, Tetrahedron Lett., 1981, 22, 3815), and treatment of the amide with MeMgBr; CrossRef the N-acetyl compound was derived from the N-benzyl derivative by reductive debenzylation over Pd/C in the presence of Ac2O; the N-Boc derivative was prepared according to the known procedure: S. Aoyagi, T.-C. Wang, and C. Kibayashi, J. Am. Chem. Soc., 1993, 115, 11393. CrossRef
12. (a) J. Inanaga, M. Ishikawa, and M. Yamaguchi, Chem. Lett., 1987, 1485; CrossRef (b) E. Hasegawa and D. P. Curran, Tetrahedron Lett., 1993, 34, 1717; CrossRef (c) F. Machrouhi, B. Hamann, J.-L. Namy, and H. B. Kagan, Synlett, 1996, 633; CrossRef (d) M. Shabangi and R. A. Flowers, II, Tetrahedron Lett., 1997, 38, 1137; CrossRef (e) M. Shabangi, J. M. Sealy, J. R. Fuchs, and R. A. Flowers, II, Tetrahedron Lett., 1998, 39, 4429. CrossRef
13. For a recent review of the coccinellied alkaloids, see: A. G. King and J. Meinwald, Chem. Rev., 1996, 96, 1105. CrossRef
14. T. Honda and M. Kimura, Org. Lett., 2000, 2, 3925. CrossRef
15. G. Lognay, J. L. Hemptinne, F. Y. Chan, CH. Gasper, M. Marlier, J. C. Braekman, D. Daloze, and J. M. Pasteels, J. Nat. Prod., 1996, 59, 510. CrossRef
16. N. Yamazaki, T. Ito, and C. Kibayashi, Tetrahedron Lett., 1999, 40, 739. CrossRef
17. (a) F. Broeders, J. C. Braekman, and D. Daloze, D. Bull. Soc. Chim. Belg., 1997, 106, 377; (b) N. Yamazaki, T. Ito, and C. Kibayashi, Synlett, 1999, 37; CrossRef (c) D. J. Wardrop, C. L. Landrie, and J. A. Ortiz, Synlett, 2003, 1352. CrossRef
18. J. S. Peterson, G. Fels, and H. Rapoport, J. Am. Chem. Soc., 1984, 106, 4539. CrossRef
19. M. Roth, P. Dubs, E. Götschi, and A. Eschenmoser, Helv. Chim. Acta, 1971, 54, 710. CrossRef
20. For Michael addition of Grignard reagent to enaminones, see: D. L. Comins, D. H. LaMunyon, and X. Chen, J. Org. Chem., 1997, 62, 8182. CrossRef
21. A small amount of uncyclized δ-amino ester could also be isolated from this reaction; however, the uncyclized compound was easily transformed to the cyclization product by heating in benzene or by standing at room temperature for few days. The yield was demonstrated as the combined yield.
22. (a) M. Katoh, R. Matsyne, H. Nagase, and T. Honda, Tetrahedron Lett., 2004, 45, 6221; CrossRef (b) M. Katoh, R. Matsune, and T. Honda, Heterocycles, 2006, 67, 189. CrossRef
23. (a) J. B. Koepfli, J. F. Mead, and J. A. Brockman, Jr., J. Am. Chem. Soc., 1947, 69, 1837; CrossRef (b) J. B. Koepfli, J. F. Mead, and J. A. Brockman, Jr., J. Am. Chem. Soc., 1949, 71, 1048; CrossRef (c) K. Murata, F. Takeno, S. Fushiya, and Y. Oshima, J. Nat. Prod., 1998, 61, 729. CrossRef
