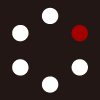
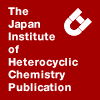
HETEROCYCLES
An International Journal for Reviews and Communications in Heterocyclic ChemistryWeb Edition ISSN: 1881-0942
Published online by The Japan Institute of Heterocyclic Chemistry
e-Journal
Full Text HTML
Received, 4th June, 2011, Accepted, 14th July, 2011, Published online, 25th July, 2011.
DOI: 10.3987/REV-11-SR(P)1
■ Recent Advances on the Synthesis of Piperidines through Ruthenium-Catalyzed Ring-Closing Metathesis (RCM) Reactions
Pedro Merino,* Tomas Tejero, Graziella Greco, Eduardo Marca, Ignacio Delso, Asier Gómez-SanJuan, and Rosa Matute
Department of Organic Chemistry, University of Zaragoza, E-50009 Zaragoza, Aragon, Spain
Abstract
Ring-closing metathesis reactions of dialkenyl amines, amides and carbamates catalyzed by Ru-catalysts provide efficient approaches to six-membered nitrogen heterocyclic compounds, which are precursors of a variety of natural products and related compounds.CONTENTS
1. Introduction
2. Synthesis of single-ring tetrahydropiperidines
2.1 Synthesis of N-carbamoyl tetrahydropyridines
2.2 Synthesis of N-benzyl tetrahydropyridines
2.3 Synthesis of spiro derivatives
2.4 Synthesis of dihydropyridin-2(3H)-ones
3. Synthesis of bicyclic compounds
4. Concluding remarks
Acknowledgments
References
1. INTRODUCTION
The presentation of Nobel Prize to Yves Chauvin, Robert H. Grubbs and Richard R. Schrock “for the development of the metathesis method”, recognized the influence and power of the metathesis reactions in Organic Synthesis.1 Several stable, functional group tolerant and commercially available catalysts have been reported for metathesis reactions,2 the most common utilized being Ru-based catalysts 1-5 although a portfolio of modified catalysts3-5 is now available from several chemical suppliers.
These catalysts have been successfully employed in many instances for the construction of small molecules and total syntheses of natural products.6,7A variety of metathesis reactions are known,8-10 including ring-closing, ring-opening, ring-expansion, domino, enyne,11,12 polymerization13 and cross-metatheses.14-17 From these reactions ring-closing metathesis (RCM) reactions have gained a prominent position in the past decades as a useful class of reactions for constructing both carbo- and heterocycles.18 Due to the extreme importance of RCM reactions and related processes there have been several reviews on this topic to date.19-25
The metathesis reactions have a wide functional groups tolerance, although in some instance the presence of a basic nitrogen or a primary amine can affect the yield of the reaction.26,27 Because of this reason the metathesis reactions with nitrogenated compounds have been studied in detail28 and, in particular, RCM reactions of nitrogen-containing compounds directed to the synthesis of alkaloids, peptidomimetics and other compounds have been recently considered.29 In this respect, an excellent compilation of approaches for synthesizing pyrrolidine and piperidine natural alkaloids using RCM reactions as a key step has been reported in 2003 by Lebreton and Felpin.30
This review focuses on recent developments of RCM reactions on nitrogen-containing substrates leading to the synthesis of nitrogenated six-membered rings of synthetic and biological importance and covers the literature from January 2003 to April 2011.
2. SYNTHESIS OF SINGLE-RING TETRAHYDROPIPERIDINES
2.1. SYNTHESIS OF N-CARBAMOYL TETRAHYDROPIPERIDINES
The piperidine alkaloid (+)-sedamine has been prepared from N-Boc protected dialkenylamine 6 using RCM as a key reaction. The metathesis reaction was promoted by Grubbs I catalyst 1 (20 mol%) and took place in good chemical yield at 50 °C for 2 h (Scheme 1).31
The reaction showed a good compatibility with acid-sensitive protecting groups and it could be carried out at room temperature, although 24 h were needed for completing the reaction.32 The reaction conditions have shown to be tolerant with free hydroxyl groups33 allowing an expeditious synthesis of cis-4-hydroxypipecolic acid (Scheme 2).34 In order to achieve a good conversion it was needed to add the catalyst again (3 mol%) after the first 4 h of reaction. A similar approach was used for preparing 3-hydroxypipecolic acid.35
In general, N-Boc dialkenylamines afforded excellent results in RCM reactions catalyzed by 1. Depending on the substrates the reaction can be carried out in different solvents at several temperatures. The ligand core of (-)-sparteine has been constructed by RCM of a N-Boc dialkenylamine in dichloromethane at room temprature, the product being obtained in essentially quantitative yield.36
By carrying out the reaction in dichloromethane at room temperature for 10 h the intermediate precursor of (-)-β-conhydrine was obtained from compound 10 (Scheme 3).37,38 This alkaloid has also been prepared by other routes involving RCM reactions of N-Boc dialkenylamines catalyzed by 1. In all cases a high compatibility with hydroxyl protecting groups including acetals,39 silyl40 and benzyl ethers,41 and acetonide groups was observed.41 In the latter case, the approach was extended to the preparation of indolizidine (-)-lentiginosine.
Enantiomeric (+)-β-conhydrine was also synthetized from the enantiomer of 11 by using the same reaction conditions illustrated in Scheme 3.41 By using this strategy and under similar reaction conditions, a general methodology for preparing 2-substituted-3-hydroxypiperidines was developed.42 RCM reactions of hydroxylated N-Boc dialkenylamines has been successfully employed in the preparation of azasugars such as 1-deoxygulonojirimycin 14 (Scheme 4).43
Catalyst 1 was also employed with aromatic N-Boc alkenylamines such as 15. By using 10 mol% of catalyst, tricyclic derivative 16 was obtained in good chemical yield (Scheme 5).44 In the case of extended dienes 15 (n= 2, 3, 4) only dimerization products 17 were obtained instead the corresponding expanded ring. The same results were observed with Grubbs II catalyst 2.
