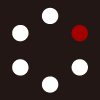
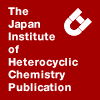
HETEROCYCLES
An International Journal for Reviews and Communications in Heterocyclic ChemistryWeb Edition ISSN: 1881-0942
Published online by The Japan Institute of Heterocyclic Chemistry
e-Journal
Full Text HTML
Received, 21st December, 2010, Accepted, 23rd March, 2011, Published online, 7th April, 2011.
DOI: 10.3987/REV-10-690
■ Air-Stable and High-Mobility Organic Semiconductors Based on Heteroarenes for Field-Effect Transistors
Shoji Shinamura, Itaru Osaka, Eigo Miyazaki, and Kazuo Takimiya*
Department of Applied Chemistry, Graduate School of Engineering, Hiroshima University, 1-4-1 Kagamiyama, Higashi-Hiroshima 739-8527, Japan
Abstract
Recent developments on heteroarene-based organic semiconductors applicable to high-performance, air-stable thin-film p-channel transistors are described. For the development of these new materials, including benzo[1,2-b:4,5-b']dichalcogenophenes (BDXs), [1]benzochalcogenopheno[3,2-b][1]benzochalcogenophenes (BXBXs), and dinaphtho[2,3-b:2',3'-f]chalcogenopheno[3,2-b]chalcogenophenes (DNXXs), new efficient synthetic methods are established. These materials are then evaluated as active layers in organic field-effect transistors (OFETs) fabricated by vacuum or solution processes. In the present work, molecular factors (molecular structures, energy levels and shapes of highest occupied molecular orbitals, molecular arrangements in the thin film) and the device performances are correlated and discussed to understand a structure-properties relationship. As a consequence of this approach, several air-stable and high-performance semiconductors for the OTFTs are successfully developed. For example, vapor-processable DNTT and solution-processable alkylated-BTBTs showing field-effect mobility as high as 3.0 cm2 V–1 s–1 and with 2.8 cm2 V–1 s–1, respectively, are among the best for recently developed new materials.CONTENTS
1. Introduction
2. Molecular Design Strategy for Improving Mobility: Plausibility of “Heavy Atom Effect” as a Working Hypothesis
3. Development of Benzo[1,2-b:4,5-b']dichalcogenophene (BDX)-Type Materials
4. [1]Benzochalchogenopheno[3,2-b][1]benzochalcogenophene (BXBX)-Type Materials
5. Dinaphtho[2,3-b:2',3'-f]chalcogenopheno[3,2-b]chalcogenophene (DNXX) -Type Materials
6. Conclusion
7. Acknowledgements
8. References and Notes
1. INTRODUCTION
The field of organic semiconductors has significantly been developed in the past two decades owing to the potential application to ultra-thin, large-area, and/or flexible devices in future generation consisting of, for instance, organic field-effect transistors (OFETs), organic light-emitting diodes (OLEDs), and organic photovoltaics (OPVs), that can be fabricated by low-cost and low-energy consumption processes, and thus offers great differentiation from the conventional silicon technologies. In particular, OFETs are an important fundamental component that would drive such devices.1
The origin of “organic semiconductors” can be date back to the 1950s, when the investigations on electrical conductivities of condensed aromatic hydrocarbons (i.e., arenes) such as perylene and pyrene were conducted by Inokuchi, Akamatsu, and co-workers.2 These studies were then extended into the field of versatile highly conducting organic materials based on charge-transfer complexes and radical ion salts, which at last produced fruitful scientific achievements of metallic organic materials and organic superconductors in the 1980s and the 1990s.3 On the other hand, as photo-induced current generation4 or electroluminescence5 in single crystals of neutral organic compounds have been long known since the 1900s, the organic compounds have been regarded as “semiconducting materials” with energy gaps. In fact, these phenomena are applied to OPVs and OLEDs actively investigated these days. In contrast to such an early discovery of photoconductivity and electroluminescence in organic compounds, it was the 1980s when the first application of their semiconducting nature to switching devices (i.e., transistors); use of poly(thiophene) in OFETs was reported.6 However, the properties of these prototypical OFETs including field-effect mobility and environmental stability were poor, and thus they were far from the practical use.
