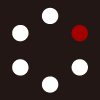
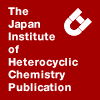
HETEROCYCLES
An International Journal for Reviews and Communications in Heterocyclic ChemistryWeb Edition ISSN: 1881-0942
Published online by The Japan Institute of Heterocyclic Chemistry
e-Journal
Full Text HTML
Received, 15th December, 2010, Accepted, 25th January, 2011, Published online, 2nd February, 2011.
DOI: 10.3987/REV-10-689
■ Sphingolipids and Glycosphingolipids — Their Synthesis and Bioactivities
Kenji Mori* and Takuya Tashiro
Glycosphinogolipid Synthesis Group, Laboratory for Immune Regulation, RIKEN Research Center for Allergy and Immunology, Wako-shi, Saitama 351-0198, Japan
Abstract
Chemistry of sphingolipids and glycosphingolipids is discussed with main emphasis on their synthetic methods. Microbial, plant, animal and marine sphingolipids and glycosphingolipids with the following biological roles are treated in this review: (i) inducers of fruiting body formation in Basidiomycetes, (ii) water barrier and supporting material of animal epidermis, and (iii) bioregulators of medicinal interests including immunostimulants.1. INTRODUCTION
1-1. Structure of glycosphingolipids
Glycosphingolipids were discovered by Thudichum (1829-1901) as components of the alcohol extract of human brains. He studied chemistry under Liebig in Giessen, became a medical doctor, went to London in 1853, and spent there the rest of his life. He worked on the chemical analysis of human brain between 1865 and 1882, and published a book in 1884 entitled "A Treatise on the Chemical Constitution of the Brain based throughout upon Original Researches." He isolated from brain an organic base in addition to sugars and fatty acids. Since the structure of the base could not be clarified, Thudichum gave it the name "sphingosine." Its structure was thought to be as mysterious as Sphinx. In mid 20th century, the structures of glycosphingolipids were clarified by the efforts of Carter, Klenk, Kuhn, Yamakawa, and others.
The typical structure of a glycosphingolipid is shown in Figure 1. Sphingosine is a serine-derived (2S,3R)-2-amino-1,3-diol with a long alkyl chain. Acylation of its amino group with a fatty acid such as palmitic acid gives ceramide, and glycosylation of the primary hydroxy group of ceramide with D-glucose or D-galactose gives cerebroside <cf. cerebrium (L.) = brain>. These two names, ceramide and cerebroside, were also given by Thudichum. Lipids containing sphingosine are called sphingolipids. Glycosphingolipids are glycosylated sphingolipids.
There are a number of reviews on sphingolipids. Pathobiochemistry of sphingolipids was reviewed by Kolter and Sandhoff.1 Kolter summarized bioorganic chemistry of ceramide.2 Bioorganic chemistry and synthesis of cerebrosides were reviewed by Tan and Chen.3 Liao et al. summarized chemistry and biology of sphingolipids.4
1-2. Biological functions of sphingolipids and glycosphingolipids
Glycosphingolipids are ubiquitous components of eukaryotic cell membranes. They are involved in many aspects of cell signaling like cell-ligand interaction, cell-cell interaction, and cell-pathogen interaction. It is impossible to treat all the aspects. We will treat only the following three roles of sphingolipids and glycosphingolipids: (i) induction of fruiting body formation in Basidyomycete, (ii) functions as water barrier and supporting material of human epidermis, and (iii) biological activities of medicinal interests including immunostimulant activities. Figure 2 shows the representative sphingolipids discussed in the present review.
1-3. Synthesis of glycosphingolipids
A cerebroside can be synthesized by coupling a ceramide with sugars, while a ceramide can be prepared by acylating sphingosine with a fatty acid.
Since various carbohydrates and fatty acids are available as natural products, the synthesis of the sphingosine part and the method for coupling the three moieties deserve critical discussion.
(1) Synthesis of the hydrophilic 2-amino-1,3-diol moiety
The chiral and hydrophilic 2-amino-1,3-diol moiety of sphingolipids is usually prepared from naturally occurring chiral building blocks such as (S)-serine, (2S,3S)-tartaric acid and carbohydrates as shown in Figure 3. The first synthesis of D-erythro-sphingosine was achieved by Newman in 1973,5 employing aldehyde 1 derived from (S)-serine as the starting material. (S)-Serine-derived aldehyde 2 was then employed by Tkaczuk and Thornton.6 These aldehydes 1 and 2 are rather unstable, and readily epimerize at their chiral centers. Garner improved the situation by preparing stereochemically stable 1,1-dimethylethyl 4-formyl-2,2-dimethyloxazolidine-3-carboxylate (3),7 which is now called Garner's aldehyde, and frequently used in sphingolipid synthesis.8-10 An excellent review on Garner's aldehyde is available, covering the period of 1984 to 2000.11
(2S,3S)-Tartaric acid was converted to epoxide 4, which served as a starting material in sphingosine synthesis.12 Carbohydrates were employed by many groups as starting materials: D-mannose by Schlosser,13 D-glucose by Ogawa,14 D-galactose by Schmidt,15 and D-xylose by Kocienski.16
Asymmetric reactions such as Sharpless epoxidation, dihydroxylation, and aminohydroxylation are employed to construct the 2-amino-1,3-diol moiety. Scheme 1 shows an example based on asymmetric epoxidation.17 Allylic alcohol 5 was epoxidized to give 6, which was converted to 7, a building block equivalent to 4 (Figure 3). D-erythro-Sphingosine was synthesized from 7 via 8. Very recently, Kumar et al. synthesized unnatural (2R,3S)-sphingosine and sphinganine together with (2R,3S,4R)- and (2R,3S,4S)-C18-phytosphingosine by utilizing tethered aminohydroxylation reaction.18
(2) Construction of the whole carbon chain
The existing syntheses of D-erythro-sphingosine are rather lengthy as exemplified in Scheme 1. Recent commercial production of D-ribo-phytosphingosine by Degussa using a mutant strain of yeast Pichia ciferii was a welcome news for those engaged in sphingosine synthesis.19 The mutant P. ciferii produces 0.20 g of tertaacetylphytosphingosine per 1 g dry weight. Choi and co-workers further studied the microbial production of phytosphingosine.20 D-ribo-Phytosphingosine is a useful starting material for glycosphingolipids, and was converted to D-erythro-sphingosine via cyclic sulfate 9 by Kim et al. as shown in Scheme 2.21 The conversion was accomplished in fewer steps than in the case of de novo synthesis of sphingosine from (S)-serine.
Since 2004, olefin cross metathesis reaction was applied to the synthesis of glycosphingolipids as illustrated in Schemes 3 and 4. Grubbs's second generation catalyst was found to be suitable for this purpose. Cross metathesis enabled simple syntheses of sphinglipids with a chain-length different from that of naturally occurring materials. Somfai,22 Basu,23 Han24 and co-workers published their successful metathesis in 2004. As can be seen in the case of Basu,23 a bulky substituent at C-3 favors the selective formation of the (E)-double bond at C-4. Basu demonstrated in 2005 that the metathesis could be applicable to a sugar-containing alkene and even to a cerebroside-type alkene.25
Results of the groups of Dhavale,26 Lombardo,27 Katsumura,28 and Castillón29 were also summarized in Scheme 4. A drawback about these metathesis approaches is the high cost of the patent-protected Grubbs's catalyst.
Preparation of sphingosines is reviewed by Howell.30
(3) Glycosylation of sphingosines and ceramides
This subject was recently reviewed by Castillón and his co-workers.31 Examples of glycosylation will be shown in the following sections.
2. CEREBROSIDES THAT INDUCE FRUITING BODY FORMATION IN Basidiomycetes
Fruiting body formation in Basidiomycetes is indeed a spectacular phenomenon especially to those who love to taste mushrooms. In every country, mushrooms are highly appreciated by gourmands. The mechanism of fruiting body formation, however, is still a mystery in spite of the intensive efforts to clarify it.
2-1. Synthesis of Sch II
In 1982 Kawai and Ikeda found that the fruiting body formation of Schizophyllum commune (Japanese name: suéhiro také) can be stimulated by some cerebrosides in its mycelia. They then identified one of the active cerebrosides as (4E,8E,2S,3R,2'R)-N-2'-hydroxyhexadecanoyl-1-O-β-D-glucopyranosyl-9-methyl-4,8-sphingadienine (10, Scheme 5), which had been isolated previously from a sea anemone (Metridium senile) by Karlsson et al. Only 0.1 μg of 10 induces the formation of the fruiting body of S. commune. A trivial name Sch II was given to 10. We became interested in synthesizing 10 because of its remarkable bioactivity and also to develop synthetic methods for sphingolipids.32-34
Cerebrosides are glycosides of ceramides. Accordingly, ceramides must be prepared prior to the synthesis of cerebrosides by glycosidation. Our synthetic plan for 10 is shown in Scheme 5. Sch II (10) can be constructed by connecting α-hydroxy acid B, sphingadienine C and D-glucose D. The acid B can be prepared from α-amino acid E, and C is to be prepared from F and an unstable aldehyde G, which can be synthesized from L-serine (H).
Scheme 6 shows the synthesis of α-hydroxy acid D. 1-Bromotetradecane (A) was converted to (±)-2-chloroacetylaminohexadecanoic acid (B), which was treated with amino acylase of Aspergillus origin to effect asymmetric hydrolysis. This enzyme is known to hydrolyze (S)-B to give (S)-2-aminohexadecanoic acid, while (R)-C remains intact. Acid hydrolysis of (R)-C was followed by deamination with nitrous acid to give (R)-D with retention of configuration. The corresponding α-acetoxy acid was converted to the activated ester E, which served as the acyl donor to the sphingadienine part.