In some instance side reactions affording isomeric enamines have been observed. RCM of 18 catalyzed by 1 afforded a 60:40 mixture of compounds 19 and 20. This ratio was changed to 85:15 by using Grubbs II 2 as a catalyst under the same reaction conditions (Scheme 6).45
Catalyst 2 has been successfully employed in RCM reactions of fluorinated dialkenyl N-Boc amines46 and in the synthesis of the alkaloid (+)-lentiginosine from 21 (Scheme 7).47 In both cases the reaction was carried out with 10 mol% of catalyst in dichloromethane at reflux.
Alternatively, catalyst 3 led also to good results quite similar to those observed with catalyst 1, with N-Boc dialkenyl derivatives in dichloromethane (or dichloroethane) at reflux.48 By using 1 mol% of 3 and a chiral auxiliary, enantiomerically pure N-Boc tetrahydropyridines 24 were readily prepared in high chemical yields and enantioselectivities (Scheme 8).49
The methodology illustrated in Scheme 8 was applied to the synthesis of the alkaloid (+)-lentiginosine.50 Substrates bearing nucleohilic amine functions such as 25 are also amenable of being used as substrates in RCM reactions by employing Hoveyda-Grubbs II catalyst 5 (Scheme 9).51 Compound 26 is a precursor of the potent neurokinin P receptor antagonist (+)-(2S,3S)-CP-99,994. The same strategy was used for preparing epimeric (-)-(2S,3R)-CP-99,994.52
Catalyst 5 was also employed with N-Boc dialkenyl substituted substrates in combination with a N-H insertion reaction. The resulting tandem protocol was used for the preparation of saturated nitrogen heterocycles.53
In a similar way to N-Boc protected substrates, dialkenylamines protected as N-Cbz carbamates underwent RCM reaction with Grubbs catalysts 1 and 2. Compounds 27 and 29 afforded the corresponding tetrahydropyridines 28 and 30, respectively in excellent chemical yields upon treatment with 10 mol% of 1 in dichloromethane at room temperature (Scheme 10).54 The reaction was also extended to the formation of 5- and 7-membered nitrogen heterocycles and further used in the synthesis of iminosugars. The reaction proceeded smoothly with substituted alkenes and in the presence of a siloxygroup. In this case, however, reflux was needed for completing the reaction.38
By using similar strategies to those illustrated with N-Boc carbamates, 3-hydroxypipecolic acids have been accessed from N-Cbz dialkenyl amines employing catalyst 1.55 When the reaction was carried out with unsusbtituted alkenes dichloromethane was used as a solvent at room temperature.56A versatile enantioselective synthesis of the marine alkaloids barrenazines was accomplished through RCM of 31 catalyzed by 1 as a key step (Scheme 11).57
The formal synthesis (-)-haouamine A was achieved from intermediate 34 readily obtained from precursor 33 through RCM catalyzed by 2 and in situ deprotection and oxidation (Scheme 12).58
The presence of a third alkene in the molecule led to both five- and six-membered products as in the case of 35 which on treatment with 2.5 mol% of 2 afforded a 43:57 mixture of pyrroline 36 and tetrahydropyridine 37. In contrast, alkenyl amide 38 only cyclized, under the same reaction conditions, to the corresponding lactam 39 (Scheme 13).59
The RCM of dialkenyl N-methoxycarbonyl derivative 40 took place in 85% yield with catalyst 2 (Scheme 14).60 Interestingly, the reaction was carried out in the presence of a free carboxylic acid and it was applied to a substituted substrate even though lower yield was observed in that case.61 Dialkylidene urea derivatives were also used as substrates in RCM reactions with 1 as a catalyst.62
N-Tosyl dialkenyl amine 42 afforded the corresponding tetrahydropyridine 43 upon treatment with catalyst 1 in dichloromethane at room temperature.63 The same reaction was achieved with compound 44 showing a good compatibility with the more labile N,O-acetonide group (Scheme 15).64
2.2. SYNTHESIS OF N-BENZYL TETRAHYDROPYRIDINES
The above mentioned RCM reactions with carbamates, leading to similar results,65 involved protection of the nitrogen atom in such a way that it cannot act as a nucleophile; nevertheless it was possible to carry out RCM reactions in the presence of a dibenzylamino group51,52 and N-benzyl dialkenyl amine 46 underwent RCM in the presence of catalyst 2 (Scheme 16).66 Notably, the reaction took place in the presence of unprotected hydroxyl groups. The procedure was also extended to the formation of pyrrolines.