The great breakthroughs in the field of OFETs were witnessed in the late the 1990s.7 Several research groups including Bell Laboratory, United States, reported important progresses in terms of materials development, purity of and purification methods for organic semiconductors, and device fabrication techniques, which brought significant improvements in the device performances; mobilities as high as 1.5 cm2 V–1 s–1 became to be reproducibly achieved in pentacene-based OFETs.8 These remarkable achievements also impressed scientific and technology fields to recognize that OFETs can be a cheap alternative of the amorphous silicon thin-film transistors currently industrialized. Owing to its easy availability and good reproducibility in OFET performances, pentacene has become the standard organic semiconductor and widely utilized in various OFET studies; not only the device physics from the scientific interests but also fabrications of prototypical electronic devices such as flexible displays and sensors from industrial interests.9
From the viewpoint of material science and solid-state physics, the superiority in the transport properties of pentacene has also been seriously investigated. Carrier transport in the organic solid is often described with the hopping model. In this picture, high mobility, i.e., rapid exchange of carrier between molecules, can be realized by small reorganization energy (λ, energy consumption at the molecular level during carrier exchange) and large intermolecular orbital coupling (transfer integral, t).10 Pentance consisting of five benzene rings fused in a linear manner has a widely delocalized π-system with a rigid structure enabling smaller λ as well as larger t between neighboring molecules, which as a result affords the ideal molecular and intermolecular structure at the same time. In addition, pentacene takes the herringbone packing structure in the solid state that affords two-dimensional (2D) isotropic electronic structure on the substrates, which is also advantageous in the application of thin film transistors, because the devices require 2D isotropic electrical conduction for achieving high performances.11
Although pentacene is an ideal organic semiconductor for the application to OFETs as discussed above, its chemical instability and relatively high oxidation potential, i.e., high-lying HOMO energy level, that makes the molecule susceptible to air-oxidation, are the major drawbacks, which makes pentacene less practical in further applications (Figure 1).12 Moreover, such instability is general characteristics in higher oligoacenes, such as naphthacene and hexacene, the latter of which is not isolable as a pure form.13 This means that further improvements of device characteristics by extension of acene structure is not realistic.
2. MOLECULAR DESIGN STRATEGY FOR IMPROVING MOBILITY: PLAUSIBILITY OF “HEAVY ATOM EFFECT” AS A WORKING HYPOSESIS
With the background as discussed above, strategies for molecular designs enabling good stability and high performance simultaneously is eagerly desired. However, simple extension of π-conjugated system in the acene framework can not compromise both the performance and stability requirements, and therefore, new molecular design strategy that satisfies these two properties should be established.
To attain high mobility in organic materials, we focused on the enhancement of intermolecular interaction, in other words, strong intermolecular orbital coupling, by employing heteroaromatic compounds consisting of chalcogen atoms such as sulfur and selenium. These “heavy atoms” with large atomic radii can contribute to the effective intermolecular interactions in the solid state, and in fact, such effect has been proven to play an important role to construct the electrical conduction paths in the highly conducting organic charge-transfer salts and organic superconductors.3
We have thus started with verifying the concept of “heavy atom effect” in organic semiconductors by comparing the characteristics of oligothiophene- and oligoselenophene- based OFETs. Although we initially expected that the oligoselenophenes with larger selenium atoms than sulfur in the oligothiophenes show better FET characteristics including higher mobilities, no apparent difference in the mobility was found; for the tetramers and pentamers of both oligothiophene (1)/selenophene (2), the OFETs showed field-effect mobilites of 10–3 cm2 V–1 s–1 and 10–2 cm2 V–1 s–1, respectively (Figure 2).14
These somewhat disappointing experimental results can be rationalized by theoretical molecular orbital (MO) calculations. In the HOMO of oligochalcogenophenes, the nodal planes reside on the chalcogen atoms, in other words no coefficients of HOMOs on the chalcogen atoms (Figure 3a). In case of the p-channel OFETs, the hole should migrate through the HOMO of each molecule in the solid state, and thus no noticeable difference in the mobilities of oligochalcogenophenes is well explained. Furthermore, the existence of the nodal planes on chalcogen atoms with large atomic radii implies that effective intermolecular interaction of HOMOs is rather hindered by the “heavy atoms”. These considerations do not just rationalize the experimental results on the similar inferiority of oligothiophene/selenophenes, but do indicate importance of the geometry of the frontier molecular orbitals in designing organic semiconductors; the molecules with large HOMO coefficients on the chalcogen atoms should be promising p-channel organic semiconductors for OFET application.
With this new concept in mind, we carried out MO calculations of several chalcogenophene-containing molecules and found that a certain combination of chalcogenophenes and benzene rings in fused manners can afford desirable geometry of HOMO (Figure 3). Among such materials, benzo[1,2-b:4,5-b']dichalcogenophenes seemed to be particularly promising as the target molecules for p-channel organic semiconductors.15
3. DEVELOPMENT OF BENZO[1,2-B:4,5-B']DICHALCOGENOPHENE (BDX16)-TYPE MATERIALS
Although the parent benzo[1,2-b:4,5-b']dithiophene (BDT) have already been known,15,17 the reported synthetic methods of BDT required multi-step synthesis using two different thiophene derivatives as the starting materials, and thus were not practical for the large-scale preparation and for the synthesis of various BDT derivatives. It must also be difficult to apply them to the synthesis of benzo[1,2-b:4,5-b']diselenophene (BDS), the selenium analogue of BDT, because the corresponding starting selenophene derivatives are not practically available. We have thus pursued a new versatile synthetic strategy for benzo[1,2-b:4,5-b']dichalcogenophene (BDX) derivatives and found that the application of the one-pot cyclization of benzo[b]chalcogenophenes from o-bromoethynylbenene, developed by Sashida et al.,18 is quite useful as shown in Scheme 1.
