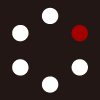
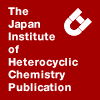
HETEROCYCLES
An International Journal for Reviews and Communications in Heterocyclic ChemistryWeb Edition ISSN: 1881-0942
Published online by The Japan Institute of Heterocyclic Chemistry
e-Journal
Full Text HTML
Received, 22nd June, 2011, Accepted, 17th August, 2011, Published online, 29th August, 2011.
DOI: 10.3987/COM-11-S(P)47
■ An Improved Synthesis of Functionalized cis-Decahydroquinolines Using a Baylis-Hillman-Type Adduct
Junfeng Huang, Jeffrey Petersen, and Stephen C. Bergmeier*
Department of Chemistry and Biochemistry, Ohio University, Clippinger Laboratories, Athens, OH 45701-2979, U.S.A.
Abstract
An efficient and diastereoselective method to synthesize highly functionalized cis-decahydroquinolines was reported. The addition of a vinyl aluminum reagent to an aldehyde followed by an intramolecular SN2’ Mitsunobu reaction gave the desired 3,7,8-trisubstituted product designed as methyllycaconitine analogs. An unexpected tandem vinylaluminum addition and Michael reaction to produce a 4-hydroxy cis-decahydroquinoline has also been reported.The cis-decahydroquinoline moiety is found in a variety of pharmacologically interesting molecules. These include CB2 agonists1 and ligands for the vesicular acetylcholine transporter.2 A variety of natural products including the dendrobatid alkaloids3 (e.g. cis-195A),4 the lepadin family of alkaloids,5-7 and myrionine8 also contain the cis-decahydroquinoline ring system. Our interest in this ring system stems from its presence in the skeleton of the norditerpenoid alkaloid methyllycaconitine (MLA, Figure 1),9,10 a potent and selective antagonist of the nicotinic acetylcholine receptor.11,12 In our approach to synthesize small molecule analogs of methyllycaconitine and to probe the subtype selectivity of nAChRs, we and others have prepared a number of simple compounds which only contain the E ring and the succinimidoylanthranilate ester of methyllycaconitine (1).13-18 These compounds showed low affinity for α7 nAChRs and have no affinity for α3β4* nAChRs; however, they appear to act as noncompetitive inhibitors of α3β4* nAChRs.19,20 In an effort to continue to define the structure activity relationship of MLA, we wished to provide additional conformational restriction to our previously reported ring E analogs, and address additional MLA-like non-covalent interactions. Simply disjoining rings A, C, D and F from this hexacyclic norditerpenoid alkaloid provides the BE-ring of MLA (2) as a 3,7,8-trisubstituted cis-decahydroquinoline derivative (3).21,22
We had previously reported a synthesis of the requisite 3,7,8-trisubstituted cis-decahydroquinoline ring system using a Knoevenagel condensation as the key step.22 This synthesis was problematic in terms of compound throughput, having several steps with low yields or providing mixtures of diastereomers. We report herein an improved method for the synthesis of the 3,7,8-trisubstituted cis-decahydroquinoline ring system using a Baylis-Hillman type coupling as the key step. As shown in Scheme 1, the proposed plan is to start with the known aldehyde 6 and add an acrylate unit via a Baylis-Hillman reaction to provide 5. A subsequent intramolecular SN2′ reaction would then yield the cis-fused ring system (4) with two differentially reactive olefins. A dihydroxylation followed by a hydrogenation would provide the requisite ring system (3) with the new stereocenters controlled by the cis-ring fusion.
As outlined in Table 1, the reaction of aldehyde 622,23 with methyl acrylate under typical Baylis-Hillman reaction conditions proved to be highly unsatisfactory. The first method examined used DABCO as the Lewis base.24 This reaction was quite slow and after two weeks the reaction was stopped and the product determined to be diasteromers 5 and 7 in a 1:1 ratio. Modification of the conditions25,26 (entries 2 and 3) did not yield an improvement of either yield or diastereoselectivity. Other Lewis bases such as DBU,27 Ph3P,28 and DMAP,29 (entries 4 – 6) were also examined but did not provide better results.