The synthesis of the sphingadienine part and the coupling of the three building blocks to give Sch II (10) are summarized in Scheme 7. Cyclopropyl methyl ketone (A) was converted to diacetate B, which was treated with octylmagnesium bromide under the Schlosser conditions to give C after deacetylation. Further chain-elongation of C gave acetylene D, which served as the hydrophobic part of sphingadienine. As to the preparation of the polar and hydrophilic part, its synthesis started from (S)-serine, which was converted to the unstable aldehyde F (= 2). Reaction of F with alkenylalane E prepared from D yielded G as crystals after chromatographic purification to remove H. Subsequently, G was converted to Sch II (10) as depicted.34 Synthetic Sch II (10) showed spectral and biological properties identical to those of the natural product. The structure of Sch II was thus confirmed as 10.34 Recently in 2010, a new synthesis of Sch II was published by Black and Kocienski.16
Ceramide (without D-glucose) corresponding to 10 was also bioactive, while the diastereomeric
ceramide derived from H was only 1/7.5 as active as the ceramide corresponding to 10. Stereochemistry at C-3 of the sphingadienine part was important for bioactivity.35
2-2. Synthesis of Pen III, Pen II and Whe II
After completion of the synthesis of Sch II (10) in 1985, we continued our studies on the cerebrosides inducing the fruiting body formation in Schizophyllum commune, and the cerebrosides shown in Schemes 8-10 were synthesized. Pen III (11) is a metabolite of Penicillium funiculosum isolated as a fruiting inducer against Schizophyllum commune. Its synthesis was carried out as shown in Scheme 8 employing Garner's aldehyde (D = 3) as a starting material.36 The aldehyde D is not so unstable, and does not racemize easily. For the preparation of (R)-hydroxy acid C, (±)-C was subjected to asymmetric acetylation with vinyl acetate in the presence of lipase PS.36
Pen II (12) is another metabolite of P. fumiculosum, and also induces fruiting body formation of S. commune. For the synthesis of the sphingadienine part C of 12, a new and simpler route was adopted as shown in Scheme 9.37 Garner's aldehyde (I = 3) was employed in this case, too.
In 1986 Kawai et al. isolated Whe II (13) from wheat grain, which showed fruiting-inducing effect on
S. commune. As shown in Scheme 10, the sphingenine part A of 13 was synthesized by the cleavage of epoxide C (= 4) with Grignard reagent B.12 The epoxide C (=4) was epoxy diester D.
The reason why these cerebrosides induces fruiting-body formation of S. commune is not clear yet even now.
3. SPHINGOLIPIDS OF ANIMAL EPIDERMIS
Sphingolipids are building blocks of the plasma membrane of eukaryotic cells. Their function is to anchor lipid-bound carbohydrates to cell surfaces, and to construct the epidermal water permeability barrier. Chemistry of sphingolipids is therefore closely related to dermatology or science of skin.
3-1. Esterified cerebroside of human and pig epidermis
In 1989, Hamanaka and her co-workers isolated a new esterified cerebroside from human epidermis, and found it to be a linoleic acid-containing acylglucosylceramide (14, Scheme 11). She later named 14 "type I epidermoside," because it was specific to epidermis. Her works were reviewed.38 The same structure 14 was also proposed by Downing and his co-workers for the cerebroside which they isolated from pig epidermis. The esterified cerebroside 14 works as a functional water barrier in the skin.
We became interested in synthesizing 14 to confirm the proposed structure, and also to supply a sufficient amount of 14 to dermatologists for its further study. Scheme 11 shows our retrosynthetic analysis of 14.39 Bond disconnection of 14 leads to D-glucose (A), (2S,3R,4E)-4-icosasphingenine (C20-sphingosine, B), 30-hydroxytriacontanoic acid (C), and linoleic acid (D) as the necessary building blocks. C20-Sphingosine (B) can be prepared from 1-heptadecyne (E) and L-serine, while C can be derived from 15-pentadecanolide (F), a musk perfume.
Our synthesis of 14 in 1991 is summarized in Scheme 12.39 Garner's aldehyde (3) was converted to D via A, B and C. It was necessary to protect the amino group of B as trichloroacetamide, which could be removed readily in a later stage. Synthesis of the p-nitrophenyl ester J of 30-hydroxytriacontanoic acid was rather complicated, starting from 15-pentadecanolide. Its methanolysis followed by oxidation with pyridinium chlorochromate (PCC) furnished aldo ester E, one of the building blocks. In the route leading to the other building block, methyl 15-hydroxypentadecanoate was silylated to give F, which was reduced to furnish an alcohol. The alcohol then gave bromide G. A Wittig reagent derived from G was coupled with E to afford H, whose double bond was hydrogenated to give methyl 30-TBDPSoxytriacontanoate (I). After converting I to J, the hydroxy group of J was acylated with linoleyl chloride (K) to furnish the desired key building block, L.
Glycosylation of the sphingosine moiety D with acetobromo-D-glucose (M) smoothly gave β-D-glucopyranoside N. After removing the acetyl and trichloroacetyl groups of N, the free amino group was acylated with L to afford, after desilylation, type I epidermoside (14).39 Thus we obtained 74 mg of 14 as a waxy solid melting at 122-125 oC. Its 1H-NMR spectrum was identical with that of the natural product, and therefore the structure 14 of type I epidermoside was confirmed.
The extremely lengthy esterified side-chain of 14 may function as a connecting livet in the lipid bilayer system of epidermis. We also synthesized ceramide 1 (Scheme 13), the free and extractable ceramide of human epidermis.40
3-2. Ceramide B, 6-hydroxylated ceramide in human epidermis
Ceramides are predominant lipids of human epidermis, acting as the water barrier to prevent loss of body water. They are classified into two groups, free ceramides and protein-bound ones. In the former case, the acyl side-chain of ceramides does not possess a terminal hydroxy group, while in the latter it is present to enable the binding of ceramides with proteins. In 1994, Downing and his co-workers reported the isolation and identification of ceramide B (15, Scheme 13), a protein-bound ceramide. It is a new 6-hydroxy-4-sphingenine-based ceramide. Its structure 15 was assigned by Downing et al. by extensive 1H-NMR studies.
As to the stereochemistry of 15, it almost certainly possesses (2S,3R,4E)-configuration, since all the
known mammalian sphingosines possess that configuration. However, the absolute configuration at C-6 of 15 remained unknown. We became interested in solving this problem by synthesizing both 6R- and 6S- isomers of ceramide B as shown in Scheme 13.41,42
Ceramide B (15) can be synthesized by connecting the sphingosine part A with the acyl part B. Preparation of B can be achieved as shown in Scheme 12. It was an intermediate for the synthesis of type I epidermoside (14). The sphingosine part A is to be synthesized from Garner's aldehyde C (= 3) and acetylene D. The problem was how to prepare both the enantiomers of 1-alkyn-3-ol like D.
1-Alkyn-3-ols are versatile intermediates in organic synthesis. In 1978, we reported asymmetric hydrolysis of the acetates of (±)-1-alkyn-3-ols with Bacillus subtilis esterase to give optically active acetates and alcohols.43 Their enantiomeric purities, however, were mediocre. Present availability of a number of commercial lipases changed the situation completely. Treatment of (±)-acetylenic alcohol E with lipase PS-C (Amano Enzyme, Inc) and vinyl acetate in diisopropyl ether gave acetate (R)-F (99% ee) and the recovered alcohol (S)-E (98% ee) almost quantitatively after 10 days at room temperature. If both the enantiomers of 1-alkyn-3-ols are required, lipase-catalyzed asymmetric acetylation leads to their successful preparation.
The acetate (R)-F was then converted to D, which was coupled with Garner's aldehyde C to give G. The building block A was prepared from G, and coupled with acid B to furnish H. Global desilylation of H afforded ceramide B (15) with 6R-configuration. Similarly, by employing (S)-I as an intermediate, (2S,3R,4E,6S)-15' was synthesized.
Both 15 and 15' were then acetylated, and the 1H-NMR spectra at 500 MHz of the two acetyl derivatives were compared with that of the acetyl derivative prepared from the natural ceramide B. By this comparison, it became clear that ceramide B is (2S,3R,4E,6R)-15. There were observed completely different signal patterns at δ = 5.0-5.8, and the acetyl derivative prepared from 15 showed the identical 1H-NMR spectrum with the authentic spectrum of the acetylated ceramide B. It is said that the content of ceramide B in old people is larger than that in young people. The hydroxylation at C-6 may reflect the aging of epidermis.
4. MARINE SPHINGOLIPIDS WITH BIOACTIVITIES OF MEDICINAL INTEREST
4-1. Aplidiasphingosine
The first target molecule in our sphingolipid synthesis started in 1979 was aplidiasphingosine (16, Figure 4), which was isolated by Carter and Rinehart, Jr. in 1978 as an antimicrobial and antitumor amino alcohol from an Aplidium sp. of marine tunicate.
We first synthesized 16 as a diastereomeric mixture,44,45 then synthesized its various stereoisomers to analyze their stereostructures by 13C-NMR spectroscopy,45-47 and finally synthesized
(2R,3R,5R,13S,14R)-16 by employing (R)-citronellic acid as a starting material and adopting Sharpless asymmetric epoxidation as a tool to control the stereochemistry at C-2 and C-3.48,49 Our synthetic (2R,3R,5R,13S,14R)-(+)-aplidiasphingosine (16) showed 13C-NMR spectral data identical to those reported for the natural product. Unfortunately, however, Carter and Rinehart, Jr. reported no chiroptical data for the natural product. We were therefore unable to determine the absolute configuration of the natural aplidiasphingosine. It must be noted that some chemists did not report anything about the chiroptical properties of their isolated products, even in 1970s and 1980s.
4-2. Symbioramide
In 1988, J. Kobayashi et al. isolated a novel ceramide symbioramide (17, Scheme 14) from the laboratory-cultured dinoflagellate Symbiodium sp. obtained from the inside of gill cells of the Okinawan bivalve, Fragum sp. At 10–4 μM concentration, it increases the sarcoplasmic reticulum Ca2+-ATPase activity by 30%. It also exhibits antileukemic activity against L1210 murine leukemia cells in vitro with an IC50 value of 9.5 μg/mL. Kobayashi's work coupled with Nakagawa's synthetic work established the stereochemistry of symbioramide as (2S,3R,2'R,3'E)-17.
We achieved a synthesis of symbioramide as shown in Scheme 14.50 Synthesis of the α-hydroxy-β,γ-unsaturated carboxylic acid moiety H started from 1-bromopentadecane (A). Chain-elongation of A with dianion of propargyl alcohol was followed by E-selective reduction of the triple bond to give allylic alcohol B. Sharpless asymmetric epoxidation of B with L-(+)-diethyl tartrate as the ligand was followed by TBS-protection of the hydroxy group to furnish C. Treatment of C with diphenyl diselenide and sodium borohydride yielded D as the major product. After oxidation and chromatographic purification, the desired F was obtained in 75% yield based on C. Protection of the secondary hydroxy group of F with t-butyldiphenylsilyl chloride gave G, whose TBS group was selectively removed with acetic acid, and the resulting alcohol was oxidized with Jones chromic acid to furnish the key building block H.