24. (a) C. S. Jang, F. Y. Fu, C. Y. Wang, K. C. Huang, G. Lu, and T. C. Thou, Science, 1946, 103, 59; CrossRef (b) T.-Q. Chou, F. Y. Fu, and Y. S. Kao, J. Am. Chem. Soc., 1948, 70, 176; CrossRef (c) A. K. Frederick, Jr., C. F. Spencer, and K. Folkers, J. Am. Chem. Soc., 1948, 70, 2091; CrossRef (d) Y. Takaya, H. Tasaka, T. Chiba, K. Uwai, M. Tanitsu, H.-S. Kim, Y. Wataya, M. Miura, M. Takeshita, and Y. Oshima, J. Med. Chem., 1999, 42, 3163; CrossRef (e) H. Kikuchi, H. Tasaka, S. Hirai, Y. Takaya, Y. Iwabuchi, H. Ooi, S. Hatakeyama, H.-S. Kim, Y. Wataya, and Y. Oshima, J. Med. Chem., 2002, 45, 2563; CrossRef (f) S. Hirai, H. Kikuchi, H.-S. Kim, K. Begum, Y. Wataya, H. Tasaka, Y. Miyazawa, K. Yamamoto, and Y. Oshima, J. Med. Chem., 2003, 46, 4351. CrossRef
25. S. Kobayashi, M. Ueno, R. Suzuki, and H. Ishitani, Tetrahedron Lett., 1999, 40, 2175. CrossRef
26. For recent syntheses of febrifugine and/or isofebrifugine, see: (a) L. E. Burgess, E. K. M. Gross, and J. Jurka, Tetrahedron Lett., 1996, 37, 3255; CrossRef (b) S. Kobayashi, M. Ueno, R. Suzuki, H. Ishitani, H.-S. Kim, and Y. Wataya, J. Org. Chem., 1999, 64, 6833; CrossRef (c) Y. Takeuchi, H. Abe, and T. Harayama, Chem. Pharm. Bull., 1999, 47, 905; (d) Y. Takeuchi, M. Hattori, H. Abe, and T. Harayama, Synthesis, 1999, 1814; CrossRef (e) Y. Takeuchi, K. Azuma, M. Takakura, H. Abe, and T. Harayama, Chem. Commun., 2000, 1643; CrossRef (f) O. Okitsu, R. Suzuki, and S. Kobayashi, Synlett, 2000, 989; CrossRef (g) T. Taniguchi and K. Ogasawara, Org. Lett., 2000, 2, 3193; CrossRef (h) Y. Takeuchi, K. Azuma, K. Takakura, H. Abe, H.-S. Kim, Y. Wataya, and T. Harayama, Tetrahedron, 2001, 57, 1213; CrossRef (i) H. Ooi, A. Urushibara, T. Esumi, Y. Iwabuchi, and S. Hatakeyama, Org. Lett., 2001, 3, 953; CrossRef (j) M. Sugiura and S. Kobayashi, Org. Lett., 2001, 3, 477; CrossRef (k) M. Sugiura, H. Hagio, R. Hirabayashi, and S. Kobayashi, J. Org. Chem., 2001, 66, 809; CrossRef (l) M. Sugiura, H. Hagio, R. Hirabayashi, and S. Kobayashi, J. Am. Chem. Soc., 2001, 123, 12510; CrossRef (m) P.-Q. Huang, B.-G. Wei, and Y.-P. Ruan, Synlett, 2003, 1663. CrossRef
27. X. Zhang, A. C. Schmitt, and W. Jiang, Tetrahedron Lett., 2001, 42, 5335. CrossRef
28. C. Nájera and M. Yus, Tetrahedron: Asymmetry, 1999, 10, 2245 and references cited therein. CrossRef
29. (a) I. Collado, J. Ezquerra, J. J. Vaquero, and C. Pedregal, doi:10.1016/0040-4039(94)80043-X, 1994, 35, 8037; CrossRef (b) J. Mulzer, F. Schülzchen, and J.-W. Bats, Tetrahedron, 2000, 56, 4289. CrossRef
30. F. N. Tebbe, G. W. Parshall, and G. S. Reddy, J. Am. Chem. Soc., 1978, 100, 3611. CrossRef