Starting from easily available 1,4-dibromo-2,5-diiodobenzene (4), the Sonogashira coupling reaction with various acetylene derivatives followed by the double cyclization reaction on the benzene ring successfully afforded not only the parent but also a variety of BDT derivatives. Furthermore, BDS and benzo[1,2-b:4,5-b']ditellurophene (BDTe), the tellurium analogue, and their derivatives were also readily synthesizable using elemental selenium and tellurium instead of sulfur, respectively, at the cyclization step.19 In addition, diiodo-BDT and -BDS (9) can easily be synthesized from bis(trimethylsilyl)derivatives (8) by a treatment with iodine monochloride (ICl), which can act as the substrate in the palladium catalyzed cross-coupling reactions to afford various BDT- and BDS- derivatives (Scheme 2). For example, diaryl- and dialkyl- derivatives were smoothly obtained and then evaluated them as the active layer in OFETs.20,21
OFET devices using diphenyl -BDT and -BDS fabricated by vacuum deposition exhibited high field-effect mobilities of hole up to 0.2 cm2 V –1 s –1. Furthermore, alkylated BDT- and BDS-based devices fabricated by spin-coating showed typical p-channel characteristics with mobilities of around 0.01 cm2 V–1 s–1. More importantly, these devices did not show severe degradation of the performances under ambient conditions, indicating that the BDX derivatives are superior semiconducting materials compared to pentacene in terms of air-stability. The energy levels of HOMO of BDX derivatives estimated from their oxidation potentials are –5.5 ~ –5.3 eV below the vacuum level, and these relatively deep HOMO energy levels than that of pentacene apparently rationalize their good air-stability.
4. [1]BENZOCHALCOGENOPHENO[3,2-B][1]BENZOCHALCOGENOPHENE (BXBX)-TYPE MATERIALS
With the successful results on the BDX-based organic semiconductors, we then designed a new π-extended heteroaromatic system by substituting the benzene ring in the BDX with a naphthalene ring, which leads to naphtho[2,3-b:6,7-b']dichalcogenophene (NDX) framework consisting of two chalcogenophenes at the both ends of naphthalene. However, the synthesis of NDXs seemed to be very difficult, besides the selective synthesis of anti-NDXs without contamination of other isomers may also be difficult. In addition, according to the MO calculations, NDXs have higher HOMO and lower LUMO energy levels as compared to those of BDXs, suggesting that NDXs are unstable in air as is the case of polyacenes. For these reasons, rearrangement of the order of the chalcogenophene and benzene rings in NDXs was examined, and then [1]benzochalcogenopheno[3,2-b][1]benzochalcogenophene (BXBX) system was proposed as a new core for semiconducting materials.22 The BXBX framework, possessing two benzene rings at the end of chalcogenopheno[3,2-b]chalcogenophene, is a structural isomer of NDXs. Very interestingly, the energy levels of the frontier molecular orbital of BXBX derivatives estimated by the MO calculations are similar to those of BDX derivatives, suggesting good air-stability (Figure 4). In addition, BXBX derivatives are much more accessible than NDXs, because several synthetic routes to BXBX derivatives have been reported.22,23 For these reasons, we targeted the BXBX derivatives as the next promising organic semiconductors.
Among several synthetic routes to [1]benzothieno[3,2-b][1]benzothiophene (BTBT) derivatives so far reported, we selected the one using 2,7-diamino-BTBT (12), which is readily prepared from commercially available disodium 4,4'-dinitrostilbene-2,2'-disulfonate, as a key intermediate, because the intermediate seems to be versatile and practical to access to a wide range of BTBT derivatives. Using 12 prepared according to the reported procedure,23 the diazotation followed by a reaction with potassium iodide gave 2,7-diiodo-BTBT (13). The iodide was turned out to be truly useful to convert into not only 2,7-diphenyl-BTBT (DPh-BTBT, 14) via the Suzuki-Miyaura coupling reaction with phenyl boronic acid,24 but also 2,7-dialkyl-BTBT (Cn-BTBT, 15) via the Sonogashira coupling reaction with various alkynes followed by hydrogenation (Scheme 3).25
Although a synthetic route to the parent [1]benzoselenopheno[3,2-b][1]benzoselenophene (BSBS, 18) has already been reported by Sashida et al. (Scheme 4),22 the method does not seem to be suitable for the large-scale preparation (> 1 g), because it requires t-BuLi and expensive 1-bromo-2-iodobenzene (16) as the starting material (Scheme 4).