RCM reactions of N-benzyl dialkenylamines have also been reported employing 1 as catalyst in refluxing benzene with sugar-derived substrates en route to trihydroxy-quinolizidine analogues.67
2.3. SYNTHESIS OF SPIRO TETRAHYDROPYRIDINES
Of particular interest is the application of the above discussed reactions in the synthesis of spiro derivatives which are difficult to prepare by other routes. RCM reaction of cyclohexyl derivative 48 afforded spirocompound 49 in excellent yield (Scheme 17).68
Several oxo-indolespirocyclic derivatives that resulted to be potent inhibitors of HIV protease have been prepared through RCM catalyzed by 1.69 Spirocyclic reverse turn mimics were accessed through a higly practical RCM strategy based on the use of catalyst 2 and solid-phase synthesis techniques.70 Spiroketal related natural products were synthesized through a ketal-tethered RCM strategy. Among those products spiroaminal51 was obtained from 50 through RCM reaction promoted by 1.71
RCM reaction of 52 catalyzed by 2 and in the presence of p-TsOH afforded a 3:1 mixture of spirocycle 53 and epimer 54, probably as a consequence of the high acidity of the C-2 proton (Scheme 19).72 This methodology was also applied to larger heterocyclic rings. RCM reactions catalyzed by 2 in the presence of p-TsOH have been reported for preparing not only tetrahydropyridines but also pyrrolines and larger rings.73
Spirocyclic compounds related to the bioactive natural products histrionicotoxins were synthesized using RCM as a key step. As an example, compound 56 was obtained from trifluoroacetamide 55 by treatment with 1 in dichloromethane at room temperature. In spite of the presence of a NBn group the reaction took place in quantitative yield (Scheme 20).74
2.4. SYNTHESIS OF DIHYDROPYRIDIN-2(3H)-ONES
N-Alkenyl acrylamides have been used as suitable starting materials for obtaining six-membered lactams through RCM reactions. Treatment of 57 with catalytic 2 in dichloromethane at room temperature afforded unsaturated lactam 58 in good yield (Scheme 21).75
With lower catalyst loading (4-5 mol%) reflux was necessary76 but good yields were obtained not only for tetrahydropyridines but also for five- and seven-membered rings.73 Similar results were obtained with Grubbs I catalyst 177 and N-(2,4-dimethoxybenzyl) derivatives.78 On the other hand, very similar reactions has also been described at room temperature by using 7.5 mol%60 and 2.7 mol%59 of catalyst 2.
Some differences in reactivity have been observed between Grubbs’ catalysts 1 and 2. While alkenyl acrylamide 59 cyclized readily in the presence of 2 at room temperature, with 1 as a catalyst the reaction did not work as well and 45% yield of 60 was obtained after several hours at reflux.79
The reaction has also been carried out with substituted alkenes but 24 h at reflux were needed for completing the reaction (Scheme 23). Lactam 62 served as an immediate precursor for the synthesis of (-)-CP-99,994 and (-)-L-733,060, two piperidine analogues with high affinity and selectivity with human NKI receptor.80
A series of six-membered lactams have been prepared by RCM reaction catalyzed with 2 in excellent yields. The obtained compounds were used in the preparation of novel bridged bicyclic lactams showing various degrees of pyramidalization of the bridgehead nitrogen atom (Scheme 24).81
N-Phenylacrylamide 65 underwent RCM reaction in the presence of 2 (10 mol%) to provide 66 in excellent yield (Scheme 25). Interestingly, the catalyst loading could be reduced to 5 mol% and 2 mol% without loss of efficiency although longer reaction times were needed.82
When the reaction was carried out with substituted alkenes good results were obtained by using 5 mol% of catalyst with the exception of substrates bearing either bulky substituents in the double bond directly linked to the aromatic ring or halogenated substituents at the acrylamide moiety.82
In the case of N-phenylethylenesulfonamides an important solvent effect was observed, whereas no reaction was observed in benzene, good yields were obtained for the reaction carried out in dichloromethane (Scheme 26).82
Two RCM reactions have been used in a reaction sequence leading to (+)-epiquinamide starting with dialkenyl amide 69, which furnished N-benzyl-1,6-dihydropyridin-2(3H)-one 70. After several steps a second RCM reaction on 71 allowed preparation of bicyclic lactam 72, precursor of the target compound. Catalyst 1 was used in both reactions under the same reaction conditions. Nevertheless, whereas RCM of 69 needed 5 mol% of 1 and 5h of reaction, the second metathesis on a reaction substituted substrate finished in 24 h by using 10 mol% of 1 (Scheme 27).83
3. SYNTHESIS OF BICYCLIC COMPOUNDS
The synthesis of bicyclic lactams by RCM of acrylamides or N-alkenylamides has been widely employed as a very convenient approach to a variety of bi- and polycyclic alkaloids and related compounds. As an example, RCM of 73 catalyzed by 2 was efficiently achieved in refluxing dichloromethane to afford bicycle 74, which was further used in the synthesis of (-)-swainsonine (Scheme 28).84 The RCM reaction of this sort of compounds have also been employed as a part of several sequential processes directed to the preparation of different heterocyclic scaffolds.85
This approach based on RCM of N-allyl-δ-lactams was employed for preparing several indolizidine alkaloids including (-)-2-epilentiginosine,86 castanospermine derivatives87 and other polyhdroxylated analogues.88 The approach is also valid for N-acryloylpiperidines leading to the same indolizidine skeleton by formation of the five-membered ring.89 Similar results were obtained with 1 as a catalyst; although longer times of reaction were required, the chemical yield was excellent.90 The methodology is also applicable to the synthesis of dihydro-1H-pyrrolizin-3(2H)-ones and expanded tetrahydro-1H-pyrrolo[1,2-a]azepin-3(2H)-ones.91
Cyclization of N-allyloxazolidinone 75 catalyzed by 1 took place smoothly at room temperature providing bicyclic compound 76 in excellent yield (Scheme 29).92 Compound 76 was further converted into the alkaloids deoxymannojirimycin and swainsonine.