The synthesis of the dihydrosphingosine moiety L could be achieved readily, starting from L-serine (I). Garner's aldehyde (3) was treated with lithium pentadecynide to give J. Hydrogenation of J afforded K, which was deprotected to give C18-dihydrosphingosine. Protection of its hydroxy groups furnished L, which was acylated with H to give protected ceramide M. Global deprotection of M in two steps afforded crystalline symbioramide (17), which was spectroscopically identical with the natural product.
Optical rotation values of symbioramide (17) taught us an interesting lesson. The temperature of the sample solution in a cell for rotation measurements influences the sign and magnitude of the specific rotation of 17. The synthetic sample of ours showed [α]D22 = –1.5 (c 0.24, CHCl3), while the natural 17 showed [α]D19 = +5.8 (c 1, CHCl3). This discrepancy annoyed us. We measured the rotation of 17 very carefully using a polarimeter equipped with a cell in a constant temperature bath. On this apparatus, the specific rotation of our synthetic 17 was recorded as: [α]D19 = +3.6, [α]D23 = +0.76, [α]D28 = –1.5, [α]D35 = –5.5 (c 0.31, CHCl3). In our previous measurement which gave the value [α]D22 = –1.5 (c 0.24, CHCl3), the room temperature was 22˚C. During the measurement, however, the temperature of the sample solution was raised by the irradiation with the sodium D-line light. The temperature of the sample solution was therefore higher than 22˚C, and we recorded a negative rotation. The discrepancy was thus removed, and the absolute configuration of symbioramide (17) was reconfirmed as depicted. The temperature dependence of the specific rotation value is a well-known but often overlooked phenomenon, which must be taken into account properly.
4-3. Penazetidine A
In 1994, Crews and his co-workers isolated and identified penazetidine A (18, Scheme 15), an inhibitor of protein kinase C, from the Indo-Pacific marine sponge, Penares sollasi. Its structure 18 was proposed on the basis of NMR and MS studies, although nothing was known about its absolute configuration. Assuming that its biosynthesis is in accord with that of other sphingosines, we synthesized both (2S,3R,4S,12'R)-18 and (2S,3R,4S,12'S)-18' as shown in Scheme 15.51,52
For the synthesis of (2S,3R,4S,12'R)-penazetidine A (18), (S)-citronellol and (S)-Garner's aldehyde (F = 3) were employed as our starting materials. Chain-elongation of (S)-citronellol under the Schlosser conditions gave A, whose double bond was cleaved to furnish aldehyde B. Treatment of B with lithium nonynide gave C, which was subjected to acetylene zipper reaction to afford D. Deoxygenation of D yielded (R)-E. Coupling of (R)-E with (S)-Garner's aldehyde (F = 3) gave G which was converted to epoxide I via H. Reduction of I with DIBAL-H was followed by mesylation to give mesylate J. This was treated with sodium hydride to give azetidine K. Removal of its tosyl and TBS protective groups gave (2S,3R,4S,12'R)-penazetidine A (18). Similarly, (R)-citronellol was converted to (2S,3R,4S,12'S)-18'. The 1H- and 13C-NMR spectra of both 18 and 18' were identical and in accord with the authentic spectra of the natural product provided by Prof. Crews. Both 18 and 18' as well as natural penazetidine A were levorotatory, and it was impossible to decide which of 18 and 18' is the natural product.
4-4. Penaresidin A and B
In 1991, penaresidin A (19, Scheme 16) and B (20, Scheme 16), actomyosin ATPase activators,
were isolated by J. Kobayashi et al. from the Okinawan marine sponge Penares sp., and characterized as a mixture of the corresponding tetraacetyl derivatives. They were shown to be azetidine alkaloids related to phytosphingosines. Their absolute configuration, however, was not known. We assumed the absolute configuration of the azetidine moiety of penaresidins to be 2S,3R,4S, considering the possible biogenetic relationship between penaresidins and phytosphingosines, and started their synthesis.
Our synthesis of penaresidin A and B is summarized in Scheme 16.52-54 For the synthesis of penaresidin A (19), L-isoleucine was chosen as the starting material. Epoxide (2S,3S)-A was prepared from L-isoleucine. Treatment of A with lithium 1-decynide yielded B, which was subjected to
acetylene zipper reaction to give terminal acetylene C. Mitsunobu inversion of the (R)-hydroxy group of C to furnish D was followed by hydrolysis and silylation to give E.
Alkynylation of Garner's aldehyde F (= 3) with alkyne E gave G with the required carbon skeleton. The triple bond of G was then reduced to give (E)-alkene H. The next epoxidation was unfortunately non-stereoselective to give the required epoxide I and its isomer. Reduction of the epoxide I was fortunately selective with DIBAL-H as the reducing agent, and J was obtained after mesylation.
Treatment of J with sodium hydride in THF closed the azetidine ring smoothly to give K in 81% yield. Then K was reduced with sodium naphthalenide to remove the tosyl group. Penaresidin A [(2S,3R,4S,11'S,12'S)-19] was obtained after further deprotection of the TBS group. Peracetylation of 19 afforded the tetraacetyl derivative, whose spectroscopic and chiroptical properties were in accord with those of the tetraacetyl derivative of the natural product. Isomers of penaresidin A with (2S,3R,4S,11'R,12'S)- and (2S,3R,4S,11'R,12'R)-configurations were also synthesized, but their physical properties were different from those of the natural penaresidin A.
Kobayashi et al. initially proposed a structure shown in the lower part of Scheme 16 for penaresidin B. Our synthesis of 19 made it possible to critically analyze the 13C-NMR spectrum of the tetraacetyl derivatives of the naturally occurring mixture of penaresidin A and B. Consequently, we proposed 20 as the structure of penaresidin B. Our proposal was confirmed by its synthesis. L-Leucine was converted to alkyne M via epoxide L. The alkyne M yielded 20, whose spectral properties were in accord with those of penaresidin B.54
Absolute configuration at C-11' of penaresidin A and B were determined as S also by J. Kobayashi et al. on the basis of 1H-NMR data of their tris-O-MTPA esters.55 As you have seen in this case, synthesis of 19 and 20 was the key to firmly establish the structures of penaresidin A and B.
4-5. Sulfobacin A, B and flavocristamide A
In 1995, sulfobacin A (21, Scheme 17) and B (22), von Willebrand factor receptor antagonists, were isolated by Kamiyama et al. from the culture broth of a terrestrial bacterium Chryseobacterium sp. In the same year, the isolation of flavocristamide A (23) and B (= sulfobacin A, 21), DNA polymerase α inhibitors, from the cultured mycelium of marine bacterium Flavobacterium sp. in Hokkaido was reported by J. Kobayashi et al. These are sulfonolipids, and unusual sphingosine derivatives. We became interested in synthesizing these three sulfur-containing compounds, and carried out their synthesis from L-cysteine.56,57
Sulfobacin B (22), the simplest member of these sulfonolipids, was synthesized as shown in Scheme 17. L-Cysteine was converted to C via A and B. Attachment of a side-chain to C furnished D, which was hydrogenated over Adams's platinum oxide to give E. Sultine F was generated, when E was
treated with hydrochloric acid. This conversion protected the hydroxy group at C-3. Acylation of F with G gave H, whose sultine ring was cleaved with ammonia to afford I. Oxidation of sulfinic acid I with hydrogen peroxide furnished sulfobacin B (22).56,57
For the synthesis of sulfobacin A (21) and flavocristamide A (23), TBS ether of (R)-3-hydroxy-15- methylhexadecanoic acid was necessary, which was synthesized from 10-bromo-1-decanol (A) as
shown in Scheme 18. Chain-elongation of A under the Schlosser conditions gave B, which was oxidized with PCC to give aldehyde C. (±)-β-Hydroxy ester D was prepared from C by treatment with ethyl acetate and LDA. The corresponding (±)-acid was acetylated with vinyl acetate in the presence of lipase PS to give enantiomerically pure (R)-hydroxy acid and the acetylated (S)-acid. The former was converted to its TBS ether E.
For the synthesis of flavocristamide A (23), (E)-iodoalkene (I) was necessary. This was prepared from 9-decen-1-ol (F). Chain-elongation of F yielded G, whose double bond was converted to the triple bond to give H. Subsquent hydroalumination of H was followed by quenching with iodine to give the desired iodoalkene I.
Sulfobacin A (21) was obtained by acylation of the amine J with E followed by deprotection and oxidation,56,57 while flavocristamide A (23) was synthesized from K by alkenylation with I to give L. In the same manner as for sulfobacin A, L was converted to flavocristamide A (23) via M.57
4-6. Plakoside A
In 1997, Fattorusso and his co-workers isolated plakoside A (24, Scheme 19) as a metabolite of the Caribbean sponge, Plakortis simplex. It is structurally unique as a glycosphingolipid with a prenylated D-galactose moiety and cyclopropane-containing alkyl chains, and shows strong immunosuppressive activity without cytotoxicity. Only 5 mg of plakoside A could be secured from 57 g (dry weight) of the sponge. Since the absolute configuration at the stereogenic centers of the two cyclopropane moieties was unknown except that they were cis-disubstituted cyclopropanes, we decided to synthesize two diastereomers of plakoside A, (2S,3R,11S,12R,2'''R,5'''Z,11'''S,12'''R)-24 and (2S,3R,11R,12S,2'''R,5'''Z,11'''R,12'''S)-24', anticipating that one of them would be the natural product. We assumed that the two cyclopropane-containing side-chains in a given molecule have the same absolute configuration due to the enantioselective biocyclopropanation process.
Schemes 19 and 20 summarizes our synthesis of plakoside A (24) and its diastereomer 24' in 2001.58,59 As will be shown later in this section, plakoside A was proved to be 24, not 24'. Plakoside A (24) can be prepared from the three building blocks, A, B and C. D-Galactose will be the starting material for A, while B and C can be synthesized from cyclopropane alcohol D and L-serine or D-glutamic acid, respectively.
The monoacetate D was obtained by lipase-catalyzed desymmetrization of meso-diol E. Chain-elongation of D afforded F. The corresponding aldehyde G was subjected to a Wittig reaction to give H. Diimide reduction of H saturated the double bond, and further functional group transformation gave iodide I. Treatment of I with lithium acetylide-ethylenediamine complex furnished J, which was coupled with Garner's aldehyde K (= 3) to give L. Diimide reduction of L smoothly furnished M, whose deprotection and silylation yielded the key building block B. Similarly, the diastereomeric alkyne J' afforded B'.