31. (a) M. Katoh, H. Mizutani, and T. Honda, Tetrahedron Lett., 2005, 46, 5161; CrossRef (b) M. Katoh, H. Mizutani, and T. Honda, Heterocycles, 2006, 69, 193. CrossRef
32. For recent reviews of synthesis and biological activity, see: (a) A. E. Nemr, Tetrahedron, 2000, 56, 8579; CrossRef (b) N. Asano, R. J. Nash, R. J. Molyneux, and G. W. J. Fleet, Tetrahedron: Asymmetry, 2000, 11, 1645; CrossRef (c) P. Sears and C.-H. Wong, Angew. Chem., Int. Ed., 1999, 38, 2300; CrossRef (d) J. P. Michael, Nat. Prod. Rep., 1999, 16, 675; CrossRef (e) J. P. Michael, Nat. Prod. Rep., 2000, 17, 579; CrossRef (f) J. P. Michael, Nat. Prod. Rep., 2001, 18, 520; CrossRef (g) J. P. Michael, Nat. Prod. Rep., 2002, 19, 719; CrossRef (h) J. P. Michael, Nat. Prod. Rep., 2003, 20, 458; CrossRef (i) J. P. Michael, Nat. Prod. Rep., 2004, 21, 625; CrossRef (j) J. P. Michael, Nat. Prod. Rep., 2005, 22, 603; CrossRef (k) A. D. Elbein and R. J. Molyneux, In Iminosugars as Glycosidase Inhibitors, ed. by A. E. Stutz, Wiley-VCH: Weinheim, Germany, 1999; p. 216; (l) H. Takahata and T. Momose, In The Alkaloids, ed. by G. A. Cordell, Academic: San Diego, CA, 1993; Vol. 44, Chapter 3; (m) A. D. Elbein and R. Molyneux, In Alkaloids: Chemical and Biological Perspectives; ed. by S. W. Pelletier, Wiley and Sons: New York, 1987; Vol. 5; (n) N. Toyooka and H. Nemoto, Trends in Heterocyclic Chemistry, 2002, 8, 145.
33. S. C. Nigam, A. Mann, M. Taddei, and C. G. Wermuth, Synth. Commun., 1989, 19, 3139. CrossRef
34. G. Bartoli, M. Bosco, M. Locatelli, E. Marcantoni, P. Melchiorre, and L. Sambri, Org. Lett., 2005, 7, 427. CrossRef
35. (a) R. Appel, Angew. Chem., Int. Ed. Engl., 1975, 14, 801; CrossRef (b) Y. Shishido and C. Kibayashi, J. Org. Chem., 1992, 57, 2876. CrossRef
36. H. K. Lee, J. S. Chun, and S. P. Chwang, J. Org. Chem., 2003, 68, 2471. CrossRef
37. For reviews on catalytic metathesis, see: (a) R. H. Grubbs, S. J. Miller, and G. C. Fu, Acc. Chem. Res., 1995, 28, 446; CrossRef (b) H.-G. Schrnalz, Angew. Chem., Int. Ed. Engl., 1995, 34, 1833; CrossRef (c) M. Schuster and S. Blechert, Angew. Chem., Int. Ed. Engl., 1997, 36, 2036; CrossRef (d) S. K. Armstrong, J. Chem. Soc., Perkin Trans. 1, 1998, 371; CrossRef (e) R. H. Grubbs and S. Chang, Tetrahedron, 1998, 54, 4413; CrossRef (f) R. R. Schlock, Tetrahedron, 1999, 55, 8141; CrossRef (g) A. J. Phillips and A. D. Abell, Aldrichim. Acta, 1999, 32, 75; (h) A. Fürstner, Angew. Chem. Int. Ed., 2000, 39, 3012; CrossRef (i) T. M. Trnka and R. H. Grubbs, Acc. Chem. Res., 2001, 34, 18; CrossRef (j) A. H. Hoveyda and R. R. Schrock, Chem. Eur. J., 2001, 7, 945; CrossRef (k) R. R. Schrock and A. H. Hoveyda, Angew. Chem. Int. Ed., 2003, 42, 4592. CrossRef