The reaction of N-homoallyloxazolidinone 77 bearing a phenyl-substituted alkene catalyzed by 2 was carried out under reflux and a good chemical yield was obtained after 1 h. (Scheme 30).93
In the case of phenyl-substituted alkenes similar results have been reported with 1.94 The same approach based on RCM of a phenyl-substituted N-alkenyloxazolidinone served for preparing the C10-C24 fragment of (+)-cannabisativine.95 In this case the reaction was catalyzed by 2 in refluxing dichloromethane and 88% yield was obtained. In a similar way, RCM on N-allylhydantoins 79 provided the corrresponding bicyclic structures 80 in good yield. The reaction worked well on a variety of substrates with different substituents on the double bonds thus providing a good versatility and the possibility of further functionalization (Scheme 31).96
Catalyst 82, similar to Grubbs II catalyst 2 was used for the synthesis of lactams 83 and 84 through RCM of acrylamides 81. The reaction was started at room temperature with a slow increase of temperature up to 70 °C. By using 5 mol% of catalyst, the reaction proceeded in good yield in several hours. The versatility of the reaction was well-demonstrates through the preparation of thiazolidine-, ozaxolidine-, benzothiazine and benzoxazine-fused lactams (Scheme 32).97
The quinolizidine ring system was conveniently approached by RCM reaction of dialkenyldihydropyridin-2-ones 85, catalyzed by 1 (Scheme 33). The reaction proceeded smoothly at room temperature for 2 h by using 8 mol% of catalyst.98 The reaction also proceeded well with 5 mol% of catalyst except in the case of susbtituted alkenes that required refluxing toluene for achieving a moderate yield.99 Good results were also obtained by using 2 as a catalyst even with low catalyst loadings (1.4 mol%).100
A two-directional approach to sparteine derivatives employed RCM reaction of dialkenylbispidine 87 as a key step. The tetracyclic derivative 88 was formed in good yield upon treatment with 4 mol% of 1 in dichloromethane at room temperature (Scheme 34).101
A series of 1,3-dioxo-tetrahydropyrido[1,2-c][1,3]-diazepines 90 with different substituents have been prepared by employing different catalysts and reaction conditions. In all cases good results were obtained (Scheme 35).102
N-Acryloyl-2-allylpiepridines 91 gave access to various compounds of biological interest. Among them, posion-frog alkaloids 233A, 235U and 251A were synthesized by using RCM of 91 as a key step (Scheme 36).103
The same type of substrate was employed in the preparation of a series of piperidines investigated as opioid receptor antagonists,104 for the asymmetric synthesis of cernuane-type Lycopodium alkaloids105,106 and for preparing novel polyhydroxylated quinolizidines with glycosidase inhibitory activity.107 The natural quinolizidine alkaloid (+)-cermizine C has been recently prepared through a highly efficient RCM of acrylamide 93 and further functional group transformation (Scheme 37).108
With acrylamides 95 the reaction only proceeded satisfactorily in the case of six- and seven-membered rings to give compounds 96 (n=1, 2) probably due to the steric hindrance of the corresponding precursor of the five-membered derivative 96 (n=0). Similarly, the presence of a quaternary center can affect the reaction as demonstrated by the low yield obtained in the formation of the eight-membered compound 96 (n=3) (Scheme 38).109
N-Homoallyl cyclic carbamate 97 underwent RCM upon treatment with 4 mol% of 1 to provide bicycle 98 in good yield. The reaction proceeded smoothly in dichloromethane at room temperature (Scheme 39).110
Similarly, treatment of diene 99 with 5 mol% of Grubbs II 2 afforded tricyclic derivative 100, having a 6-azaspiro[4,5]decane skeleton, in high yield (Scheme 40).111
Bicyclic structures were also accessible from dialkenylcarbamates like 101. Treatment of this compound with 1 (5 mol%) provided bicyclic derivative 102 in very good yield. The reaction was also applied to an epimer as well as to an azepine derivative (Scheme 41).112
The obtained compounds were further converted into azasugar analogues that showed inhibitory activity towards α-glycosidases at mM concentration. The same approach was employed for preparing carbohydrate-derived hybrids with 1-deoxynojirimycin which showed to be biologically active towards α-glucosidase and β-galactosidase.113 Sugar-fused piperidines have been prepared by the RCM-based methodology illustrated in Scheme 41 and further used as key precursors of deoxyazasugars.114
Isoquinuclidine 104 was obtained in good yield through RCM of divinylpiperidine 103. In spite of the unfavourable boat conformation required to bring the substituents at positions 2- and 5- into the axial orientation needed for the RCM, the reaction proceeded smoothly with 2 in refluxing dichloromethane (Scheme 42).115 Compound 104 was further used as a key intermediate in the synthesis of the alkaloid (+)-cantharanthine.
The 1,3-bis(allyl)piperidine 105 underwent RCM in the presence of catalytic 1 to give 106, an immediate precursor of the alkaloid (-)-lasubine (Scheme 43).116
Although several examples can be found in the literature concerning compatibility of free hydroxyl groups with the ruthenium-catalyzed RCM reaction,33,34,45,65,74,92 TBS protection was required in the case of compound 107 to avoid undesired elimination. The reaction was catalyzed with catalyst 5 and it was carried out in a sealed tube for 2.5 h at 60 °C (Scheme 44).117 A similar derivative was also subjected to RCM with the same catalyst and 15 h at 55 °C were needed for completing the reaction.
The indolizidine ring has also been accessed through RCM reactions from substrates with basic nitrogen atoms. Enantiomerically pure polyhydroxylate indolizidines were prepared starting from dialkenyl sugars like 109 (Scheme 45).
In spite that free amines do not tolerate completely the RCM reaction due to deactivation of the catalyst by basic nitrogen, the catalyst 2 is compatible with the presence of ammonium salts.118 Accordingly, RCM of 109 in the presence of camphorsulfonic acid (CSA) using 1 mol% of 2 proceeded successfully to afford bicyclic derivative 110.