We therefore looked for a new method for the synthesis of BSBS. First, we tried the direct ortho-metalation of commercially available diphenylacetylene (19) with the LICKOR superbase (n-BuLi/t-BuOK) followed by a treatment with selenium powder. Although this reaction did not afford BSBS directly, the bis(selenolate) anion in situ generated was successfully trapped with methyl iodide, giving bis(methylseleno)diphenylacetylene (20) in 68% yield (Scheme 5).26 Then, the isolated intermediate was cyclized with excess iodine into BSBS in 90% yield. With the same synthetic protocol, bis(biphenyl-4-yl)acetylene can be converted into 2,7-diphenyl-BSBS (DPh-BSBS, 21).26
On the contrary, the synthesis of alkylated BSBS with the synthetic method in Scheme 5 was not successful; ortho-lithiation of 4,4'-dialkyl diphenylacetylene with the LICKOR superbase did not take place. We then tried direct bromination of BSBS at 2- and 7- positions, and it turned out that moderately selective bromination takes place by carefully controlling the reaction conditions, giving 2,7-dibromo-BSBS (29) in 40% isolated yield after recrystallization. As in the case for diiodo-BTBT, the dibrominated BSBS is a versatile intermediate for the synthesis of various BSBS derivatives including 2,7-dialkyl- and 2,7-diphenyl-BSBSs, and thus we needed to have the intermediate in a good amount for further development of BSBS-based organic semiconductors. For this reason, a new scalable synthetic route to BSBS was necessary, and after intensive synthetic studies, we finally found that the synthesis using 2-methylselenobenzaldehyde (26), which was easily prepared from methyl anthranilate (22) in 4 steps, as the key intermediate successfully provided BSBS in a good total yield and amount.27 In this synthesis, 26 was first dimerized into trans-2,2'-bis(methylseleno)stilbene (27) via the low-valent titanium-mediated olefination, and then demethylation-ring closing reaction with iodine gave the parent BSBS. After the bromination of BSBS, the Sonogashira coupling reaction with various acetylenes gave bis(alkynyl)-BSBS derivatives, which were then readily converted into the alkylated BSBSs (Cn-BSBSs, 30) via hydrogenation in the presence of Pd/C. DPh-BSBS was also easily obtained via the Suzuki-Miyaura coupling of 29 with phenyl boronic acid (Scheme 6).27
To examine the BXBX derivatives as organic semiconductors, we fabricated and evaluated their FET devices. Physical vapor deposition of the diphenyl derivatives (DPh-BTBT and DPh-BSBS) gave homogeneous thin films on Si/SiO2 substrates, and the devices based on their thin films showed typical FET behaviors under ambient conditions with mobilities of 2.0 and 0.3 cm2 V–1 s–1 for DPh-BTBT and DPh-BSBS, respectively.24,26 Among these, the very high mobilites of DPh-BTBT-based devices, almost comparable to that of the best pentacene OFET, are notable. Furthermore, extremely good air-stability and durability of DPh-BTBT-based OFETs were confirmed by shelf-life test under ambient lab conditions more than a half year, indicating that DPh-BTBT is one of the best materials among organic semiconductors applicable to thin-film OFETs.