Scheme 20 shows the completion of the synthesis. Alcohol A was converted to phosphonium salt B, which was coupled with C by the Wittig reaction to give D. Deprotection, bis-silylation, and mono-desilylation of D furnished alcohol E, which was oxidized to the key building block F (= C of Scheme 19). Acylation of the sphingosine part G with the acid F was executed in the presence of
N,N'-dicyclohexylcarbodiimide (DCC) and 1-hydroxybenzotriazole (HOBt). The product was partially desilylated to afford H. Glycosidation of the ceramide H with I gave J. Treatment of J with hydrazine acetate removed its monochloroacetyl (ClAc) protective group. Then the product was treated with prenyl trichloroacetimidate (K) to attach a prenyl group at C-2' of the galactose moiety. Subsequent desilylation and deacetylation yielded plakoside A (24). Similarly, three building blocks A', G' and I were assembled to give 24'.58,59
In 2000, Nicolaou et al. synthesized 24' by a different route, found its 1H- and 13C-NMR spectra to be identical to those of plakoside A, and claimed the structure of plakoside A as 24'.60 We found that both 24 and 24' exhibit entirely identical 1H- and 13C-NMR spectra. Moreover, they were in complete agreement with those reported for the natural product. Although 24 and 24' are diastereomeric, their stereogenic centers are separated by seven or eight carbon atoms, and they therefore showed identical spectroscopic properties. It was concluded that the absolute configuration of plakoside A could not be solved by its synthesis alone. Degradation and derivatization of plakoside A seemed necessary to solve the stereochemical problem.
Consequently, we decided to resume degradation studies on natural plakoside A. Prof. Fattorusso reisolated 5 mg of plakoside A from the Caribbean sponge. Our strategy is shown in Scheme 21. Degradation of plakoside A pentaacetate (A) will give two cyclopropane acids D and E resulting from the two carbon-chains. By determining the absolute configuration of D and E, we will be able to determine the stereochemistry of plakoside A. Then, what kind of analytical method will enable us the stereochemical assignment ? HPLC analysis of esters F and G prepared from D and E after derivatization with Ohrui's chiral and fluorescent reagent (1S,2S)- and (1R,2R)-R*OH will meet the challenge.61
Degradation of plakoside A pentaacetate (A, 2.0 mg) was executed by first treating it with nitrous acid in acetic anhydride through N-nitrosation at the amide nitrogen of A to give B and C, which were further cleaved to give D and E, respectively.62,63 A mixture of D and E was derivatized with Ohrui's reagent R*OH, and the products F and G were subjected to HPLC analysis at the column temperature of –50˚C. Owing to the presence of the anthracene ring in F and G, their picogram quantities were detectable by fluorescence, and therefore minute amounts of the degradation products could be analyzed.
In order to determine the absolute configuration of D and E, it was necessary to prepare the synthetic reference samples of known absolute configuration. Conversion of meso-H to monoacetate I was followed by further synthetic steps to give D, D', E and E', all the possible candidates of D and E obtained by degradation. These were derivatized with Ohrui's reagent R*OH, and analyzed by HPLC. The esters derived from plakoside A were (6S,7R)-F and (9S,10R)-G. Accordingly, the absolute configuration of plakoside A must be (2S,3R,11S,12R,2'''R,5'''Z,11'''S,12'''R)-24.62,63
A combination of enantioselective synthesis and HPLC analysis is a powerful method for
determination of the absolute configuration of a compound with stereogenic centers remote from other functionalities and stereogenic centers.
5. GLYCOSPHINGOLIPIDS WHICH STIMULATE NATURAL KILLER T CELL
5-1. KRN7000
KRN7000 (25) is an anticancer drug candidate developed by researchers at Kirin Brewery Company.64 It was obtained through the modification of the structures of agelasphins (see Scheme 22 for the structure of agelasphin 9b), which had been isolated in 1993 as anticancer glycosphingolipids from the extract of an Okinawan marine sponge, Agelas mauritianus. These glycosphingolipids exhibit anticancer activity in vivo in mice and humans, while they show no cytotoxicity at all in vitro. As some of K.M.'s former students joined Kirin Brewery Co., and K.M. too had a good relationship with Kirin, we knew the structure 25 of KRN7000. Indeed in 1998, we published a synthesis of KRN7000 as shown in Scheme 22.65
Our synthesis of KRN7000 started from C18-sphingosine, which was prepared from Garner's aldehyde. The key step was the stereoselective epoxidation of A to give B as the major product. This epoxidation was examined under various different conditions. MCPBA gave a mixture of epoxides, B having been the minor isomer [cf. Schemes 15 (H → I) and 16 (H → I)]. Fortunately, dimethyldioxirane in acetone was found to give B as the major product (β-epoxide B/α-epoxide = 84:16 after 3 days at 4˚C). Reductive cleavage of the epoxy ring of B with DIBAL-H in toluene took place regioselectively to give the desired alcohol C.
Reductive removal of the N-tosyl group of C gave D, to which was added the acylating agent E to furnish F. Silylation of F was followed by partial desilylation of the persilylated product to provide G. Galactosylation of G with fluorosugar H gave the protected form I of the target molecule. Desilylation followed by debenzylation of I afforded KRN7000 (25). In 1998, synthesis of phytosphingosine derivative like D was rather complicated and cumbersome as shown here. At present, however, phytosphingosine is manufactured by fermentation, and commercially available as already described in section 1-3 (2).
Taniguchi and his co-workers have shown that KRN7000 is a ligand to make a complex with CD1d (CD = cluster of differentiation) protein, a glycolipid-presenting protein on the surface of the antigen-presenting cells of the immune system.66,67 Two lipid alkyl chains of 25 are bound in grooves in the interior of the CD1d protein, and the galactose head group of 25 is presented to the antigen receptors of natural killer (NK) T cells of the immune system (Figure 5).
After activation by recognition of CD1d-25 complex, NKT cells release both helper T(Th)1 and Th2
types of cytokines at the same time in large quantities. Th1 type cytokines such as interferon(IFN)-γ mediate protective immune functions like tumor rejection, whereas Th2 type cytokines such as interleukin(IL)-4 mediate regulatory immune functions to ameliorate autoimmune diseases. Th1 and Th2 type cytokines can antagonize each other's biological actions. Because of this antagonism, use of
KRN7000 for clinical therapy has not been successful yet. Our task in 2003 was to synthesize glycosphingolipids, which induce NKT cells to produce preferentially either Th1 or Th2 type cytokines.
In 2003, when we began our work, there were two remarkable analogues of KRN7000 as shown in Scheme 22. Franck, Tsuji, and their co-workers reported that their synthetic α-D-C-galactosylceramide (α-C-GalCer, Scheme 22) caused an enhanced Th1 type response in vivo in mice.68 On the other hand, Miyamoto et al. found that OCH (Scheme 22), an analogue of KRN7000 with a truncated sphingosine alkyl chain, caused NKT cells to produce IL-4 predominantly.69
There are three excellent reviews available to understand the chemistry and biology of KRN7000 and related compounds.70-72
5-2. Analogues of KRN7000 prepared in 2003-2006
There are two options in choosing the strategy for drug discovery. One is to depend on high-throughput screening of a huge number of drug candidates produced by combinatorial chemistry. The other is to design candidate compounds by so-called "structure-based design" using the structural information obtained by X-ray crystallographic analysis of a lead compound and its receptor protein. This is a rational way. In our case, if we can have the structural information about CD1d protein-KRN7000-T cell receptor protein complex as revealed by X-ray crystallography, we can design possible drug candidates. In 2003 when we started our works, there was no X-ray information available. Accordingly, we had to design our drug candidates empirically or by trial and error.
We first wanted to clarify the effect of conformational restriction in the ceramide part as caused by azetidine or pyrrolidine ring formation (Figure 6).73 We synthesized many compounds, and gave them code names as RCAI-1, RCAI-2, RCAI-3 and so on. RCAI stands for Research Center for Allergy and Immunology, RIKEN. Thanks to our experience in synthesizing azetidines such as penazetidine A (Scheme 15) and penaresidin A and B (Scheme 16), we quickly synthesized RCAI-18 and RCAI-51.
They could induce the production of cytokines just like KRN7000. But their potencies could not surpass that of KRN7000, although RCAI-18 was almost as active as KRN7000. No preferential production of either Th1 or Th2 type of cytokines could be observed.73
We also synthesized compounds with a single acyl-chain and a spacer such as RCAI-5, because Elofsson and his co-workers reported an analogue which could be presented by CD1d protein.74 However, both RCAI-5 and RCAI-6 were almost inactive.
In 2004, Zhou et al. proposed isogloboside 3 to be the natural ligand for CD1d protein.75 Their claim might mean that the presence of a spacer between D-galactose and sphingosine could be allowed for the NKT cell activation. We synthesized RCAI-16, 28 and 30. All of them were inactive or only very slightly active.
Bioisosterism is an important and useful concept in drug design. Replacement of an atom in a bioactive compound by an atom of other elements of course gives a different compound. Sometimes, however, the new compound can also be bioactive. We therefore imagined that replacement of carboxamide in KRN7000 by sulfonamide linkage might give bioactive analogues. Accordingly, sulfonamide analogues such as RCAI-17, 25 and 36 were synthesized and bioassayed.76 These analogues were shown to be the stimulants of mouse NKT cells to induce the production of Th2-biased cytokines such as IL-4 in vitro.
At the end of 2006, we were really waiting for the success of the X-ray crystallographic analysis of the CD1d protein-KRN7000-T cell receptor protein complex so that we would be able to achieve more meaningful work in this area.