The reaction was kept cold (10-15 °C) to avoid the obtention of a quinolizidine as a result of the formation of an intermediate aziridinium ion (resulted from an intramolecular substitution of the primary iodide by the nitrogen atom) and further ring opening by attack of the iodide counterion.119
The use of a basic N-allyl group in RCM reactions in the absence of any acid is exemplified by the reaction of 111 catalyzed by 2, which furnished the corresponding bicyclic derivative 112 in good yield. Compound 112 was used for preparing the alkaloid (+)-epiquinamide (Scheme 46).120
RCM of N-allyliminosugars has been used as a key step in the synthesis of various indolizidine skeletons including 8-epi-swainsonine. Similar approaches using both Grubbs I 1121 and Grubbs II 2122 have been reported. For the latter, the catalyst was added in two separate portions over 24 h in order to complete the reaction.123
N-Allylpyrrolidine 113 provided bicycle 114 upon treatment with catalyst 2 in two portions of 10 mol% of catalyst over 4 h. The reaction was carried out in toluene at 100 °C to achieve 80% yield (Scheme 47).124 Compound 114 was used as a precursor of (+)-6-epi-castanospermine.
The enantioselective synthesis of the alkaloid (+)-meloscine was achieved by constructing the last ring through RCM reaction catalyzed by 2 (Scheme 48).125
4. CONCLUDING REMARKS
The ring-closing metathesis reactions of nitrogen-containing compounds have been used intensively for the synthesis of a variety of six-membered nitrogen heterocycles, clearly establishing that this reaction has become a standard synthetic methodology in the arsenal of heterocyclic chemists. The tetrahydropyridines and related derivatives prepared by RCM reactions are excellent precursors for the synthesis of saturated nitrogen heterocycles bearing a resemblance either to natural products or bioactive molecules. The synthetic potential of the intermediates generated through this chemistry has been further explored successfully. Nevertheless, although tremendous advances have been achieved in the field of RCM reactions, the applications of such processes to heterocyclic chemistry will continue to grow.
ACKNOWLEDGEMENTS
Thanks are due to the Spanish Ministry of Science and Innovation (Project CTQ2010-19606, Madrid, Spain), FEDER Program and the Government of Aragón (Group E-10, Zaragoza, Spain). E.M. thanks the MEC for a pre-doctoral grant (FPU Program).
References
1. For Nobel Lectures see: R. H. Grubs, Angew. Chem. Int. Ed., 2006, 45, 3760; CrossRef and Adv. Synth. Cat., 2007, 349, 34; CrossRef R. R. Schrock, Angew. Chem. Int. Ed., 2006, 45, 3748; CrossRef and Adv. Synth. Cat., 2007, 349, 41; CrossRef Y. Chauvin, Angew. Chem. Int. Ed., 2006, 45, 3740; CrossRef and Adv. Synth. Cat., 2007, 349, 27. CrossRef
2. G. C. Vougioukalakis and R. H. Grubbs, Chem. Rev., 2010, 110, 1746. CrossRef
3. Y. Schrodi and R. L. Pederson, Aldrichimica Acta, 2007, 40, 45.
4. L. Gulajski, M. Mauduit, and K. Grela, Pure Appl. Chem., 2009, 81, 200. CrossRef
5. C. Samojlowicz, M. Bieniek, and K. Grela, Chem. Rev., 2009, 109, 3708. CrossRef
6. ‘Metathesis in Natural Product Synthesis: Strategies, Substrates and Catalysis’, ed by J. Cossy, S. Arseniyadis, and C. Meyer, Wiley: New York, 2010.
7. A. H. Hoveyda, S. J. Malcolmson, S. J. Meek, and A. R. Zhugralin, Angew. Chem. Int. Ed., 2010, 49, 34.
8. S. P. Nolan and H. Clavier, Chem. Soc. Rev., 2010, 39, 3305. CrossRef
9. Adv. Synth. Catal. 2007, 349, 1 (Several authors, Olefin Metathesis Special Issue). CrossRef
10. R. H. Grubbs,‘Handbook of Metathesis’, Wiley-VCH: Weinheim, 2003; Vols. 1-3. CrossRef
11. S. Kotha, M. Meshram, and A. Tiwari, Chem. Soc. Rev., 2009, 38, 2065. CrossRef
12. A. M. Lozano-Vila, S. Monsaert, A. Bajek, and F. Verpoort, Chem. Rev., 2010, 110, 4865. CrossRef
13. H. Mutlu, L. Montero de Espinosa, and M. A. R. Meier, Chem. Soc. Rev., 2011, 40, 1404. CrossRef
14. R. H. Grubbs, Tetrahedron, 2004, 60, 7117. CrossRef
15. A. Fürstner, Angew. Chem. Int. Ed., 2000, 39, 3012. CrossRef
16. M. Schuster and S. Blechert, Angew. Chem. Int. Ed., 1997, 36, 2036. CrossRef
17. T. M. Trnka and R. H. Grubbs, Acc. Chem. Res., 2001, 34, 18. CrossRef
18. R. H. Grubbs, S. J. Miller, and G. C. Fu, Acc. Chem. Res., 1995, 28, 446. CrossRef
19. L. Yet, Chem. Rev., 2000, 100, 2963. CrossRef
20. S. K. Chattopadhyay, S. Karmakar, T. Biswas, K. C. Majumdar, H. Rahaman, and B. Roy, Tetrahedron, 2007, 63, 3919. CrossRef