38. M. G. M. D’Oca, R. A. Pilli, and I. Vencato, Tetrahedron Lett., 2000, 41, 9709. CrossRef
39. M. Miyazawa, K. Yoshio, Y. Ishikawa, and H. Kameoka, J. Agric. Food Chem., 1998, 46, 1059. CrossRef
40. L. Zhang, Y. Huang, Y. Qian, and P. Xiao, Zhongguo Yixue Kexueyuan Xuebao, 1995, 17, 343.
41. Y. Suzuki, K. Taguchi, Y. Hagiwara, K. Kajiyama, and T. Ikeda, Jpn. J. Pharmacol., 1981, 31, 653. CrossRef
42. H. Matsuda, T. Morikawa, M.; Oda, Y. Asao, and M. Yoshikawa, Bioorg. Med. Chem. Lett., 2003, 13, 4445. CrossRef
43. (a) S. Yasuda, M. Hanaoka, and Y. Arata, Chem. Pharm. Bull., 1980, 28, 831; (b) Y. C. Hwang and F. W. Fowler, J. Org. Chem., 1985, 50, 2719; CrossRef (c) Y. C. Hwang, M. Chu, and F. W. Fowler, J. Org. Chem., 1985, 50, 3885. CrossRef
44. (a) W. J. Moran, K. M. Goodenough, P. Raubo, and J. P. A. Harrity, Org. Lett., 2003, 5, 3427; CrossRef (b) K. M. Goodenough, W. J. Moran, P. Raubo, and J. P. A. Harrity, J. Org. Chem., 2005, 70, 207. CrossRef
45. (a) H. Bredereck, G. Simchen, S. Rebsdat, W. Kantlehner, P. Horn, R. Wahl, H. Hoffman, and P. Grieshaber, Chem. Ber., 1968, 101, 41; CrossRef (b) S. Danishefsky, E. Berman, L. A. Clizbe, and M. Hirama, J. Am. Chem. Soc., 1979, 101, 4385. CrossRef
46. (a) J.-M. Nuzilland, A. Boumendjel, and G. Massiot, Tetrahedron Lett., 1989, 30, 3779; CrossRef (b) D. F. Netz and J. L. Seidel, Tetrahedron Lett., 1992, 33, 1957. CrossRef
47. T. Honda, R. Takahashi, and H. Namiki, J. Org. Chem., 2005, 70, 499. CrossRef
48. (a) S. Ohmiya, K. Saito, and I. Murakoshi, Lupine Alkaloids. In The Alkaloids; ed. by G. A. Cordell, Academic Press: New York, 1995; Vol. 47, p. 1; (b) A. El-Shazly, T. Sarg, A. Ateya, E. A. Witte, and M. Wink, Pharmazie, 1996, 51, 768.
49. (a) A. Partheil, Arch. Pharm. (Weinheim), 1894, 232, 161; (b) H. R. Ing, J. Chem. Soc., 1932, 2778; CrossRef (c) N. J. Leonard, In The Alkaloids; ed. by R. H. F. Manske and H. L. Holmes, Academic Press: New York, 1953; Vol. 3, pp. 119-199.
50. (a) E. E. van Tamelen and J. S. Baran, J. Am. Chem. Soc., 1955, 77, 4944; CrossRef (b) E. E. van Tamelen and J. S. Baran, J. Am. Chem. Soc., 1956, 78, 2913; CrossRef (c) E. E. van Tamelen and J. S. Baran, J. Am. Chem. Soc., 1958, 80, 4659; CrossRef (d) F. Bohlmann, A. English, N. Ottawa, H. Sander, and W. Weise, Chem. Ber., 1956, 89, 792; CrossRef (e) T. R. Govindachari, S. Rajadurai, M. Subramanian, and B. S. Thyagarajan, J. Chem. Soc., 1957, 3839. CrossRef
51. For recent synthesis of racemic cytisine, see: (a) P. Nshimyumukiza, D. Cahard, J. Rouden, M.-C. Lasne, and J.-C. Plaquevent, Tetrahedron Lett., 2001, 42, 7787; CrossRef (b) B. T. O’Neill, D. Yohannes, M. W. Bundesmann, and E. P. Arnold, Org. Lett., 2000, 2, 4201; CrossRef (c) J. W. Coe, Org. Lett., 2000, 2, 4205; CrossRef (d) C. Botuha, C. M. S. Galley, and T. Gallagher, Org. Biomol. Chem., 2004, 2, 1825 and references therein; CrossRef For chiral synthesis of (-)-cytisine, see: (e) B. Danieli, G. Lesma, D. Passarella, A. Sacchetti, A. Silvani, and A. Virdis, Org. Lett., 2004, 6, 493. CrossRef
52. (a) R. B. Barlow and L. J. McLeod, Br. J. Pharmacol., 1969, 35, 161; (b) I. A. McDonald, N. Cosford, and J.-M. Vernier, Annual Reports in Medicinal Chemistry; Academic Press: New York, 1994; Vol. 30, p. 41; (c) J. D. Schmitt and M. Bencherif, Annual Reports in Medicinal Chemistry; Academic Press: New York, 2000; Vol. 35, p. 41.