On the other hand, alkylated BXBXs (Cn-BXBXs) in general have high solubility in various organic solvents and gave homogeneous thin films on the Si/SiO2 substrates by solution deposition processes such as spin-coating. FET devices based on Cn-BXBXs with a top contact configuration (W/L = 30) showed typical p-channel FET responses under ambient conditions. Cn-BTBTs with long alkyl groups (longer than n-octyl group) exhibited very high mobilities exceeding 0.5 cm2 V–1 s–1, and, in particular, C13-BTBT showed the best performances with the maximum mobility of 2.8 cm2 V–1 s–1.25 To our best knowledge, these markedly high mobilities are one of the highest values among the solution processed thin-film OFETs so far reported. In contrast, the field-effect mobilities of Cn-BSBS-based devices were at most 0.03 cm2 V–1 s–1, which is lower than those of Cn-BTBT-based devices by two-orders of magnitude.27
To clarify the difference of these device performances, we investigated the molecular arrangement by using thin-film X-ray diffraction (XRD) measurements and X-ray single crystal structure analyses. Both Cn-BTBT and Cn-BSBS thin films showed crystalline peaks in the XRD patterns indicating the formation of the lamellar structures, in which the molecular long axes are aligned parallel to the surface normal on the substrate. However, the molecular arrangements of Cn-BTBT and Cn-BSBS within the lamellar layer are different. For the Cn-BTBT thin films, the diffraction peaks are indexed with the bulk single crystal cell, evidencing that the solid-state structures in the both phases are the same. This indicates that the herringbone molecular arrangements that ensure the 2D electronic structure suitable for the effective carrier transport have grown on the substrate surface in the Cn-BTBT thin-film.28 On the contrary, the molecular arrangement in the Cn-BSBS thin-film was apparently different from that in the bulk single crystal phase, judged from the comparison between the thin-film XRDs and the simulated powder patterns from the bulk single crystal structures. Although the exact molecular arrangement in the thin film phase is not completely clear, the ideal 2D-herringbone structure that observed in the Cn-BTBT thin films is not realized in the Cn-BSBS thin-film, which likely rationalizes the lower performance of the Cn-BSBS-based OFETs.27
More interestingly, the single crystal X-ray structure analyses of a series of Cn-BTBTs (n = 8, 10, 12) revealed that the lattice size in the lamella layer, i.e., the crystallographic ab cell, becomes smaller as the alkyl chain elongates. This means that the BTBT core is densely packed as a result of intermolecular van der Waals interaction enhanced by the longer alkyl chains, that is, “molecular fastener effect”, contributing the improved transistor performances (Figure 5).29
These results demonstrate that the introduction of long alkyl groups at the end of the π-conjugated core acts not only as solubilizing groups but also as functional groups that render the π-conjugated core pack tightly and thus enhance the mobility. Note that the concept of “molecular fastener effect” can be recognized as a new strategy for improving performances of OFETs, in particular, solution processable molecular organic semiconductors.30
5. DINAPHTHO[2,3-B:2',3'-F]CHALCOGENOPHENO[3,2-B]CHALCOGENOPHENE (DNXX) -TYPE MATERIALS
As described above, the BXBX derivatives have low-lying HOMO energy levels and thus are fairly stable in air despite their π-extended structure with four aromatic/heteroaromatic rings fused in a liner manner. This is in sharp contrast to the oligoacene system with a similar molecular geometry such as tetracene and pentacene. To clarify the difference between these two classes, we shed light on their electronic structures. Since chalcogenophene has the aromatic sextet structure similar to benzene, one might regard BXBXs as to be isoelectronic with tetracene, where four benzene rings fuse in a liner manner. However, taking into account the fact that the chalcogenophene’s aromatic sextet is completed by two electrons provided from the chalcogen atom, the isoelectronic hydrocarbon of BXBX should not be tetracene but chrysene, one of the phene-type aromatic hydrocarbons. In general, increase of the number of fused benzene rings in the phene-type aromatics does not drastically destabilize the HOMO and stabilize the LUMO energy levels (Figure 6). This is in sharp contrast to the tendency observed in the acene-type aromatics (Figure 1).
Hence, the low-lying HOMO energy level of BXBX derivatives and thus good air-stability can be understood with their phene-like electronic structures.31
From these considerations, it should be possible to extend the π-conjugated system with ensuring air-stability, or keeping low-lying HOMO energy levels. In fact, the MO calculations predict that dinaphtho[2,3-b:2',3'-f]thieno[3,2-b]thiophene (DNTT), where two benzene rings are fused to the BTBT core would possess sufficiently low-lying HOMO energy level and thus high air-stability, which prompted us to target this promising material. By applying the above-mentioned synthetic strategy for the parent BSBS (Scheme 6), we successfully established the synthesis of DNTT and its selenium analogue (DNSS). Commercially available 2-naphthaldehyde (31) was selectively lithiated at the 3-position using the ortho-directing lithiation procedure with lithium N,N,N'-trimethylethylenediamide and excess n-BuLi, followed by a treatment with dimethyldisulfide or selenium powder and methyl iodide gave 3-methylthio-2-naphthaldehyde (32) or 3-methylseleno-2-naphthaldehyde (33), respectively. Although the isomeric 1-methylthio(seleno)naphthaldehydes were also produced as byproducts, they were readily removed by column chromatography. Then, 32 and 33 were dimerized into the olefinic precursors via the low-valent titanium-mediated McMurry coupling, which was finally treated with excess iodine to undergo the ring-closing reaction, forming DNTT (36) and DNSS (37) in good yields (Scheme 7).32,33