Given the poor yields using traditional Baylis-Hillman reaction conditions, an alternative method was sought to carry out the necessary transformation. Tsuda and coworkers reported that the anionic intermediate of β-unsubstituted [α-(alkoxycarbonyl)-vinyl]aluminum (8), formed by the hydroalumination of α,β-acetylenic esters with DIBAL-H in the presence of HMPA, reacted with aldehydes at room temperature to produce the Baylis-Hillman adduct.30 Replacment of the carcinogenic HMPA with NMO has more recently been shown to provide improved yields.31 Application of these reaction conditions to aldehyde 6, resulted in product (5) being obtained in 71% yield as a single diastereomer (Scheme 2). The relative stereochemistry of the hydroxyl group was determined via X-ray analysis (Figure 2).32 It seems likely that a chelation controled addition of the vinyl aluminum reagent to the aldehyde is responsible for the excellent stereocontrol. The relatively rigid cyclohexene linking the aldehyde and the carbamate nitrogen would be expected to enhance the stereoselectivity.
Interestingly, when the reaction was allowed to proceed at room temperature for 24 h under the conditions described in Scheme 2, we obtained a mixture of compound 5 and an unknown compound in a ratio of 1:4. After carefully characterizing the unknown compound, we found it was an unexpected 4-hydroxy cis-decahydroquinoline product 9. The observation of an NOE interaction between H3 and H10 was used to determine the relative stereochemistry. While unexpected, the isolation of 9 as the major product is significant in that it demonstrated that the decahydroquinoline ring system could be formed in a single step. We found that the yield of 9 could be improved to 75% by carrying out the reaction in THF and replacing NMO with HMPA as the alumination ligand (Scheme 3). The stereoselectivity of the reaction can be rationalized by an examination of the cyclization product. A chelate between the alkoxide and the ester enolate provides a very rigid convex molecule. Protonation of the enolate would be expected to occur only from the top face of the molecule providing 9 as the sole product.
With the allylic alcohol 5 in hand, construction of the bicyclic compound 4 could proceed. It was anticipated that an intramolecular SN2′ reaction could be effected using Mitsunobu conditions.33-37 Standard Mitsunobu conditions however provided DEAD-adduct 10 (Entry 1, Table 2) as the sole product. The possibility that this product was formed as a result of competition between an inter- and intramolecular reaction was confirmed by decreasing the concentration. Reducing the concentration to 0.05 M (entry 2) provided a mixture of the desired product 4 and the undesired DEAD-adduct, 10. In their synthesis of medium-sized cyclic amines, the Fukuyama group demonstrated that both highly dilute solutions and less polar solvents were best for an intramolecular SN2 reaction.38 We thus maintained the concentration of our reaction system at 0.05 M and decreased the polarity of solvent system by using the non-polar solvent toluene. This provided only the product 4 in a low yield (entry 3). THF was then added to enhance the reactivity. An optimum toluene:THF ratio was determined to be 3:1 which provided the product 4 in 70% yield with only a 10% yield of the DEAD-adduct also isolated (entry 5). More THF led to greater amounts of 10, while less provided increasing amounts of starting material.
Chemoselective reaction of the two double bonds was carried out via an initial dihydroxylation of 4 to generate the corresponding diol 11. As expected, this dihydroxylation occured from the top or convex face of the bicyclic molecule.22 The diol appeared to polymerize upon isolation, thus, it was directly converted to acetonide to provide 12 in an 85% yield from 4 (Scheme 4).
The next step was to stereospecifically reduce the remaining double bond. It was anticipated that reduction would occur from the top or convex face of the bicyclic molecule as previously shown.22 The reduction conditions were also expected to concomitantly remove the carbobenzyloxy protecting group. Initial attempts at the reduction lead to slow reaction rates and mixtures of products where either the Cbz or double bond had been reduced. The reaction was modified by changing the solvent to 20% AcOH in EtOH. This provided a clean reduction of the double bond, removal of the Cbz group and solvolysis of the acetonide. While this compound was quite stable, it was quite polar and very difficult to purify. An N-alkylation of the crude hydrogenation product provided 13 as a more readily purifiable product in 65% yield from 12.