53. (a) L. A. Pabreza, S. Dhawan, and K. J. Keller, Mol. Pharmacol., 1991, 39, 9; (b) M. Hall, L. Zerbe, S. Leonard, and R. Freedman, Brain Res., 1993, 600, 127; CrossRef (c) H. K. Happe, J. L. Peters, D. A. Bergman, and L. C. Murrin, Neuroscience, 1994, 62, 929. CrossRef
54. I. Murakoshi, E. Kidoguchi, J. Haginiwa, S. Ohmiya, K. Higashiyama, and H. Otomasu, Phytochemistry, 1981, 20, 1407. Kuraramine was isolated as an amorphous solid. CrossRef
55. P. Máximo and A. Lourencüo, J. Nat. Prod., 2000, 63, 201. CrossRef
56. I. Murakoshi, E. Kidoguchi, J. Haginiwa, S. Ohmiya, K. Higashiyama, and H. Otomasu, Phytochemistry, 1982, 21, 2379. The optical rotation has not been reported, due to the small amount available. CrossRef
57. A. M. P. Koskinen, J. Schwerdtfeger, and M. Edmonds, Tetrahedron Lett., 1997, 38, 5399. CrossRef
58. (a) C. González, E. Guilián, and L. Castedo, L. Tetrahedron, 1999, 55, 5195; CrossRef (b) M. Adamczyk, S. R. Akireddy, and R. E. Reddy, Org. Lett., 2000, 2, 3421; CrossRef (c) J. E. Baldwin, R. M. Adlington, A. Conte, N. R. Irtapati, R. Marquez, and G. J. Pritchard, Org. Lett., 2002, 4, 2125. CrossRef
59. Although the value of the optical rotation of ent-87 was slightly lower than that in the literature, we believe that our synthetic compound is optically pure, since the intermediate 96 was successfully transformed into cytisine with the correct optical rotation.
60. Y.-H. Wang, J.-S. Li, H. Kubo, K. Higashiyama, H. Komiya, and S. Ohmiya, Chem. Pharm. Bull., 1999, 47, 1308.
61. M. Normatov, K. A. Abduazimov, and S. Y. Yunusov, Dokl. Akad. Nauk UzSSR, 1962, 19, 45.
62. (a) M. Katoh, H. Inoue, A. Suzuki, and T. Honda, Synlett, 2005, 2820; CrossRef (b) M. Katoh, H. Inoue, and T. Honda, Heterocycles, 2007, 72, 497. CrossRef