DNTT and DNSS are air-stable compounds as expected, and their thin-film based OFETs fabricated by vacuum deposition process showed excellent mobilities reproducibly (3.0 cm2V-1s-1 as DNTT and 2.0 cm2V-1s-1 as DNSS).32 In particular, the DNTT-based devices showed negligible degradation over long period of time and hysteresis in the transfer characteristics. These FET characteristics are comparable to or even better than those of pentacene. Because of its high performances and stability, we believe that DNTT will be a new standard organic semiconductor in the next generation.34
6. CONCLUSION
We have designed and synthesized a number of new organic semiconductors based on π-extended heteroarene compounds with high mobility and stability. Importantly, it has been proven that the theoretical molecular orbital calculations and X-ray structural analyses are useful tools to understand the nature of materials’ charge transport properties and thus to design new materials with even better performances. The device properties including charge carrier mobility and stability can be well correlated with the electronic structures, which are apparently consistent with the molecular orbital calculations, and the crystal structures. Furthermore, the use of “molecular fastener effect” that promotes strong intermolecular interactions has been found to greatly improve the molecular ordering of the materials in the thin film, resulting in high mobilities. We have also developed several facile and practical synthetic methodologies to access a variety of heteroarene-based materials, which can be adapted to the synthesis of other new materials,35 not only molecular semiconductors but also polymer semiconductors.36 Needless to say, precise purification of the materials and optimization of the device fabrication are also crucial for the detailed structure–property relationship studies. We believe that these important findings together with the developed materials will pave the way to overcome the difficulties on commercializing the organic electronic devices that have yet to be achieved with the conventional materials.
7. ACKNOWLEDGEMENTS
The authors express their sincere thanks to Professor Yoshihito Kunugi (Tokai University), Professor Jun Takeya (Osaka University), and Dr. Tatusya Yamamoto for cooperative research. The authors are also deeply indebted to our colleagues who energetically studied the present subjects in Hiroshima University and have their names in the references.
References
1. ‘Organic Electronics: Materials, Manufacturing’, ed. by H. Klauk, Wiley-VCH, Weinheim, 2006; ‘Organic Field-Effect Transistors’, ed. by Z. Bao and J. Locklin, CRC press, Boca Raton, 2007.
2. H. Akamatu, H. Inokuchi, and Y. Matsunaga, Nature, 1954, 173, 168. CrossRef
3. T. Ishigura, K. Yamaji, and G. Saito, “Organic Superconductors”, Springer, Hidelberg, 1998.
4. A. Pocchettino, Acad. Lincei Rendiconti, 1906, 15, 355.
5. W. Helfrich and W. G. Schneider, Phys. Rev. Lett., 1965, 14, 229. CrossRef
6. A. Tsumura, H. Koezuka, and T. Ando, Appl. Phys. Lett., 1986, 49, 1210. CrossRef
7. H. E. Katz, J. Mater. Chem., 1997, 7, 369. CrossRef
8. Y. Y. Lin, D. J. Gundlach, S. F. Nelson, and T. N. Jackson, IEEE Electron Device Lett., 1997, 606; CrossRef T. W. Kelley, L. D. Boardman, T. D. Dunbar, D. V. Muyres, M. J. Pellerite, and T. P. Smith, J. Phys. Chem. B, 2003, 107, 5877; CrossRef H. Klauk, M. Halik, U. Zschieschang, G. Schmid, W. Radlik, and W. Weber, J. Appl. Phys., 2002, 92, 5259. CrossRef
9. I. Yagi, N. Hirai, M. Noda, A. Imaoka, Y. Miyamoto, N. Yoneya, K. Nomoto, J. Kasahara, A. Yumoto, and T. Urabe, SID ’07 Digest, 2007, 1753; T. Sekitani, M. Takamiya, Y. Noguchi, S. Nakano, Y. Kato, T. Sakurai, and T. Someya, Nat Mater, 2007, 6, 41; CrossRef T. Sekitani, H. Nakajima, H. Maeda, T. Fukushima, T. Aida, K. Hata, and T. Someya, Nat Mater, 2009, 8, 494; CrossRef G. Gelinck, P. Heremans, K. Nomoto and T. D. Anthopoulos, Adv. Mater., 2010, 22, 3778; CrossRef T. Sekitani, U. Zschieschang, H. Klauk, and T. Someya, Nat. Mater., 2010, 9, 1015; CrossRef T. Sekitani and T. Someya, Adv. Mater., 2010, 22, 2228. CrossRef
10. J.-L. Brédas, D. Beljonne, V. Coropceanu, and J. Cornil, Chem. Rev., 2004, 104, 4971; CrossRef V. Coropceanu, J. Cornil, D. A. da Silva Filho, Y. Olivier, R. Silbey, and J.-L. Brédas, Chem. Rev., 2007, 107, 926. CrossRef
11. H. Yoshida and N. Sato, Phys. Rev. B., 2008, 77, 235205. CrossRef
12. A. Maliakal, K. Raghavachari, H. Katz, E. Chandross, and T. Siegrist, Chem. Mater., 2004, 16, 4980. CrossRef
13. R. Mondal, R. M. Adhikari, B. K. Shah, and D. C. Neckers, Org. Lett., 2007, 9, 2505. CrossRef
14. Y. Kunugi, K. Takimiya, K. Yamane, K. Yamashita, Y. Aso, and T. Otsubo, Chem. Mater., 2003, 15, 6. CrossRef
15. BDT derivatives were utilized as a core structure for organic semiconductors for OFET applicaiotns; see, G. L. Laquindanum, H. E. Katz, A. J. Lovinger, and A. Dodabalapur, Adv. Mater., 1997, 9, 36; CrossRef H. E. Katz, Z. Bao, and S. L. Gilat, Acc. Chem. Res., 2001, 34, 359. CrossRef
16. Since the compound with fused ring system described in the present paper have generally long formal names, they are conveniently abbreviated by using their acronym, e.g., BDT for BenzoDiThiophene or BDS for BenzoDiSelenophene. In the same manner, general chalcogenophene system, e.g. BenzoDiCharcogenophenes (BDX), are abbreviated by using “X”, which well corresponds to the structural drawings.