5-3. Cyclitol, carbasugar and modified D-galactose analogues of KRN7000: RCAI-56 and RCAI-61
In 2005, an X-ray crystallographic analysis revealed the structure of the complex of human CD1d and KRN7000.77 It was proved that the two lipid alkyl chains of KRN7000 are bound in the interior of the CD1d protein, and the galactose head group of KRN7000 is presented to the outside. However, we could not know anything about the roles of the oxygen functions of galactose in interaction with the T cell receptor. The awaited and decisive X-ray crystallographic structure of a human T cell receptor in complex with CD1d bound to KRN7000 was published in 2007 by McClusky, Rossjohn and their co-workers in Australia and UK.78
It was revealed that human T cell receptor of NKT cell is interacting with the 2'-, 3'- and 4'-hydroxy groups of the galactose part of KRN7000, while 6'-hydroxy group is not involved in this hydrogen bond network with any residues of human CD1d or T cell receptor. Moreover, it was found that the oxygen atom of the galactopyranose ring makes no hydrogen bonding with CD1d or T cell receptor, while the glycosidic oxygen atom makes a hydrogen bonding with CD1d. We therefore started our works to synthesize cyclitol or carbasugar analogues lacking the oxygen atom of the pyranose ring.79,80
Modification at 6'-hydroxy group of galactose was also attempted.81
Among carbasugar and cyclitol analogues of KRN7000, RCAI-56 (26), RCAI-59 and RCAI-92 (Scheme 23) were remarkably potent stimulants of mouse lymphocytes to produce Th1-biased cytokines such as interferon-γ in vivo. They were over 5 times more potent than KRN7000. Substitution of the pyranose oxygen with a methylene group turned out to be quite a successful way to enhance the bioactivity of the analogues of KRN7000.79,80
Synthesis of RCAI-56 (26) is summarized in Scheme 23. So as to obtain D-carbagalactose, methyl α-D-galactopyranoside was converted to A, which was subjected to palladium-catalyzed Ferrier rearrangement to give B. The keto cyclitol B served as the key-intermediate to furnish 2,3,4,6-tetrabenzylated carbagalactose C. As to the sphingosine part, we were happy to purchase phytosphingosine manufactured by fermentation. Phytosphingosine was converted to Bittman's cyclic sulfaimidate D, which was coupled with the carbasugar C to give E. Deprotection of E afforded F, which was acylated to furnish RCAI-56 (26). This was a lengthy synthesis, and the overall yield of 26 was 6.7% based on methyl α-D-galactopyranoside (16 steps).79,80 Although its bioactivity is remarkable, RCAI-56 is rather difficult to prepare efficiently with a low cost.
Our subsequent attempts were to modify the primary hydroxy group (6'-OH) of KRN7000, and provided various analogues as shown in Scheme 24. RCAI-61 (27) was shown to be the most promising analogue of KRN7000 because of its synthetic availability and high bioactivity. RCAI-61 (27) brought about highly remarkable increase (x 8.2 times stronger than KRN7000 as estimated by total amount of the produced interferon-γ at the dosage of 2 μg/mouse in vivo) in the production of interferon-γ even at a low concentration.81
Synthesis of RCAI-61 (27) is shown in Scheme 24. Fluorosugar A, prepared from methyl α-D-galactopyranoside, was coupled with ceramide B to give C, whose deprotection furnished RCA-61 (27). The overall yield was 21% (10 steps) based on methyl α-D-galactopyranoside.81 The short and efficient synthetic route leading to 27 makes it a promising drug candidate. "Structure-based design" was eventually fruitful. Reliable X-ray crystallographic structure determination evokes imagination of chemists to design better drug candidates.
5-4. Other analogues of KRN7000 and their immuno-stimulating activities
A number of analogues of KRN7000 have been synthesized and assayed by many research groups around the world. Some of those analogues showed Th1- or Th2-biased cytokine production compared to KRN7000 (25). However, the definitive partial structures of 25 or the critical factors which induce biased cytokine production by NKT cells have not been clarified yet. The accumulation of the results on the structure-activity relationship studies will clarify them in the future.
The structure of 25 contains four modifiable parts: (i) the lipid chains, (ii) the galactose-ceramide linkage, (iii) the polar part of ceramide, and (iv) the sugar part. In this section, we summarize the structures of the analogues of 25 together with their bioactivities, basing on the above four categories.
(1) Modification of the two lipid alkyl chains of KRN7000
The researchers at KIRIN Brewery Co. examined the relationship between the chain length of the ceramide part of KRN7000 (25) and biological activity.64 They found that the analogue with a longer alkyl chains showed a more potent antitumor activity induced by Th1-type cytokines. In 2004, Oki et al. suggested that IFN-γ production by NKT cells required a longer period of TCR stimulation than for IL-4 production.82 The glycolipids with shorter sphingosine or fatty acyl chains like OCH (Scheme 22, A in Figure 7) make less stable complex with CD1d than 25, therefore, they can stimulate NKT cells for a shorter period than 25 can do, and induce Th2-biased cytokine response.82 The correlation between the chain length of the lipid part and cytokine response was examined by Savage and his co-workers in 2004, and also by the researchers at Daiichi Asubio Pharma Co. in 2007, independently.83,84
Yu et al. reported some analogues of 25 with truncated and unsaturated N-acyl group (the most optimized analogue is C20:2, B in Figure 7), which induced Th2-biased cytokine production.85 Recently, Kim and his co-workers found that the analogue of 25 with a di(n-heptyl)amino group at the end of the acyl chain (C) induced enhanced and biased IL-4 production in mice in vitro.86 According to the docking model of Kim’s analogue with CD1d, two branched alkyl chains of the amino group of C effectively filled the donuts-type A’ pocket. It should be noted that these amino-branched analogues possess higher solubilities in both aqueous and organic solvents than 25.
Wong and his co-workers introduced an aromatic ring at the end of the fatty acyl chain.87-89 They expected that their analogues possess additional π-π stacking interactions with the aromatic amino acid residues at the bottom of the hydrophobic pocket of CD1d, leading to increased complex stability. Their aromatic acyl analogues, C8Ph (D, Figure 7) and its relatives, indeed exhibited a stronger Th1-biased cytokine response in humans in vitro.87-89 They performed quantitative microarray analysis to investigate the correlation of the binding affinity of the aromatic analogues to CD1d with produced Th1/Th2 cytokine balances.89 C8PhF (E) showed the highest affinity with CD1d and the largest Th1/Th2 ratio. The docking model study of these aromatic analogues to CD1d supports these results of affinity analyses.90
(2) Modification of the galactose-ceramide linkage
α-C-GalCer (Scheme 22) is included in this group. It has the stable ether bond instead of an acetal linkage and is resistant against enzymatic degradation or decomposition in the low pH environment. This functional stability of α-C-GalCer caused stable presentation by CD1d, prolonged stimulation of NKT cells, and a great downstream activation of NK cells to produce a large amount of IFN-γ for a long-term.91
As described in section 5-1, α-C-GalCer induced a potent Th1-type response in mice in vivo.68,92 Although α-C-GalCer showed rather weak activities against human lymphocytes, its improved
analogue, GCK152 (Figure 8) with both an E-alkene linker production against human lymphocytes.93 Synthesis of the S-glycoside analogue of KRN7000 (25) (α-S-GalCer, Figure 8) with no bioactivity was also reported.94,95
The spacer analogues of 25 (RCAI-16, 28 and 30, Figure 6) are included in this group. One-methylene-truncated (nor-) and elongated (homo-) C-glycoside analogues of 25 were also synthesized and bioassayed.96,97 The former induced weak and Th1-biased cytokine production, while the latter showed no bioactivity at all. From the X-ray information about TCR-25-CD1d complex,77,78 spatial relationship between the galactose and the ceramide part seems to be very important to make a rigid complex with CD1d so as to show potent bioactivities.
(3) Modification of the polar part of the ceramide
All of the eight diastereomers of KRN7000 (25) were synthesized and bioassayed.98 Among them, 4-epi-KRN7000 (A, Figure 9) activated NKT cells to induce similar levels of cytokines compared with 25.98,99 In addition, both of the diastereomers of 4-deoxy-4-amino analogues (B, C) showed moderate bioactivity.100 These results indicates that the 4-hydroxy group of 25 is modifiable,
and the absolute configuration at 4-position has no influence on its bioactivity. In addition, it is known that 4-deoxy analogue of 25 (D, Figure 9) also shows a potent bioactivity in mice and humans.101,102 On the other hand, 4-deoxy-4,4-difluoro analogue E, one of the mimics of D, induced only moderate and slightly Th1-biased cytokine production.103 According to Wong, a galactosyl serine analogue F showed weak bioactivity, and therefore a hydroxy group at the 3-position is preferable as a proton donor.104 Among the four stereoisomers of 4-deoxy (sphinganine-type) analogues, the natural (2S,3R)-isomer showed the strongest antitumor effects.105,106 As the synthetic building block, D-erythro-sphinganine [(2S,3R)-2-amino-1,3-octadecanediol] is commercially available, although it is terribly expensive. In contrast, D-ribo-phytosphingosine [(2S,3S,4R)-2-amino-1,3,4-octadecanetriol] can be obtained in a large quantity by fermentation as we described in 1-3 (2), and commercially available at a reasonable price.
Isosteric replacement of the 2-amido moiety of 25 with a 1,2,3-triazole group (G)107 or an ester (H)108 were reported, and led the analogues to Th2-type cytokine production. Besides the sulfonamide analogues (Figure 6), an a,a-difluoroacylated one (I) with an acidic amide NH also showed the Th2-biased cytokine response.109 We found that an ureido analogue (J) induced Th1-type cytokine production.110 The order of the pKa values of amide NH is ureide > carboxamide > sulfonamide ≈ α,α-difluoro amide. These results suggested that the analogue which has an amide NH with a small pKa value shows the Th2-type response. This amide NH makes a hydrogen bonding with Thr156 of mouse CD1d (human: Thr154). Therefore, the strength of that hydrogen bonding seems to be the key factor to lead the analogue to either Th1- or Th2-biased.
Recently, Park et al. reported that 3,4-dideoxy analogue of 25 with an aromatic acyl chain (K) induced a potent Th2-biased cytokine response.111
(4) Modification of the sugar part of KRN7000
The sugar part of KRN7000 (25) is an α-D-galactopyranose. Until the discovery of 25, many mono- and disaccharide analogues of glycosphingolipids were synthesized. Koezuka and his co-workers investigated immunostimulatory and antitumor activities, and an enhancing effects on NK cell activity of many kinds of monoglycosyl ceramides.66,106,112,113 They found that (1) α-epimers were bioactive, whereas β-ones were almost inactive; (2) pyranoses were preferable to furanoses; and (3) among the α-D-glycopyranosyl ceramides, α-D-galactose analogue and α-D-fucose one showed potent bioactivities. From these findings, they concluded α-D-galactopyranoside to be the most preferable agent for the immunostimulation.
Several disaccharide analogues were also examined by Koezuka, Taniguchi, and their co-workers, and they found that the inner sugar linked to the ceramide should have the α-anomeric configuration to show bioactivity.66,114 None of these disaccharides analogues, however, showed more potent proliferative activity than α-galactopyranosyl ceramide, except αGal(1→6)αGalCer (AGL-586).115 This result suggests that the 6-hydroxy group of the galactose moiety of 25 is modifiable without diminution of the bioactivity.