63. K. Hayashi, K. Nunami, K. Sakai, Y. Ozaki, J. Kato, K. Kinashi, and N. Yoneda, Chem. Pharm. Bull., 1983, 31, 3553.
64. N. Gulavita, A. Hori, Y. Shimizu, P. Laszlo, and J. Clardy, Tetrahedron Lett., 1988, 29, 4381. CrossRef
65. T. Honda, A. Yamamoto, Y. Cui, and M. Tsubuki, J. Chem. Soc., Perkin Trans. 1, 1992, 531. CrossRef
66. K. O. Hallinan and T. Honda, Tetrahedron, 1995, 51, 12211. CrossRef
67. Synthesis of (-)- and racemic aphanorphine: (a) S. Takano, K. Inomata, T. Sato, M. Takahashi, and K. Ogasawara, J. Chem. Soc., Chem. Commun., 1990, 290; CrossRef (b) A. N. Hulme, S. S. Henry, and A. I. Meyers, J. Org. Chem., 1995, 60, 1265; CrossRef (c) A. I. Meyers, W. Schmidt, and B. Santiango, Heterocycles, 1995, 40, 525; CrossRef (d) A. Fadal and O. Arzel, Tetrahedron:Asymmetry, 1995, 6, 893; CrossRef (e) M. Node, H. Imazato, R. Kurosaki, Y. Kawano, T. Inoue, K. Nishide, and K. Fuji, Heterocycles, 1996, 42, 811; CrossRef (f) S. Shiotani, H. Okada, K. Nakamata, T. Yamamoto, and F. Sekino, Heterocycles, 1996, 43, 1031; CrossRef (g) A. Fadal and O. Arzel, Tetrahedron:Asymmetry, 1997, 8, 371; CrossRef (h) M. Shimizu, T. Kamikubo, and K. Ogasawara, Heterocycles, 1997, 46, 21; CrossRef (i) K. Tanaka, T. Taniguchi, and K. Ogasawara, Tetrahedron Lett., 2001, 42, 1049; CrossRef (j) O. Tamura, T. Yanagimachi, T. Kobayashi, and H. Ishibashi, Org. Lett., 2001, 3, 2427; CrossRef (k) A. S. ElAzab, T. Taniguchi, and K. Ogasawara, Heterocycles, 2002, 56, 39; CrossRef (l) S. K. Taylor, M. Ivanovic, L. J. Simons, and M. M. Davis, Tetrahedron:Asymmetry, 2003, 14, 743; CrossRef (m) Y. Kita, J. Futamura, Y. Ohba, Y. Sawama, J. K. Genesh, and H. Fujioka, J. Org. Chem., 2003, 68, 8268; CrossRef (n) O. Tamura, T. Yanagimachi, and H. Ishibashi, Tetrahedron:Asymmetry, 2003, 14, 3033; CrossRef (o) T. Yoshimitsu, C. Atsumi, E. Iimori, H. Nagaoka, and T. Tanaka, Tetrahedron Lett., 2008, 49, 4473. CrossRef
68. J. R. Fuchs and R. L. Funk, Org. Lett., 2001, 3, 3923. CrossRef
69. J. Jurczak, D. Gryko, E. Kobrzycka, H. Gruza, and P. Prokopowicz, Tetrahedron, 1998, 54, 6051. CrossRef
70. (a) A. R. Katritzky, X. Lan, J. Z. Yang, and O. V. Denisko, Chem. Rev., 1998, 98, 409; CrossRef (b) A. R. Katritzky, J. Cobo-Domingo, B. Yang, and P. J. Steel, Tetrahedron:Asymmetry, 1999, 10, 255; CrossRef (c) O. Koepler, S. Laschat, A. Baro, P. Fischer, B. Miehlich, M. Horfilder, and C. le Viseur, Eur. J. Org. Chem., 2004, 3611. CrossRef
71. S. Takano, K. Inomata, T. Sato, and K. Ogasawara, J. Chem. Soc., Chem. Commun., 1989, 1591. CrossRef
72. T. Honda, E. Aranishi, and K. Kaneda, Org. Lett., 2009, 11, 1857. CrossRef
73. (a) G. Blasko, In The Alkaloids; ed. by A. Brossi, Academic Press Inc.: New York, 1990; p. 157; (b) T. B. Lee, Illustrated Flora of Korea; Hanygmoonsa: Seoul, 1989; p. 385.
74. J. Fidalgo, L. Castedo, and D. Dominguez, Tetrahedron Lett., 1993, 34, 7317. CrossRef
75. (a) G. Blasko, S. F. Hussain, A. J. Freyer, and M. Shamma, Tetrahedron Lett., 1981, 22, 3127; CrossRef (b) N. Murugesan, G. Blasko, R. D. Minard, and M. Shamma, Tetrahedron Lett., 1981, 22, 3131; CrossRef (c) G. Blasko, N. Murugesan, S. F. Hussain, R. D. Minard, M. Shamma, B. Sener, and M. Tanker, Tetrahedron Lett., 1981, 22, 3135; CrossRef (d) G. Blasko, N. Murugesan, A. J. Freyer, D. J. Gula, B. Sener, and M. Shamma, Tetrahedron Lett., 1981, 22, 3139; CrossRef (e) G. Blasko, N. Murugesan, A. J. Freyer, R. D. Minard, and M. Shamma, Tetrahedron Lett., 1981, 22, 3143. CrossRef
76. G. I. Yakimov, N. M. Mollov, J. E. Leet, H. Guinaudeau, A. J. Freyer, and M. Shamma, J. Nat. Prod., 1984, 47, 1048. CrossRef