21. K. J. Ivin, J. Mol. Cat. A: Chem., 1998, 133, 1. CrossRef
22. H. M. A. Hassan, Chem. Commun., 2010, 9100. CrossRef
23. K.-i. Takao and K.-i. Tadano, Heterocycles, 2010, 81, 1603. CrossRef
24. W. A. L. van Otterlo and C. B. de Koning, Chem. Rev., 2009, 109, 3743. CrossRef
25. K. C. Majumdar, S. Muhuri, R. U. Islam, and B. Chattopadhyay, Heterocycles, 2009, 78, 1109. CrossRef
26. . CrossRef
27. G. C. Fu, S. T. Nguyen, and R. H. Grubbs, J. Am. Chem. Soc., 1993, 115, 9856. CrossRef
28. A. J. Vernall and A. D. Abell, Aldrichimica Acta, 2003, 36, 93.
29. A. J. Phillips and A. D. Abell, Aldrichimica Acta, 1999, 32, 75.
30. F.-X. Felpin and J. Lebreton, Eur. J. Org. Chem., 2003, 3693. CrossRef
31. J. S. Yadav, M. S. Reddy, P. P. Rao, and A. R. Prasad, Synthesis, 2006, 4005. CrossRef
32. M. S. Reddy, M. Narender, and K. R. Rao, Tetrahedron, 2006, 63, 331. CrossRef
33. H. Ouchi, Y. Mihara, and H. Takahata, J. Org. Chem., 2005, 70, 5207. CrossRef
34. C. Alegret, X. Ginesta, and A. Riera, Eur. J. Org. Chem., 2008, 1789. CrossRef
35. C. Ohara, R. Takahashi, T. Miyagawa, Y. Yoshimura, A. Kato, I. Adachi, and H. Takahata, Bioorg. Med. Chem. Lett., 2008, 18, 1810. CrossRef
36. B. Danieli, G. Lesma, D. Passarella, A. Sacchetti, and A. Silvani, Tetrahedron Lett., 2005, 46, 7121. CrossRef
37. P. P. Saikia, G. Baishya, A. Goswami, and N. C. Barua, Tetrahedron Lett., 2008, 49, 6508. CrossRef
38. A. Kamal, S. R. Vangala, N. V. Subba Reddy, and V. Santhosh Reddy, Tetrahedron: Asymmetry, 2009, 20, 2589. CrossRef
39. L. Banfi, G. Guanti, M. Paravidino, and R. Riva, Org. Biomol. Chem., 2005, 3, 1729. CrossRef
40. A. K. Srivastava, S. K. Das, and G. Panda, Tetrahedron, 2009, 65, 5322. CrossRef
41. M. Venkataiah and N. W. Fadnavis, Tetrahedron, 2009, 65, 695. CrossRef
42. M. Venkataiah, B. Venkateswara Rao, and N. W. Fadnavis, Tetrahedron: Asymmetry, 2009, 20, 198. CrossRef
43. S.-J. Pyun, K.-Y. Lee, C.-Y. Oh, J.-E. Joo, S.-H. Cheon, and W.-H. Ham, Tetrahedron, 2005, 61, 1413. CrossRef
44. L.-S. Li and S. C. Sinha, Tetrahedron Lett., 2009, 50, 2932. CrossRef
45. R. Rengasamy, M. J. Curtis-Long, W. D. Seo, S. H. Jeong, I.-Y. Jeong, and K. H. Park, J. Org. Chem., 2008, 73, 2898. CrossRef
46. Z.-F. Xie, Z. Chai, G. Zhao, and J.-D. Wang, Synthesis, 2008, 3805. CrossRef
47. T. M. Shaikh and A. Sudalai, Tetrahedron: Asymmetry, 2009, 20, 2287. CrossRef
48. D. Gagnon, S. Lauzon, C. Godbout, and C. Spino, Org. Lett., 2005, 7, 4769. CrossRef
49. L. Boisvert, F. Beaumier, and C. Spino, Can. J. Chem., 2006, 84, 1290. CrossRef
50. S. Lauzon, F. Tremblay, D. Gagnon, C. Godbout, C. Chabot, C. Mercier-Shanks, S. Perreault, H. DeSeve, and C. Spino, J. Org. Chem., 2008, 73, 6239. CrossRef
51. F. A. Davis, Y. Zhang, and D. Li, Tetrahedron Lett., 2007, 48, 7838. CrossRef
52. F. A. Davis and Y. Zhang, Tetrahedron Lett., 2009, 50, 5205. CrossRef
53. O. Pavlyuk, H. Teller, and M. C. McMills, Tetrahedron Lett., 2009, 50, 2716. CrossRef
54. T. S. Cooper, A. S. Larigo, P. Laurent, C. J. Moody, and A. K. Takle, Org. Biomol. Chem., 2005, 3, 1252. CrossRef
55. I. S. Kim, Y. J. Ji, and Y. H. Jung, Tetrahedron Lett., 2006, 47, 7289. CrossRef
56. I. S. Kim, J. S. Oh, O. P. Zee, and Y. H. Jung, Tetrahedron, 2007, 63, 2622. CrossRef
57. M. Pena-Lopez, M. M. Martinez, L. A. Sarandeses, and J. P. Sestelo, Org. Lett., 2010, 12, 852. CrossRef
58. A. Fürstner and J. Ackerstaff, Chem. Commun., 2008, 2870. CrossRef
59. H. Nomura and C. J. Richards, Org. Lett., 2009, 11, 2892. CrossRef
60. M. L. Bennasar, T. Roca, and D. Garcia-Diaz, Synlett, 2008, 1487. CrossRef
61. M. L. Bennasar, T. Roca, and D. Garcia-Diaz, J. Org. Chem., 2008, 73, 9033. CrossRef