17. P. Beimling and G. Koβmehl, Chem. Ber., 1986, 119, 3198; CrossRef S. Yoshida, M. Fujii, Y. Aso, T. Otsubo, and F. Ogura, J. Org. Chem., 1994, 59, 3077. CrossRef
18. H. Sashida, K. Sadamori, and T. Tsuchiya, Synth. Commun., 1998, 28, 713. CrossRef
19. K. Takimiya, Y. Kunugi, Y. Konda, N. Niihara, and T. Otsubo, J. Am. Chem. Soc., 2004, 126, 5084; CrossRef K. Takimiya, Y. Konda, H. Ebata, N. Niihara, and T. Otsubo, J. Org. Chem., 2005, 70, 10569. CrossRef
20. K. Takimiya, Y. Kunugi, and H. Ebata, T. Otsubo. Chem. Lett., 2006, 35, 1200. CrossRef
21. T. Kashiki, E. Miyazaki, and K. Takimiya, Chem. Lett., 2008, 37, 284; CrossRef S. Shinamura, T. Kashiki, T. Izawa, E. Miyazaki, and K. Takimiya, Chem. Lett., 2009, 38, 35. CrossRef
22. H. Sashida and S.Yasuike, J. Heterocycl. Chem., 1988, 35, 725. CrossRef
23. S. Y. Zherdeva, A. Barudi, A. Y. Zheltov, and B. I. Stepanov, Zh. Organi. Khimi., 1980, 16, 430.
24. K. Takimiya, H. Ebata, K. Sakamoto, T. Izawa, T. Otsubo, and Y. Kunugi, J. Am. Chem. Soc., 2006, 128, 12604. CrossRef
25. H. Ebata, T. Izawa, E. Miyazaki, K. Takimiya, M. Ikeda, H. Kuwabara, and T. Yui, J. Am. Chem. Soc., 2007, 129, 15732. CrossRef
26. K. Takimiya, Y. Kunugi, Y. Konda, H. Ebata, Y. Toyoshima, and T. Otsubo, J. Am. Chem. Soc., 2006, 127, 3044. CrossRef
27. T. Izawa, E. Miyazaki, and K. Takimiya, Chem. Mater., 2009, 21, 903. CrossRef
28. T. Izawa, E. Miyazaki, and K. Takimiya, Adv. Mater., 2008, 20, 3388. CrossRef
29. H. Inokuchi, K. Imaeda, T. Enoki, T. Mori, Y. Maruyama, G. Saito, N. Okada, H. Yamochi, K. Seki, Y. Higuchi, and N. Yasuoka, Nature, 1987, 329, 39. CrossRef
30. Among alkylated BTBTs, C8-BTBT have been widely used in the fabrication of printed transistors; see: M. Uno, Y. Tominari, and J. Takeya, Appl. Phys. Lett., 2008, 93, 173301; CrossRef T. Minari, M. Kano, T. Miyadera, S.-D. Wang, Y. Aoyagi, and K. Tsukagoshi, Appl. Phys. Lett., 2009, 94, 093307; CrossRef T. Uemura, Y. Hirose, M. Uno, K. Takimiya, and J. Takeya, Appl. Phys. Express, 2009, 2, 111501; CrossRef T. Endo, T. Nagase, T. Kobayashi, K. Takimiya, M. Ikeda, and H. Naito, Appl. Phys. Express, 2010, 3, 121601; CrossRef C. Liu, T. Minari, X. Lu, A. Kumatani, K. Takimiya, and K. Tsukagoshi, Adv. Mater., 2011, 23, 523. CrossRef
31. K. Takimiya, T. Yamamoto, H. Ebata, and T. Izawa, Sci. Tech. Adv. Mater., 2007, 8, 273. CrossRef
32. T. Yamamoto and K. Takimiya, J. Am. Chem. Soc., 2007, 129, 2224; CrossRef T. Yamamoto and K. Takimiya, J. Photopolymer Sci. Tech., 2007, 20, 57; CrossRef T. Yamamoto, S. Shinamura, E. Miyazaki, and K. Takimiya, Bull. Chem. Soc. Jpn., 2010, 83, 120. CrossRef