77. G. Blasko, Acta Chim. Hung., 1991, 128, 819.
78. For transformation to protopine alkaloid, see: (a) K. Orito and M. Itoh, J. Chem. Soc., Chem. Commun., 1978, 812; CrossRef (b) K. Orito, Y. Kurokawa, and M. Itoh, Tetrahedron, 1980, 36, 617; CrossRef (c) Y. Wada, H. Kaga, S. Uchiito, E. Kumazawa, M. Tomiki, Y. Onozaki, N. Kurono, M. Tokuda, T. Ohkuma, and K. Orito, J. Org. Chem., 2007, 72, 7301; CrossRef For transformation to rhoeadine alkaloid, see: (d) K. Orito, R. H. Manske, and R. Rodrigo, J. Am. Chem. Soc., 1974, 96, 1944; CrossRef (e) M. Hanaoka, M. Inoue, N. Kobayashi, and S. Yasuda, Chem. Pharm. Bull., 1987, 35, 980.
79. M. W. Giese and W. H. Moser, J. Org. Chem., 2005, 70, 6222. CrossRef
80. For photochemical synthesis of benzindenoazepine ring system, see: (a) J. Fidalgo, L. Castedo, and D. Dominguez, Heterocycles, 1994, 39, 581. For a radical cyclization approach, see ref 2; CrossRef For transformation of protoberberines to benzindenoazepines, see: (b) M. Hanaoka, M. Iwasaki, S. Sakurai, and C. Mukai, Tetrahedron Lett., 1983, 24, 3845; CrossRef (c) M. Hanaoka, A. Ashimori, H. Yamagishi, and S. Yasuda, Chem. Pharm. Bull., 1983, 31, 2172; (d) M. Hanaoka, S. K. Kim, M. Inoue, K. Nagami, Y. Shimada, and S. Yasuda, Chem. Pharm. Bull., 1985, 33, 1434.
81. I. Matsuo, T. Takahashi, and S. Oki, Yakugaku Zasshi, 1964, 84, 711.
82. The deethoxycarbonyl analogue of 128 was generated as a byproduct under these reaction conditions.
83. L. A. Mitscher, D. L. Flynn, H. E. Gracey, and S. D. Drake, J. Med. Chem., 1979, 22, 1354. CrossRef
84. (a) T. Honda and C. Hisa, Tetrahedron Lett., 2009, 50, 4654; CrossRef (b) T. Honda and C. Hisa, Heterocycles, 2010, 80, 1381. CrossRef
85. (a) E. Hasegawa, K. Ishiyama, T. Fujita, T. Kato, and T. Abe, J. Org. Chem., 1997, 62, 2396; CrossRef (b) G. E. Keck, C. A. Wager, T. Sell, and T. T. Wager, J. Org. Chem., 1999, 64, 2172; CrossRef (c) A. Dahlën and G. Hilmersson, Chem. Eur. J., 2003, 9, 1123; CrossRef (d) P. R. Chopade, E. Prasad, and R. A. Flowers, J. Am. Chem. Soc., 2004, 126, 44; CrossRef (e) H. Farran and S. Shmaryahu Hoz, J. Org. Chem., 2009, 74, 2075; CrossRef (f) T. Ankner and G. Hilmersson, Org. Lett., 2009, 11, 1865. CrossRef
86. B. S. Bal, W. E. Childers, Jr., and H. W. Pinnick, Tetrahedron, 1981, 37, 2091. CrossRef
87. M. Katoh, C. Hisa, and T. Honda, Tetrahedron Lett., 2007, 48, 4691. CrossRef