In summary, we developed an efficient (6 chemical steps, 4 isolated intermediates) and highly stereoselective method to synthesize functionalized cis-decahydroquinolines via a Baylis-Hillman type intermediate 5. The traditional Baylis-Hillman reaction was not successful in our system, however the addition of a vinylaluminum reagent to aldehyde 6 provided access to this intermediate. An intramolecular SN2′ Mitsunobu reaction of compound 5 gave a bicyclic system, which has not been previously reported. By using different reaction conditions, the vinylaluminum reaction also stereospecificlly afforded a tandem addition and Michael reaction, which produced a 4-hydroxy cis-decahydroquinoline system 9. These azabicyclic ring systems can be used for the synthesis of BE-ring analogs of methyllycaconitine. This work is underway and will be reported in due course.
EXPERIMENTAL
All reagents used were purchased from commercial sources or prepared according to standard literature methods using references given in the text and purified as necessary prior to use by standard literature procedures. THF and CH2Cl2 were dried using a Solv-Tek solvent purification system. Thin-layer chromatography was performed on precoated silica gel aluminum plates and visualized by UV-absorbance, staining with 5% phosphomolybdic acid (PMA) in ethanol, or 1.5% ninhydrin in HOAc-EtOH (3:100) solution. Column chromatography was performed using silica gel 60A. Proton (1H) and carbon (13C) magnetic resonance spectra (NMR) were recorded on a Bruker 300 MHz Fourier transform spectrometer, and chemical shift are expressed in parts per million (δ) relative to tetramethylsilane (TMS, 0 ppm) as internal reference. The relative stereochemistry of all compounds is denoted using the R* or S* notation.39
Methyl 2-((S*)-((1S*,2R*)-2-(((Benzyloxy)carbonyl)amino)cyclohex-3-en-1-yl)(hydroxy)methyl)-acrylate (5). DIBAL-H (16.5 mL of a 1.0 M solution in hexane, 16.5 mmol) was added to a solution of NMO (3.9 g, 33.2 mmol) in CH2Cl2 (40 mL) at 0 °C over 15 min. The reaction was stirred for 0.5 h. Methyl propiolate (1.6 mL, 17.9 mmol) was added and the reaction stirred for an additional 1 h at 0 °C, followed by the addition of a solution of compound 6 (4.2 g, 16.2 mmol) in THF (15 mL). The reaction was stirred for 5 h, and carefully quenched with 1.0 M HCl at 0 °C. The mixture was extracted with Et2O, washed with saturated aq. NaHCO3, brine, dried over MgSO4, filtered, concentrated, and chromatographed (20% EtOAc in hexanes) to provide 3.98 g (71%) of 5 as a colorless oil, Rf 0.5 (45% EtOAc in hexanes); 1H NMR (CDCl3, 300 MHz) δ 7.58 (m, 5H), 6.28 (s, 1H), 5.68 (m, 3H), 5.35 (m, 1H), 5.08 (bs, 2H), 4.53 (s, 1H), 4.21 (bs, 1H), 3.72 (s, 3H), 3.01 (bs, 1H), 2.02 (m, 5H), 1.40 (m, 1H); 13C NMR (CDCl3, 70 MHz) δ 166.9 (C), 156.1 (C), 140.7 (C), 136.6 (C), 132.5 (CH), 128.6 (CH), 128.4 (CH), 128.3 (CH), 128.2 (CH), 127.3 (CH), 126.6 (CH2), 72.3 (CH), 66.8 (CH2), 51.8 (CH3), 47.6 (CH), 41.6 (CH), 25.5 (CH2), 17.8 (CH2); HRMS (ESI) C19H23NO5 m/z calculated M + H+ 346.1655, measured M + H+ 346.1645 (2.7 ppm); Anal. Calcd for C19H23NO5: C, 66.07; H, 6.71; N, 4.06. Found C, 66.28; H, 6.68; N, 4.00.