The above suggestion was supported by X-ray crystallographic analysis of CD1d-25-TCR complex.77,78 According to the X-ray information, 2’-, 3’-, and 4’-hydroxy groups of 25 are interacting with Gly96, Ser30, and Phe29 of TCR, respectively. Meanwhile, the 6’-hydroxy group of the galactose is not involved in the hydrogen bonding network.77,78 Therefore, the former three hydroxy groups seem to play important roles to make a stable TCR-antigen-CD1d complex so as to show the potent immunostimulatory activities. Indeed, many analogues were developed by modification of the former three hydroxy groups to other functional groups, for example, H (deoxy), F, N3, NH2, NHAc, OMe, or O(CH2)2OH.69,116-121 However, none of these analogues showed potent bioactivities except 3-O-sulfo-α-galactosyl analogue of 25 (A, Figure 10), which stimulated mouse and human NKT cells to secrete IFN-γ and IL-4 comparable to those caused by 25.117,122,123
Synthesis and bioactivities of 6’-modified analogues of 25 were also investigated. As shown in Scheme 24 in section 5-3, RCAI-61 (27), the 6’-O-methylated analogue of 25, and its related analogues showed a potent IFN-γ production.81 Calenbergh and his co-workers developed 6’-amido and 6’-ureido analogues of 25 (B, C) in 2008.124 They reported that these analogues induced the comparable levels of IFN-γ and the significantly reduced IL-4 productions compared to 25 in mice in vivo (i.p.). Among these analogues, the amide derivatives showed a more pronounced Th1-bias. In addition, synthesis of a 6’-acetamido analogue of 25 (D) was also reported.125 The analogue D showed activity comparable to that of 25, and showed improved solubility in DMSO.
The activities of some carbasugar analogues of 25 were also investigated. RCAI-56 (26, Scheme 23), carba-α-D-galactopyranosyl analogue of 25, and its relatives showed a potent immunostimulant activities in mouse and humans.79,80 These analogues have ether O atom, which can interact with Thr156 (mouse, human: Thr154) of CD1d, and make a rigid complex with CD1d like 25. Additionally, these analogues have an ether linkage instead of an acetal bond, and are stable in vivo as we mentioned in the case of α-C-GalCer.
The carbasugar analogues could therefore stimulate NKT cells for a longer period than 25 can do, and induced the Th1-biased cytokine production. Indeed, RCAI-56 (26) resisted enzymatic degradation more than 25 in liver microsomes assay, and induced the Th1-biased cytokine production.126
Llebaria and his co-workers reported that an aminocyclitol analogue (E, Figure 10) induced weak cytokine production and strong proliferation activity.127 Interestingly, both O- and N-inositol-type analogues of 25 showed no bioactivity.80,127
As the non-glycopyranosyl analogues of 25, Celundolo and his co-workers reported that L-arabitol analogue (F) and L-threitol one (G) also induced cytokine productions.128 Especially, they found that the threitol ceramide could be an effective adjuvant for priming both T and B cell responses.129
5-5. Research aimed to discover the natural and endogenous ligand for NKT cells
Identification of the truly endogenous ligand for NKT cells remains an unsolved mystery at this stage. The existence of endogenous self-ligands has been speculated on the basis of the observation that NKT cells appear to be activated in vivo. Bendelac and his co-workers reported that the frequency and functional properties of NKT cells of germ-free mice were essentially identical to those of normal mice.130 These results suggested that NKT cells could be generated and matured by endogenous
self-ligand(s). As we described in section 5-2, Zhou et al. proposed isogloboside 3 (iGb3, Figure 6) to be an endogenous ligand for NKT cells.75 The crystal structure of mouse CD1d-iGb3 was published by Zajonc et al.,131 however, it should be noted that iGb3 synthase-deficient mice have normal NKT cell function.132 The conclusion has not been drawn yet, whether iGb3 is the really endogenous ligand or not.133
Some candidates of exogenous NKT cell ligands were proposed, such as mycobacterial phosphatidylinositol mannoside (PIM),134 α-galacturonyl ceramide as a component of the cell wall of Sphingomonas,135,136 and diacylglycerol from Borrelia burgdorferi spirochetes.137 Recent investigations on the natural ligand of CD1d are summarized by Kronenberg.138
6. CONCLUSION
We discussed the synthesis of various bioactive sphingolipids of terrestrial and marine origin. There are many other bioactive sphingolipids, whose synthesis could not be discussed in detail. Compound B1a (28, Figure 11) was isolated by Okuyama and Yamazaki as an anti-ulcerogenic principle from an oriental drug (the whole plant of Tetragonia tetragonoides). We synthesized it in 1988, and confirmed its bioactivity.139 Sphingofungin D (29) was isolated as an antifungal metabolite of Aspergillus fumigatus ATCC20857, and its structure was confirmed by our synthesis.140,141 Ceramide 30 was isolated as a female sex pheromone of the hair crab, Erimacrus isenbeckii. We confirmed its structure 30 by synthesis.142 Myriocin is a fungal metabolite isolated as an immunosuppressant. Its structural mofdification by Yoshitomi Pharmaceutical Co. (Now Tanabe-Mitsubishi Pharma) resulted in the development of FTY720 as an immunosuppressant and immunomodulator for multiple sclerosis, an autoimmune disorder.143
In conclusion, sphingolipids and glycosphingolipids show interesting bioactivities of wide ranges. Their synthesis will continue to be an important field to develop new drugs and bioregulators. Highly modified mimics such as FTY720 will be meaningful targets for medicinal chemists to invent. Basic studies in synthetic methods for sphingolipids will continue to be a worthwhile work for synthetic chemists.
The literature survey for this review was made up to the end of November, 2010. For more details on the bioactivities of our RCAI compounds, please see the Supporting Information.
ACKNOWLEDGMENT
We thank our past and present co-workers for their dedicated works in sphingolipid syntheses. Our thanks are also due to Dr. M. Taniguchi (Director of RCAI, RIKEN) for his generous support.
References
1. T. Kolter and K. Sandhoff, Angew. Chem. Int. Ed., 1999, 38, 1532. CrossRef
2. S. Budesser, P. Sawatski, and T. Kolter, Eur. J. Org. Chem., 2003, 2021. CrossRef
3. R. X. Tan and J. H. Chen, Nat. Prod. Rep., 2003, 20, 509. CrossRef
4. J. Liao, J. Tao, G. Lin, and D. Liu, Tetrahedron, 2005, 61, 4715. CrossRef
5. H. Newman, J. Am. Chem. Soc., 1973, 95, 4098. CrossRef
6. P. Tkaczuk and E. R. Thornton, J. Org. Chem., 1981, 46, 4393. CrossRef
7. P. Garner and J. M. Park, J. Org. Chem., 1982, 52, 2361. CrossRef
8. P. Garner, Tetrahedron Lett., 1984, 25, 5855. CrossRef
9. P. Garner, J. M. Park, and E. Malecki, J. Org. Chem., 1988, 53, 4395. CrossRef
10. P. Garner and J. M. Park, Org. Synth., 1991, 70, 18; Org. Synth. Col. Vol., 1998, 9, 300.
11. X. Liang, J. Andersch, and M. Bols, J. Chem. Soc., Perkin Trans. 1, 2001, 2136. CrossRef
12. K. Mori and T. Kinsho, Liebigs Ann. Chem., 1991, 1309. CrossRef
13. M. Obayashi and M. Schlosser, Chem. Lett., 1985, 1715. CrossRef
14. K. Koike, M. Numata, M. Sugimoto, Y. Nakahara, and T. Ogawa, Carbohydr. Res., 1986, 158, 113. CrossRef
15. T. Bär and R. R. Schmidt, Liebigs Ann. Chem., 1988, 669. CrossRef
16. F. J. Black and P. J. Kocienski, Org. Biomol. Chem., 2010, 8, 1188. CrossRef
17. G. Righi, S. Ciainbrone, C. D'Achille, A. Leonelli, and C. Bonini, Tetrahedron, 2006, 62, 11821. CrossRef
18. P. Kumar, A. Dubey, and V. G. Puranik, Org. Biomol. Chem., 2010, 8, 5074. CrossRef
19. L. De Boer and J. F. C. Van der Wildt, US Patent 5,910,425 (June 8, 1999). Degussa Care Specialties, Cosmoferm B. V., Delft, The Netherlands. D-ribo-Phytosphingosine is also available from Tokyo Chemical Industry Co. (TCI Catalogue P1765; JPYen 22400 for 25 g).
20. J.-H. Bae, J.-H. Sohn, C.-S. Park, J.-S. Rhee, and E.-S. Choi, Appl. Environ. Microb., 2003, 69, 812. CrossRef
21. S. Kim, S. Lee, T. Lee, H. Ko, and D. Kim, J. Org. Chem., 2006, 71, 8661. CrossRef
22. S. Torssell and P. Somfai, Org. Biomol. Chem., 2004, 2, 1643. CrossRef
23. A. N. Rai and A. Basu, Org. Lett., 2004, 6, 2861. CrossRef
24. O. V. Singh, D. J. Kampf, and H. Han, Tetrahedron Lett., 2004, 45, 7239. CrossRef
25. A. N. Rai and A. Basu, J. Org. Chem., 2005, 70, 8228. CrossRef
26. V. D. Chaudhari, K. S. A. Kumar, and D. D. Dhavale, Org. Lett., 2005, 7, 5805. CrossRef
27. M. Lombardo, M. G. Capdevila, F. Pasi, and C. Trombini, Org. Lett., 2006, 8, 3303. CrossRef
28. T. Yamamoto, H. Hasegawa, T. Hakogi, and S. Katsumura, Org. Lett., 2006, 8, 5569. CrossRef
29. J. Llaveria, Y. Díaz, M. I. Matheu, and S. Castillón, Org. Lett., 2009, 11, 205. CrossRef
30. A. R. Howell, R. C. Su, and S. K. Richardson, Tetrahedron, 2004, 60, 11327. CrossRef
31. J. A. Molares-Serna, O. Boutureira, Y. Díaz, M. I. Matheu, and S. Castillón, Carbohydr. Res., 2007, 342, 1595. CrossRef