62. F. M. Sabbatini, R. Di Fabio, C. Griffante, G. Pentassuglia, L. Zonzini, S. Melotto, G. Alvaro, A. M. Capelli, L. Pippo, E. Perdona, Y. St. Denis, S. Costa, and M. Corsi, Bioorg. Med. Chem. Lett., 2010, 20, 623. CrossRef
63. S. K. Chattopadhyay, T. Biswas, and T. Biswas, Tetrahedron Lett., 2008, 49, 1365. CrossRef
64. S. K. Chattopadhyay, K. Sarkar, L. Thander, and S. P. Roy, Tetrahedron Lett., 2007, 48, 6113. CrossRef
65. H. Takahata, Y. Suto, E. Kato, Y. Yoshimura, and H. Ouchi, Adv. Synth. Catal., 2007, 349, 685. CrossRef
66. T. Ayad, Y. Genisson, and M. Baltas, Org. Biomol. Chem., 2005, 3, 2626. CrossRef
67. D. D. Dhavale, S. M. Jachak, N. P. Karche, and C. Trombini, Synlett, 2004, 1549. CrossRef
68. A. Boto, Y. De Leon, J. A. Gallardo, and R. Hernandez, Eur. J. Org. Chem., 2005, 3461. CrossRef
69. A. K. Ghosh, G. Schiltz, R. S. Perali, S. Leshchenko, S. Kay, D. E. Walters, Y. Koh, K. Maeda, and H. Mitsuya, Bioorg. Med. Chem. Lett., 2006, 16, 1869. CrossRef
70. H. Bittermann, F. Boeckler, J. Einsiedel, and P. Gmeiner, Chem.Eur. J., 2006, 12, 6315. CrossRef
71. S. K. Ghosh, C. Ko, J. Liu, J. Wang, and R. P. Hsung, Tetrahedron, 2006, 62, 10485. CrossRef
72. A. F. Gasiecki, J. L. Cross, R. F. Henry, V. Gracias, and S. W. Djuric, Tetrahedron Lett., 2008, 49, 6286. CrossRef
73. J. H. Lee, S. Shin, J. Kang, and S.-G. Lee, J. Org. Chem., 2007, 72, 7443. CrossRef
74. I. D. Jenkins, F. Lacrampe, J. Ripper, L. Alcaraz, P. Van Le, G. Nikolakopoulos, P. de Almeida Leone, R. H. White, and R. J. Quinn, J. Org. Chem., 2009, 74, 1304. CrossRef
75. A. Niida, S. Oishi, Y. Sasaki, M. Mizumoto, H. Tamamura, N. Fujii, and A. Otaka, Tetrahedron Lett., 2005, 46, 4183. CrossRef
76. D. Bensa, I. Coldham, P. Feinaeugle, R. B. Pathak, and R. J. Butlin, Org. Biomol. Chem., 2008, 6, 1410. CrossRef
77. A. Niida, H. Tanigaki, E. Inokuchi, Y. Sasaki, S. Oishi, H. Ohno, H. Tamamura, Z. Wang, S. C. Peiper, K. Kitaura, A. Otaka, and N. Fujii, J. Org. Chem., 2006, 71, 3942. CrossRef
78. A. Niida, M. Mizumoto, T. Narumi, E. Inokuchi, S. Oishi, H. Ohno, A. Otaka, K. Kitaura, and N. Fujii, J. Org. Chem., 2006, 71, 4118. CrossRef
79. G. Lesma, B. Danieli, A. Sacchetti, and A. Silvani, J. Org. Chem., 2006, 71, 3317. CrossRef
80. K. Takahashi, H. Nakano, and R. Fujita, Tetrahedron Lett., 2005, 46, 8927. CrossRef
81. T. P. Ribelin, A. S. Judd, I. Akritopoulou-Zanze, R. F. Henry, J. L. Cross, D. N. Whittern, and S. W. Djuric, Org. Lett., 2007, 9, 5119. CrossRef
82. J. Minville, J. Poulin, C. Dufresne, and C. F. Sturino, Tetrahedron Lett., 2008, 49, 3677. CrossRef
83. S. Ghosh and J. Shashidhar, Tetrahedron Lett., 2009, 50, 1177. CrossRef
84. J. Ceccon, A. E. Greene, and J.-F. Poisson, Org. Lett., 2006, 8, 4739. CrossRef
85. J. D. Sunderhaus, C. Dockendorff, and S. F. Martin, Org. Lett., 2007, 9, 4223. CrossRef
86. T. Muramatsu, S. Yamashita, Y. Nakamura, M. Suzuki, N. Mase, H. Yoda, and K. Takabe, Tetrahedron Lett., 2007, 48, 8956. CrossRef
87. V. Zambrano, G. Rassu, A. Roggio, L. Pinna, F. Zanardi, C. Curti, G. Casiraghi, and L. Battistini, Org. Biomol. Chem., 2010, 8, 1725. CrossRef
88. (a) N. Langlois, B. K. Le Nguyen, P. Retailleau, C. Tarnus, and E. Salomon, Tetrahedron: Asymmetry, 2006, 17, 53; CrossRef (b) G. Lesma, A. Colombo, A. Sacchetti, and A. Silvani, J. Org. Chem., 2009, 74, 590. CrossRef
89. G. Lesma, A. Colombo, N. Landoni, A. Sacchetti, and A. Silvani, Tetrahedron: Asymmetry, 2007, 18, 1948. CrossRef
90. A. G. H. Wee, G.-J. Fan, and H. M. Bayirinoba, J. Org. Chem., 2009, 74, 8261. CrossRef
91. F. Mo, F. Li, D. Qiu, and J. Wang, Tetrahedron, 2010, 66, 1274. CrossRef