(4S*,4aS*,8aR*)-1-Benzyl 3-methyl 4-hydroxy-4,4a,5,6-tetrahydroquinoline-1,3(8aH)-dicarboxylate (9). DIBAL-H (8.5 mL of a 1.0 M solution in hexane, 8.5 mmol) was added to a solution of HMPA (2.2 mL, 12.7 mmol) in THF (40 mL) at 0 °C over 15 min. The reaction was stirred for 0.5 h, methyl propiolate (0.8 mL, 8.9 mmol) was added and the reaction stirred for another 1 h at 0 °C. A solution of compound 6 (2.1 g, 8.0 mmol) in THF (15 mL) was added, the ice bath removed, and the reaction warmed to 40 °C for 5 h. The reaction was cooled to 0 °C quenched with 1.0 M HCl and extracted with Et2O. The combined organic layer was washed with saturated aq. NaHCO3, brine, dried over MgSO4, filtered, concentrated, and chromatographed (30% EtOAc in hexanes) to provide 2.1 g (75%) of 9 as a white solid, Rf 0.5 (45% EtOAc in hexanes); 1H NMR (CDCl3, 300 MHz) δ 7.38 (m, 5H), 5.95 (bs, 1H), 5.64 (bs, 1H), 5.20 (m, 2H), 4.87 (bs, 1H), 4.36 (m, 2H), 3.73 (s, 3H), 3.25 (bt, J = 11.2 Hz, 1H), 2.65 (m, 3H), 2.05 (m, 4H); 13C NMR (CDCl3, 70 MHz) δ 172.2 (C), 155.5 (C), 136.6 (C), 132.6 (CH), 129.2 (CH), 128.5 (CH), 128.4 (CH), 128.0 (CH), 127.9 (CH), 71.4 (CH), 67.4 (CH2), 51.9 (CH3), 49.1 (CH), 47.4 (CH), 44.7 (CH), 35.2 (CH2), 35.2 (CH), 25.1 (CH), 22.8 (CH); HRMS (ESI) C19H23NO5 m/z calculated M + H+ 346.1655, measured M + H+ 346.1656 (0.3 ppm); Anal. Calcd for C19H23NO5: C, 66.07; H, 6.71; N, 4.06. Found C, 66.03; H, 6.82; N, 4.11.
(4aR*,8aR*)-1-Benzyl 3-methyl 4a,5,6,8a-tetrahydroquinoline-1,3(2H)-dicarboxylate (4). PPh3 (795 mg, 3.0 mmol) was added to a solution of compound 5 (520 mg, 1.51 mmol) in toluene-THF (3:1, 30 mL). The reaction was then cooled to -40 °C, followed by the addition of DEAD (0.47 mL, 3.0 mmol). The reaction was slowly warmed to room temperature and stirred for and additional 6 h. The reaction was quenched with saturated aq. NaHCO3 and extracted with EtOAc. The combined organic layers were washed with brine, dried over MgSO4, filtered, concentrated, and chromatographed (15% EtOAc in hexanes) to provide 350 mg of 4 (70%) as colorless oil. Rf 0.7 (20% EtOAc in hexanes); 1H NMR (CDCl3, 300 MHz) δ 7.40 (m, 5H), 6.80 (bs, 1H), 5.81 (m, 1H), 5.32 (m, 1H), 5.20 (bs, 2H), 5.08 (m, 1H), 4.60 (m, 1H), 3.77 (s, 3H), 3.70 (bs, 1H), 2.85 (bs, 1H), 1.92 (m, 4H); 13C NMR (CDCl3, 70 MHz) δ 165.6 (C), 155.5 (C), 143.7 (CH), 136.6 (C), 131.2 (CH), 128.5 (CH), 128.4 (CH), 128.3 (CH), 128.1 (CH), 127.9 (CH), 126.4 (CH), 67.4 (CH2), 51.8 (CH3), 48.3 (CH), 38.9 (CH2), 32.9 (CH), 26.0 (CH2), 20.6 (CH2); HRMS (ESI) C19H21NO4 m/z calculated M + H+ 328.1548, measured M + H+ 328.1557 (2.4 ppm); Anal. Calcd for C19H21NO4: C, 69.71; H, 6.47; N, 4.28. Found C, 69.90; H, 6.59; N, 3.98.