32. K. Mori and Y. Funaki, Tetrahedron Lett., 1984, 25, 5291. CrossRef
33. K. Mori and Y. Funaki, Tetrahedron, 1985, 41, 2369. CrossRef
34. K. Mori and Y. Funaki, Tetrahedron, 1985, 41, 2379. CrossRef
35. Y. Funaki, G. Kawai, and K. Mori, Agric. Biol. Chem., 1986, 50, 615.
36. T. Abe and K. Mori, Biosci. Biotechnol. Biochem., 1994, 58, 1671. CrossRef
37. K. Mori and Uenishi, Liebigs Ann., 1996, 1. CrossRef
38. S. Hamanaka, M. Suzuki, A. Suzuki, and T. Yamakawa, Proc. Jpn. Acad. Ser. B, 2001, 77, 51. CrossRef
39. K. Mori and H. Matsuda, Liebigs Ann. Chem., 1991, 529. CrossRef
40. Y. Masuda and K. Mori, J. Indian Chem. Soc., 2003, 80, 1081.
41. K. Mori and Y. Masuda, Tetrahedron Lett., 2003, 44, 9197. CrossRef
42. Y. Masuda and K. Mori, Eur. J. Org. Chem., 2005, 4789. CrossRef
43. K. Mori and H. Akao, Tetrahedron Lett., 1978, 19, 4127. CrossRef
44. K. Mori and T. Umemura, Tetrahedron Lett., 1981, 22, 4429. CrossRef
45. T. Umemura and K. Mori, Agric. Biol. Chem., 1987, 51, 217.
46. K. Mori and T. Umemura, Tetrahedron Lett., 1981, 22, 4433. CrossRef
47. T. Umemura and K. Mori, Agric. Biol. Chem., 1982, 46, 1797.
48. K. Mori and T. Umemura, Tetrahedron Lett., 1982, 23, 3391. CrossRef
49. T. Umemura and K. Mori, Agric. Biol. Chem., 1987, 51, 1973.
50. K. Mori and K. Uenishi, Liebigs Ann. Chem., 1994, 41. CrossRef
51. A. Yajima, H. Takikawa, and K. Mori, Liebigs Ann., 1996, 1083. CrossRef
52. K. Mori, J. Heterocycl. Chem., 1996, 33, 1497. CrossRef
53. H. Takikawa, T. Maeda, and K. Mori, Tetrahedron Lett., 1995, 36, 7689. CrossRef
54. H. Takikawa, T. Maeda, M. Seki, H. Koshino, and K. Mori, J. Chem. Soc., Perkin Trans. 1, 1997, 97. CrossRef
55. J. Kobayashi, M. Tsuda, J.-F. Cheng, M. Ishibashi, H. Takikawa, and K. Mori, Tetrahedron Lett., 1996, 37, 6775. CrossRef
56. H. Takikawa, S. Muto, D. Nozawa, A. Kayo, and K. Mori, Tetrahedron Lett., 1998, 39, 6931. CrossRef
57. H. Takikawa, D. Nozawa, A. Kayo, S. Muto, and K. Mori, J. Chem. Soc., Perkin Trans. 1, 1999, 2467. CrossRef
58. M. Seki, A. Kayo, and K. Mori, Tetrahedron Lett., 2001, 42, 2357. CrossRef
59. M. Seki and K. Mori, Eur. J. Org. Chem., 2001, 3797. CrossRef
60. K. C. Nicolaou, J. Li, and G. Zenke, Helv. Chim. Acta, 2000, 83, 1977. CrossRef
61. H. Ohrui, Proc. Jpn. Acad. Ser. B, 2007, 83, 127. CrossRef
62. K. Mori, T. Tashiro, K. Akasaka, H. Ohrui, and E. Fattorusso, Tetrahedron Lett., 2002, 43, 3719. CrossRef
63. T. Tashiro, K. Akasaka, H. Ohrui, E. Fattorusso, and K. Mori, Eur. J. Org. Chem., 2002, 3659. CrossRef
64. M. Morita, K. Motoki, K. Akimoto, T. Natori, T. Sakai, E. Sawa, K. Yamaji, Y. Koezuka, E. Kobayashi, and H. Fukushima, J. Med. Chem., 1995, 38, 2176. CrossRef
65. H. Takikawa, S. Muto, and K. Mori, Tetrahedron, 1998, 54, 3141. CrossRef
66. T. Kawano, J. Cui, Y. Koezuka, I. Toura, Y. Kaneko, K. Motoki, H. Ueno, R. Nakagawa, H. Sato, E. Kondo, H. Koseki, and M. Taniguchi, Science, 1997, 278, 1626. CrossRef
67. N. Kamada, H. Iijima, K. Kimura, M. Harada, E. Shimizu, S. Motohashi, T. Kawano, H. Shinkai, T. Nakayama, T. Sakai, L. Brossay, M. Kronenberg, and M. Taniguchi, Int. Immunol., 2001, 13, 853. CrossRef
68. G. Yang, J. Schmieg, M. Tsuji, and R. W. Franck, Angew. Chem. Int. Ed., 2004, 43, 3818. CrossRef
69. K. Miyamoto, S. Miyake, and T. Yamamura, Nature, 2001, 413, 531. CrossRef
70. P. B. Savage, L. Teyton, and A. Bendelac, Chem. Soc. Rev., 2006, 35, 771. CrossRef
71. R. W. Franck and M. Tsuji, Acc. Chem. Res., 2006, 39, 692. CrossRef
72. D. Wu, M. Fujio, and C.-H. Wong, Bioorg. Med. Chem., 2008, 16, 1073. CrossRef
73. K. Fuhshuku, N. Hongo, T. Tashiro, Y. Masuda, R. Nakagawa, K. Seino, M. Taniguchi, and K. Mori, Bioorg. Med. Chem., 2008, 16, 950. CrossRef
74. F. K. Wallner, L. Chen, A. Moliner, M. Jondal, and M. Elofsson, ChemBioChem, 2004, 5, 437. CrossRef
75. D. Zhou, J. Mattner, C. Cantu III, N. Schrantz, N, Yin, Y. Gao, Y. Sagiv, K. Hudspeth, Y.-P. Wu, T. Yamashita, S. Tenenberg, D. Wang, R. L. Proia, S. B. Levery, P. B. Savage, L. Teyton, and A. Bendelac, Science, 2004, 306, 1786. CrossRef
76. T. Tashiro, N. Hongo, R. Nakagawa, K. Seino, H. Watarai, Y. Ishii, M. Taniguchi, and K. Mori, Bioorg. Med. Chem., 2008, 16, 8896. CrossRef
77. M. Koch, V. S. Stronge, D. Shepherd, S. D. Gadola, B. Mathew, G. Ritter, A. R. Fersht, G. S. Besra, R. R. Schmidt, E. Y. Jones, and V. Cerundolo, Nat. Immunol., 2005, 6, 819. CrossRef
78. N. A. Borg, K. S. Wun, L. Kjer-Nielsen, M. C. J. Wilce, D. G. Pellici, R. Koh, G. S. Besra, M. Bharadwaj, D. I. Godfrey, J. McClusky, and J. Rossjohn, Nature, 2007, 448, 44. CrossRef
79. T. Tashiro, R. Nakagawa, T. Hirokawa, S. Inoue, H. Watarai, M. Taniguchi, and K. Mori, Tetrahedron Lett., 2007, 48, 3343. CrossRef
80. T. Tashiro, R. Nakagawa, T. Hirokawa, S. Inoue, H. Watarai, M. Taniguchi, and K. Mori, Bioorg. Med. Chem., 2009, 17, 6360. CrossRef
81. T. Tashiro, R. Nakagawa, S. Inoue, M. Shiozaki, H. Watarai, M. Taniguchi, and K. Mori, Tetrahedron Lett., 2008, 49, 6827. CrossRef
82. S. Oki, A. Chiba, T. Yamamura, and S. Miyake, J. Clin. Invest., 2004, 113, 1631.
83. R. D. Goff, Y. Gao, J. Mattner, D. Zhou, N. Yin, C. Cantu, III, L. Teyton, A. Bendelac, and P. B. Savage, J. Am. Chem. Soc., 2004, 126, 13602. CrossRef
84. T. Toba, K. Murata, K. Nakanishi, B. Takahashi, N. Takemoto, M. Akabane, T. Nakatsuka, S. Imajo, T. Yamamura, S. Miyake, and H. Annoura, Bioorg. Med. Chem. Lett., 2007, 17, 2781. CrossRef
85. K. O. A. Yu, J. S. Im, A. Molano, Y. Dutronc, P. A. Illarionov, C. Forestier, N. Fujiwara, I. Arias, S. Miyake, T. Yamamura, Y.-T. Chang, G. S. Besra, and S. A. Porcelli, Proc. Natl. Acad. Sci. U.S.A., 2005, 102, 3383. CrossRef
86. D. J. Baek, Y.-S. Lee, C. Lim, D. Lee, T. Lee, J.-Y. Lee, K.-A. Lee, W.-J. Cho, C.-Y. Kang, and S. Kim, Chem. Asian J., 2010, 5, 1560. CrossRef
87. M. Fujio, D. Wu, R. Garcia-Navarro, D. D. Ho, M. Tsuji, and C.-H. Wong, J. Am. Chem. Soc., 2006, 128, 9022. CrossRef
88. Y.-J. Chang, J.-R. Huang, Y.-C. Tsai, J.-T. Hung, D. Wu, M. Fujio, C.-H. Wong, and A. L. Yu, Proc. Natl. Acad. Sci. U.S.A., 2007, 104, 10299. CrossRef
89. P.-H. Liang, M. Imamura, X. Li, D. Wu, M. Fujio, R. T. Guy, B.-C. Wu, M. Tsuji, and C.-H. Wong, J. Am. Chem. Soc., 2008, 130, 12348. CrossRef
90. A. Schiefner, M. Fujio, D. Wu, C.-H. Wong, and I. A. Wilson, J. Mol. Biol., 2009, 394, 71. CrossRef
91. B. A. Sullivan, N. A. Nagarajan, G. Wingender, J. Wang, I. Scott, M. Tsuji, R. W. Franck, S. A. Porcelli, D. M. Zajonc, and M. Kronenberg, J. Immunol., 2010, 184, 1141. CrossRef
92. J. Schmieg, G. Yang, R. W. Franck, and M. Tsuji, J. Exp. Med., 2003, 198, 1631. CrossRef
93. X. Li, G. Chen, R. Garcia-Navarro, R. W. Franck, and M. Tsuji, Immunology, 2008, 127, 216. CrossRef
94. R. T. Dere and X. Zhu, Org. Lett., 2008, 10, 4641. CrossRef
95. M. L. Blauvelt, M. Khalili, W. Jaung, J. Paulsen, A. C. Anderson, S. B. Wilson, and A. R. Howell, Bioorg. Med. Chem. Lett., 2008, 18, 6374. CrossRef
96. X. Lu, L. Song, L. S. Metelitsa, and R. Bittman, ChemBioChem, 2006, 7, 1750. CrossRef