92. R. Martin, C. Murruzzu, M. A. Pericas, and A. Riera, J. Org. Chem., 2005, 70, 2325. CrossRef
93. M. S. M. Pearson, R. O. Saad, T. Dintinger, H. Amri, M. Mathe-Allainmat, and J. Lebreton, Bioorg. Med. Chem. Lett., 2006, 16, 3262. CrossRef
94. K. Maki, M. Kanai, and M. Shibasaki, Tetrahedron, 2007, 63, 4250. CrossRef
95. S. Chandrasekhar and B. Tiwari, Tetrahedron: Asymmetry, 2009, 20, 1924. CrossRef
96. N. Dieltiens, D. D. Claeys, V. V. Zhdankin, V. N. Nemykin, B. Allaert, F. Verpoort, and C. V. Stevens, Eur. J. Org. Chem., 2006, 2649. CrossRef
97. K. Schulz, M. Watzke, K. Johannes, P. Ullrich, and J. Martens, Synthesis, 2009, 665. CrossRef
98. J. G. Sosnicki, Tetrahedron Lett., 2006, 47, 6809. CrossRef
99. S.-S. P. Chou, C.-F. Liang, T.-M. Lee, and C.-F. Liu, Tetrahedron, 2007, 63, 8267. CrossRef
100. J. G. Sosnicki, Synlett, 2009, 2508. CrossRef
101. N. R. Norcross, J. P. Melbardis, M. F. Solera, M. A. Sephton, C. Kilner, L. N. Zakharov, P. C. Astles, S. L. Warriner, and P. R. Blakemore, J. Org. Chem., 2008, 73, 7939. CrossRef
102. N. Dieltiens, D. D. Claeys, B. Allaert, F. Verpoort, and C. V. Stevens, Chem. Commun., 2005, 4477. CrossRef
103. N. Toyooka, S. Kobayashi, D. Zhou, H. Tsuneki, T. Wada, H. Sakai, H. Nemoto, T. Sasaoka, H. M. Garraffo, T. F. Spande, and J. W. Daly, Bioorg. Med. Chem. Lett., 2007, 17, 5872. CrossRef
104. B. Le Bourdonnec, A. J. Goodman, M. Michaut, H.-F. Ye, T. M. Graczyk, S. Belanger, T. Herbertz, G. P. A. Yap, R. N. DeHaven, and R. E. Dolle, J. Med. Chem., 2006, 49, 7278. CrossRef
105. Y. Nishikawa, M. Kitajima, and H. Takayama, Org. Lett., 2008, 10, 1987. CrossRef
106. Y. Nishikawa, M. Kitajima, N. Kogure, and H. Takayama, Tetrahedron, 2009, 65, 1608. CrossRef
107. N. Kumari and Y. D. Vankar, Org. Biomol. Chem., 2009, 7, 2104. CrossRef
108. G. Cheng, X. Wang, D. Su, H. Liu, F. Liu, and Y. Hu, J. Org. Chem., 2010, 75, 1911. CrossRef
109. S. Fustero, N. Mateu, L. Albert, and J. L. Acena, J. Org. Chem., 2009, 74, 4429. CrossRef
110. R. W. Bates, J. A. Nemeth, and R. H. Snell, Synthesis, 2008, 1033. CrossRef
111. A. Adrien, H.-J. Gais, F. Koehler, J. Runsink, and G. Raabe, Org. Lett., 2007, 9, 2155. CrossRef
112. B. G. Reddy and Y. D. Vankar, Angew. Chem. Int. Ed., 2005, 44, 2001. CrossRef
113. A. Kumar, G. K. Rawal, and Y. D. Vankar, Tetrahedron, 2008, 64, 2379. CrossRef
114. S. Ghosh, J. Shashidhar, and S. K. Dutta, Tetrahedron Lett., 2006, 47, 6041. CrossRef
115. L. Moisan, P. Thuery, M. Nicolas, E. Doris, and B. Rousseau, Angew. Chem. Int. Ed., 2006, 45, 5334. CrossRef
116. S. Liu, Y. Fan, X. Peng, W. Wang, W. Hua, H. Akber, and L. Liao, Tetrahedron Lett., 2006, 47, 7681. CrossRef
117. P. Kim, L. Zhang, U. H. Manjunatha, R. Singh, S. Patel, J. Jiricek, T. H. Keller, H. I. Boshoff, C. E. Barry, III, and C. S. Dowd, J. Med. Chem., 2009, 52, 1317. CrossRef
118. D. L. Wright, J. P. Schulte, and M. A. Page, Org. Lett., 2000, 2, 1847. CrossRef
119. S. H. L. Verhelst, B. P. Martinez, M. S. M. Timmer, G. Lodder, G. A. Van der Marel, H. S. Overkleeft, and J. H. Van Boom, J. Org. Chem., 2003, 68, 9598. CrossRef
120. T. L. Suyama and W. H. Gerwick, Org. Lett., 2006, 8, 4541. CrossRef
121. M. Nath, R. Mukhopadhyay, and A. Bhattacharjya, Org. Lett., 2006, 8, 317. CrossRef
122. A. J. Murray and P. J. Parsons, Synlett, 2006, 1443. CrossRef
123. A. J. Murray and P. J. Parsons, and P. Hitchcock, Tetrahedron, 2007, 63, 6485. CrossRef
124. J. Louvel, C. Botuha, F. Chemla, E. Demont, F. Ferreira, and A. Perez-Luna, Eur. J. Org. Chem., 2010, 2921. CrossRef
125. P. Selig and T. Bach, Angew. Chem. Int. Ed., 2008, 47, 5082. CrossRef