(3aS*,5aR*,9aS*,9bR*)-9-Benzyl 7-methyl 2,2-dimethyl-3a,4,5,5a,9a,9b-hexahydro-[1,3]dioxolo[4,5-h]quinoline-7,9(8H)-dicarboxylate (12). NMO (215 mg, 1.84 mmol) was added to a solution of compound 4 (300 mg, 0.92 mmol) in acetone-H2O (3 : 1, 20 mL). After stirring for 10 min at 0 °C, a solution of OsO4 (2.5% in H2O, 0.4 mL, 0.04 mmol) was added and the reaction stirred for an additional 8 h at room temperature. A solution of Na2S2O4 (100 mg) in water (5 mL) was added to the reaction and stirred for 20 min. The reaction was extracted with EtOAc (3 x 20 mL). The combined organic layers were washed with brine, dried over MgSO4, filtered, and concentrated, to provide 300 mg of crude diol 11. The crude diol 11 was immediately added to a solution of 2,2-dimethoxypropane:acetone (2:1, 15 mL), followed by the addition of TsOH•H2O (8 mg, 0.04 mmol) and MgSO4 (1.0 g). The reaction was stirred for 6 h at room temperature, filtered, concentrated and chromatographed (20% EtOAc in hexanes) to provide 314 mg (85%) of 12 as a colorless oil, Rf 0.5 (20% EtOAc in hexanes); 1H NMR (CDCl3, 300 MHz) δ 7.38 (m, 5H), 6.86 (bs, 1H), 5.20 (m, 2H), 4.70 (m, 1.5H), 4.37 (m, 0.5H), 4.23 (bs, 1H), 3.90 (m, 2H), 3.77 (s, 3H), 2.75 (bs, 1H), 2.02 (m, 2H), 1.66 (bs, 3H), 1.32 (m, 5H); 13C NMR (CDCl3, 70 MHz) δ 165.1 (C), 155.4 (C), 140.7 (CH), 136.5 (C), 128.5 (CH), 128.4 (CH), 128.3 (CH), 128.1 (CH), 128.0 (CH), 109.1 (C), 72.3 (CH), 67.5 (CH2), 51.8 (CH3), 39.3 (CH2), 34.5 (CH), 28.0 (CH3), 26.5 (CH3), 23.2 (CH2), 21.1 (CH2); HRMS (ESI) C22H27NO6 m/z calculated M + H+ 402.1917, measured M + H+ 402.1936 (4.8 ppm); Anal. Calcd for C22H27NO6: C, 65.82; H, 6.78; N, 3.49. Found C, 65.60; H, 7.10; N, 3.50.