33. Using the same synthetic method, several DNTT deriavatives were also reported; see, M. J. Kang, T. Yamamoto, S. Shinamura, E. Miyazaki, and K. Takimiya, Chem. Sci., 2010, 1, 179; CrossRef M. J. Kang, I. Doi, H. Mori, E. Miyazaki, K. Takimiya, M. Ikeda, and H. Kuwabara, Adv. Mater., 2011, 23, 1222; CrossRef K. Nakayama, Y. Hirose, J. Soeda, M. Yoshizumi, T. Uemura, M. Uno, W. Li, M. J. Kang, M. Yamagishi, Y. Okada, E. Miyazaki, Y. Nakazawa, A. Nakao, K. Takimiya, and J. Takeya, Adv. Mater., 2011, 23, 1626.. CrossRef
34. Various applications of DNTT as the active material for OFETs have been recently published; S. Haas, Y. Takahashi, K. Takimiya, and T. Hasegawa, Appl. Phys. Lett., 2009, 95, 022111; CrossRef M. Uno, I. Doi, K. Takimiya, and J. Takeya, Appl. Phys. Lett., 2009, 94, 103307; CrossRef M. Uno, Y. Tominari, M. Yamagishi, I. Doi, E. Miyazaki, K. Takimiya, and J. Takeya, Appl. Phys. Lett., 2009, 94, 223308; CrossRef M. A. McCarthy, B. Liu, and A. G. Rinzler, Nano Lett., 2010, 10, 3467; CrossRef M. Uno, Y. Hirose, T. Uemura, K. Takimiya, Y. Nakazawa, and J. Takeya, Appl. Phys. Lett., 2010, 97, 013301; CrossRef U. Zschieschang, F. Ante, T. Yamamoto, K. Takimiya, H. Kuwabara, M. Ikeda, T. Sekitani, T. Someya, K. Kern, and H. Klauk, Adv. Mater., 2010, 22, 982; CrossRef U. Zschieschang, T. Yamamoto, K. Takimiya, H. Kuwabara, M. Ikeda, T. Sekitani, T. Someya, and H. Klauk, Adv. Mater., 2011, 23, 644. CrossRef
35. Very recently, another facile methods for the syntheses of BDXs, BTBT, DNTT, and related heteroarens were also reported; see, T. Kashiki, S. Shinamura, M. Kohara, E. Miyazaki, K. Takimiya, M. Ikeda, and H. Kuwabara, Org. Lett., 2009, 11, 2473; CrossRef S. Shinamura, E. Miyazaki, and K. Takimiya, J. Org. Chem., 2010, 75, 1228; CrossRef M. Saito, T. Yamamoto, I. Osaka, E. Miyazaki, K. Takimiya, H. Kuwabara, and M. Ikeda, Tetrahedron Lett., 2010, 51, 5277; CrossRef M. Saito, I. Osaka, E. Miyazaki, K. Takimiya, H. Kuwabara, and M. Ikeda, Tetrahedron Lett., 2011, 52, 285; CrossRef S. Shinamura, I. Osaka, E. Miyazaki, A. Nakano, M. Yamagishi, J. Takeya, and K. Takimiya, J. Am. Chem. Soc., 2011, 133, 5024. CrossRef
36. I. Osaka, T. Abe, S. Shinamura, E. Miyazaki, and K. Takimiya, J. Am. Chem. Soc., 2010, 132, 5000; CrossRef I. Osaka, T. Abe, S. Shinamura, and K. Takimiya, J. Am. Chem. Soc., 2011, 2011, 133, 6852. CrossRef
37. T. Siegrist, C. Kloc, R. A. Laudise, H. E. Katz, and R. C. Haddon, Adv. Mater., 1998, 10, 379. CrossRef
38. H. Nakanishi, S. Inoue, Y. Aso, and T. Otsubo, Synth. Met., 1999, 101, 639. CrossRef