97. G. Chen, J. Schmieg, M. Tsuji, and R. W. Franck, Org. Lett., 2004, 6, 4077. CrossRef
98. J.-J. Park, J. H. Lee, S. C. Ghosh, G. Bricard, M. M. Venkataswamy, S. A. Porcelli, and S.-K. Chung, Bioorg. Med. Chem. Lett., 2008, 18, 3906. CrossRef
99. M. Trappeniers, S. Goormans, K. Van Beneden, T. Decruy, B. Linclau, A. Al-Shamkhani, T. Elliott, C. Ottensmeier, J. M. Werner, D. Elewaut, and S. Van Calenbergh, ChemMedChem, 2008, 3, 1061. CrossRef
100. M. Trappeniers, R. Chofor, S. Aspeslagh, Y. Li, B. Linclau, D. M. Zajonc, D. Elewaut, and S. Van Calenbergh, Org. Lett., 2010, 12, 2928. CrossRef
101. R. M. Ndonye, D. P. Izmirian, M. F. Dunn, K. O. A. Yu, S. A. Porcelli, A. Khurana, M. Kronenberg, S. K. Richardson, and A. R. Howell, J. Org. Chem., 2005, 70, 10260. CrossRef
102. V. Lacône, J. Hunault, M. Pipelier, V. Bolt, T. Lecourt, J. Rocher, A.-L. Turcot-Dubois, S. Marionneau, J.-Y. Douillard, M. Clément, J. Le Pendu, M. Bonneville, L. Micouin, and D. Dubreuil, J. Med. Chem., 2009, 52, 4960. CrossRef
103. L. Leung, C. Tomassi, K. Van Beneden, T. Decruy, D. Elewaut, T. Elliott, A. Al-Shamkhani, C. Ottensmeier, S. Van Calenbergh, J. Werner, T. Williams, and B. Linclau, Org. Lett., 2008, 10, 4433. CrossRef
104. G.-T. Fan, Y. Pan, K.-C. Lu, Y.-P. Cheng, W.-C. Lin, S. Lin, C.-H. Lin, C.-H. Wong, J.-M. Fang, and C.-C. Lin, Tetrahedron, 2005, 61, 1855. CrossRef
105. M. Morita, T. Natori, K. Akimoto, T. Osawa, H. Fukushima, and Y. Koezuka, Bioorg. Med. Chem. Lett., 1995, 5, 699. CrossRef
106. K. Motoki, E. Kobayashi, T. Uchida, H. Fukushima, and Y. Koezuka, Bioorg. Med. Chem. Lett., 1995, 5, 705. CrossRef
107. T. Lee, M. Cho, S.-Y. Ko, H.-J. Youn, D. J. Baek, W.-J. Cho, C.-Y. Kang, and S. Kim, J. Med. Chem., 2007, 50, 585. CrossRef
108. M. Shiozaki, T. Tashiro, H. Koshino, R. Nakagawa, S. Inoue, T. Shigeura, H. Watarai, M. Taniguchi, and K. Mori, Carbohydr. Res., 2010, 345, 1663. CrossRef
109. L. Leung, C. Tomassi, K. Van Beneden, T. Decruy, M. Trappeniers, D. Elewaut, Y. Gao, T. Elliott, A. Al-Shamkhani, C. Ottensmeier, J. M. Werner, A. Williams, S. Van Calenbergh, and B. Linclau, ChemMedChem, 2009, 4, 329. CrossRef
110. T. Tashiro, K. Mori, M. Shiozaki, M. Taniguchi, and H. Watarai, JP2010-024859.
111. J.-J. Park, J. H. Lee, K.-C. Seo, G. Bricard, M. M. Venkataswamy, S. A. Porcelli, and S.-K. Chung, Bioorg. Med. Chem. Lett., 2010, 20, 814. CrossRef
112. K. Motoki, M. Morita, E. Kobayashi, T. Uchida, K. Akimoto, H. Fukushima, and Y. Koezuka, Biol. Pharm. Bull., 1995, 18, 1487.
113. E. Kobayashi, K. Motoki, Y. Yamaguchi, T. Uchida, H. Fukushima, and Y. Koezuka, Bioorg. Med. Chem., 1996, 4, 615. CrossRef
114. A. Uchimura, T. Shimizu, M. Nakajima, H. Ueno, K. Motoki, H. Fukushima, T. Natori, and Y. Koezuka, Bioorg. Med. Chem., 1997, 5, 1447. CrossRef
115. A. Uchimura, T. Shimizu, M. Morita, H. Ueno, K. Motoki, H. Fukushima, T. Natori, and Y. Koezuka, Bioorg. Med. Chem., 1997, 5, 2245. CrossRef
116. V. Costantino, E. Fattorusso, C. Imperatore, and A. Mangoni, Tetrahedron, 2002, 58, 369. CrossRef
117. D. Wu, G.-W. Xing, M. A. Poles, A. Horowitz, Y. Kinjo, B. Sullivan, V. Bodmer-Narkevitch, O. Plettenburg, M. Kronenberg, M. Tsuji, D. D. Ho, and C.-H. Wong, Proc. Natl. Acad. U.S.A., 2005, 102, 1351. CrossRef
118. L. Barbieri, V. Costantino, E. Fattorusso, A. Mangoni, N. Basilico, M. Mondani, and D. Taramelli, Eur. J. Org. Chem., 2005, 3279. CrossRef
119. L. Barbieri, V. Costantino, E. Fattorusso, A. Mangoni, E. Aru, S. Parapini, and D. Taramelli, Eur. J. Org. Chem., 2004, 468. CrossRef
120. R. Raju, B. F. Castillo, S. K. Richardson, M. Thakur, R. Severins, M. Kronenberg, and A. R. Howell, Bioorg. Med. Chem. Lett., 2009, 19, 4122. CrossRef
121. C. Xia, W. Zhang, Y. Zhang, W. Chen, J. Nadas, R. Severin, R. Woodward, B. Wang, X. Wang, M. Kronenberg, and P. G. Wang, ChemMedChem, 2009, 4, 1810. CrossRef
122. G.-W. Xing, D. Wu, M. A. Poles, A. Horowitz, M. Tsuji, D. D. Ho, and C.-H. Wong, Bioorg. Med. Chem., 2005, 13, 2907. CrossRef
123. L. Franchini, P. Matto, F. Ronchetti, L. Panza, L. Barbieri, V. Costantino, A. Mangoni, M. Cavallari, L. Mori, and G. De Libero, Bioorg. Med. Chem., 2007, 15, 5529. CrossRef
124. M. Trappeniers, K. Van Beneden, T. Decruy, U. Hillaert, B. Linclau, D. Elewaut, and S. Van Calenbergh, J. Am. Chem. Soc., 2008, 130, 16468. CrossRef
125. Y. Liu, R. D. Goff, D. Zhou, J. Mattner, B. A. Sullivan, A. Khurana, C. Cantu, III, E. V. Ravkov, C. C. Ibegbu, J. D. Altman, L. Teyton, A. Bendelac, and P. B. Savage, J. Immunol. Method, 2006, 312, 34. CrossRef
126. T. Tashiro, E. Sekine-Kondo, T. Shigeura, R. Nakagawa, S. Inoue, M. Omori-Miyake, T. Chiba, N. Hongo, S. Fujii, K. Shimizu, Y. Yoshiga, T. Sumida, K. Mori, H. Watarai, and M. Taniguchi, Int. Immunol., 2010, 22, 319. CrossRef
127. Y. Harrak, C. M. Barra, C. Bedia, A. Delgado, A. R. Castaño, and A. Llebaria, ChemMedChem, 2009, 4, 1608. CrossRef
128. B. G. Reddy, J. D. Silk, M. Salio, R. Balamurugan, D. Shepherd, G. Ritter, V. Cerundolo, and R. R. Schmidt, ChemMedChem, 2009, 4, 171. CrossRef
129. J. D. Silk, M. Salio, B. G. Reddy, D. Shepherd, U. Gileadi, J. Brown, S. H. Masri, P. Polzella, G. Ritter, G. S. Besra, E. Y. Jones, R. R. Schmidt, and V. Cerundolo, J. Immunol., 2008, 180, 6452.
130. S.-H. Park, K. Benlagha, D. Lee, E. Balish, and A. Bendelac, Eur. J. Immunol., 2000, 30, 620. CrossRef
131. D. M. Zajonc, P. B. Savage, A. Bendelac, I. A. Wilson, and L. Teyton, J. Mol. Biol., 2008, 377, 1104. CrossRef
132. S. Porubsky, A. O. Speak, B. Luckow, V. Cerundolo, F. M. Platt, and H.-J. Gröne, Proc. Natl. Acad. Sci. U.S.A., 2007, 104, 5977. CrossRef
133. D. I. Godfrey, D. G. Pellicci, and M. J. Smyth, Science, 2004, 306, 1687. CrossRef
134. D. M. Zajonc, G. D. Ainge, G. F. Painter, W. B. Severn, and I. A. Wilson, J. Immunol., 2006, 177, 4577.
135. Y. Kinjo, D. Wu, G. Kim, G.-W. Xing, M. A. Poles, D. D. Ho, M. Tsuji, K. Kawahara, C.-H. Wong, and M. Kronenberg, Nature, 2005, 434, 520. CrossRef
136. J. Mattner, K. L. DeBord, N. Ismail, R. D. Goff, C. Cantu, III, D. Zhou, P. Saint-Mezard, V. Wang, Y. Gao, N. Yin, K. Hoebe, O. Schneewind, D. Walker, B. Beutler, L. Teyton, P. B. Savage, and A. Bendelac, Nature, 2005, 434, 525. CrossRef
137. Y. Kinjo, E. Tupin, D. Wu, M. Fujio, R. Garcia-Navarro, M. R.-E.-I. Benhnia, D. M. Zajonc, G. Ben-Menachem, G. D. Ainge, G. F. Painter, A. Khurana, K. Hoebe, S. M. Behar, B. Beutler, I. A. Wilson, M. Tsuji, T. J. Sellati, C.-H. Wong, and M. Kronenberg, Nat. Immunol., 2006, 7, 978. CrossRef
138. D. M. Zajonc and M. Kronenberg, Immunol. Rev., 2009, 230, 188. CrossRef
139. K. Mori and T. Kinsho, Liebigs Ann. Chem., 1988, 807. CrossRef
140. K. Mori and K. Otaka, Tetrahedron Lett., 1994, 35, 9207. CrossRef
141. K. Otaka and K. Mori, Eur. J. Org. Chem., 1999, 1795. CrossRef
142. Y. Masuda, M. Yoshida, and K. Mori, Biosci. Biotechnol. Biochem., 2002, 66, 1531. CrossRef
143. E. J. Corey, B. Czakó, and L. Kürti, 'Molecules and Medicine,' John Wiley & Sons, Inc., Hoboken, N. J., 2007, p.126.