(3S*,4aR*,7S*,8R*,8aS*)-Methyl 1-ethyl-7,8-dihydroxydecahydroquinoline-3-carboxylate (13). Compound 12 (400 mg, 1.0 mmol) was dissolved in a solution of HOAc (4 mL) in 95% EtOH (16 mL). The reaction was flushed with Ar, then 10% Pd/C (106 mg) was added. The Ar was replaced with H2, and the reaction stirred for 4 h under an atmosphere of H2 (balloon). The reaction was filtered through a celite pad, washed with MeOH, and concentrated. The crude material (225 mg) was immediately dissolved in acetone (10 mL), then iodoethane (0.95 mmol, 1.2 mmol) was added, followed by the addition of K2CO3 (550 mg, 4 mmol). The mixture was stirred for 6 h at room temperature, filtered, concentrated, and chromatographed (10% MeOH in CH2Cl2) to provide 154 mg (65%) of 13 as a colorless oil, Rf 0.1 (10% MeOH in CH2Cl2) 1H NMR (CDCl3, 300 MHz) δ 4.20 (q, J = 2.9 Hz, 1H), 3.83 (dd, J = 10.6, 2.9 Hz, 1H), 3.69 (s, 3H), 2.93 (m, 4H), 2.75 (m, 3H), 2.02 (m, 2H), 1.81 (m, 2H), 1.65 (m, 2H), 1.34 (m, 2H), 1.11 (t, J = 7.1, 3H); 13C NMR (CDCl3, 70 MHz) δ 174.9 (C), 68.3 (CH), 65.9 (CH), 58.1 (CH), 51.8 (CH3), 47.6 (CH2), 46.5 (CH2), 36.7 (CH), 27.9 (CH2), 27.7 (CH), 24.9 (CH2), 24.2 (CH2), 14.5 (CH3); HRMS (ESI) C13H23NO4 m/z calculated M + H+ 258.1705, measured M + H+ 258.1712 (2.7 ppm); Anal. Calcd for C13H23NO4: C, 60.68; H, 9.01; N, 5.44. Found C, 60.34; H, 9.31; N, 5.31.
ACKNOWLEDGEMENTS
This work was supported in part by grant DA13939 from the National Institutes on Drug Abuse at the National Institutes of Health. We would also like to acknowledge the support of Ohio University through the funding of the BioMolecular Innovation and Technology project.
References
1. P. J. Manley, A. Zartman, D. V. Paone, C. S. Burgey, D. A. Henze, K. Della Penna, R. Desai, M. D. Leitl, W. Lemaire, R. B. White, S. Yeh, M. O. Urban, S. A. Kane, G. D. Hartman, M. T. Bilodeau, and B. W. Trotter, Bioorg. Med. Chem. Lett., 2011, 21, 2359. CrossRef
2. S. M. N. Efange, A. B. Khare, R. H. Mach, and S. M. Parsons, J. Med. Chem., 1999, 42, 2862. CrossRef
3. J. W. Daly, T. F. Spande, and H. M. Garraffo, J. Nat. Prod., 2005, 68, 1556. CrossRef
4. J. W. Daly, T. Tokuyama, G. Habermehl, I. Karle, and B. Witkop, Liebigs Ann. Chem., 1969, 729, 198. CrossRef
5. J. Kubanek, D. E. Williams, E. D. d. Silva, T. Allen, and R. J. Anderson, Tetrahedron Lett., 1995, 36, 6189. CrossRef
6. R. A. Davis, A. R. Carroll, and R. J. Quinn, J. Nat. Prod., 2002, 65, 454. CrossRef
7. A. D. Wright, E. Goclik, G. M. König, and R. Kaminsky, J. Med. Chem., 2002, 45, 3067. CrossRef
8. V. C. Pham, A. Jossang, A. Chiaroni, T. Sévenet, V. H. Nguyen, and B. Bodo, Org. Lett., 2007, 9, 3531. CrossRef
9. R. H. Manske, Can. J. Res., 1938, 16B, 57. CrossRef
10. J. A. Goodson, J. Chem. Soc., 1943, 139. CrossRef
11. D. R. E. Macallan, G. G. Lunt, S. Wonnacott, K. L. Swanson, H. Rapoport, and E. X. Albuquerque, FEBS Lett., 1988, 226, 357. CrossRef
12. J. M. Ward, V. B. Cockcroft, G. G. Lunt, F. S. Smillie, and S. Wonnacott, FEBS Lett., 1990, 270, 45. CrossRef
13. S. C. Bergmeier, K. A. Ismail, K. M. Arason, S. McKay, D. L. Bryant, and D. B. McKay, Bioorg. Med. Chem. Lett., 2004, 14, 3739. CrossRef
14. S. C. Bergmeier, D. J. Lapinsky, R. B. Free, and D. B. McKay, Bioorg. Med. Chem. Lett., 1999, 9, 2263. CrossRef
15. D. L. Bryant, R. B. Free, S. M. Thomasy, D. J. Lapinsky, K. A. Ismail, K. M. Arason, S. C. Bergmeier, and D. B. McKay, Ann. N. Y. Acad. Sci., 2002, 971, 139. CrossRef
16. D. L. Bryant, R. B. Free, S. M. Thomasy, D. J. Lapinsky, K. A. Ismail, S. B. McKay, S. C. Bergmeier, and D. B. McKay, Neurosci. Res., 2002, 42, 57. CrossRef
17. J. Huang, C. M. Orac, S. McKay, D. L. Bryant, D. B. McKay, and S. C. Bergmeier, Bioorg. Med. Chem., 2008, 16, 3816. CrossRef
18. Y. Chan, J. Balle, J. K. Sparrow, P. D. W. Boyd, M. A. Brimble, and D. Barker, Tetrahedron, 2010, 66, 7179. CrossRef
19. T. F. González-Cestari, B. J. Henderson, R. E. Pavlovicz, S. B. McKay, R. A. El-Hajj, A. B. Pulipaka, C. M. Orac, D. D. Reed, R. T. Boyd, M. X. Zhu, C. Li, S. C. Bergmeier, and D. B. McKay, J. Pharmacol. Exp. Ther., 2009, 328, 504.
20. D. B. McKay, C. Chang, T. F. Gonzalez-Cestari, S. B. McKay, R. A. El-Hajj, D. L. Bryant, M. X. Zhu, P. W. Swaan, K. M. Arason, A. B. Pulipaka, C. M. Orac, and S. C. Bergmeier, Mol. Pharmacol., 2007, 71, 1288. CrossRef
21. H. Guthmann, D. Conole, E. Wright, K. Koerber, D. Barker, and M. A. Brimble, Eur. J. Org. Chem., 2009, 1944. CrossRef
22. J. Huang and S. C. Bergmeier, Tetrahedron, 2008, 68, 6434. CrossRef
23. P. Wipf and X. Wang, Tetrahedron Lett., 2000, 41, 8747. CrossRef
24. E. Ciganek, Org. React., 2004, 51, 201.
25. V. K. Aggarwal, D. K. Dean, A. Mereu, and R. Williams, J. Org. Chem., 2002, 67, 510. CrossRef
26. S. Rafel and J. W. Leahy, J. Org. Chem., 1997, 62, 1521. CrossRef
27. V. K. Aggarwal and A. Mereu, Chem. Commun., 1999, 2311. CrossRef
28. M. Shi and Y.-M. Xu, Eur. J. Org. Chem., 2002, 696. CrossRef
29. M. Shi, C.-Q. Li, and J.-K. Jiang, Tetrahedron, 2003, 59, 1181. CrossRef
30. T. Tsuda, T. Yoshida, and T. Saegusa, J. Org. Chem., 1988, 53, 1037. CrossRef
31. P. V. Ramachandran, M. T. Rudd, T. E. Burghardt, and M. V. R. Reddy, J. Org. Chem., 2003, 68, 9310. CrossRef
32. CCDC 838224 contains the supplementary crystallographic data for this paper (compound 5). These data can be obtained free of charge from The Cambridge Crystallographic Data Centre via http://www.ccdc.cam.ac.uk/data_request/cif.
33. B. H. Lipshutz, D. W. Chung, B. Rich, and R. Corral, Org. Lett., 2006, 8, 5069. CrossRef
34. Y. Otsuka, F. Inagaki, and C. Mukai, J. Org. Chem., 2010, 75, 3420. CrossRef
35. M. A. Zancanella and D. Romo, Org. Lett., 2008, 10, 3685. CrossRef
36. A. Fujiwara, T. Kan, and T. Fukuyama, Synlett, 2000, 1667. CrossRef
37. N. Langlois and O. Calvez, Tetrahedron Lett., 2000, 41, 8285. CrossRef
38. T. Kan and H. Kobayashi, Synlett, 2002, 697. CrossRef
39. E. L. Eliel, S. H. Wilen, and L. N. Mander In Stereochemistry of Organic Compounds; Wiley: New York, NY, 1994, p. 1260.