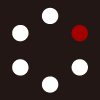
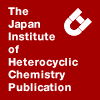
HETEROCYCLES
An International Journal for Reviews and Communications in Heterocyclic ChemistryWeb Edition ISSN: 1881-0942
Published online by The Japan Institute of Heterocyclic Chemistry
e-Journal
Full Text HTML
Received, 1st October, 2011, Accepted, 18th October, 2011, Published online, 25th October, 2011.
■ Preparation and Synthetic Applications of Azetidines
Tina M. Bott and F. G. West*
Gunning/Lemieux Chemistry Center, Department of Chemistry, University of Alberta, Edmonton, Alberta T6G 2G2, Canada
Abstract
Azetidines (four-membered saturated nitrogen heterocycles) are found in a diverse range of natural products, and also serve as valuable building blocks for other structural classes. The ring-strain inherent in these compounds poses challenges in their preparation, and a number of creative methods for the synthesis of substituted azetidines are presented. The application of azetidines in the construction of acyclic products or other heterocyclic systems is also described. Finally, recent examples of the use of chiral, enantiomerically pure azetidines in asymmetric catalysis are detailed.CONTENTS
I. Introduction
II. Azetidine-containing Natural Products
III. Synthesis of Azetidines
A. Ring-closure By C–N Bond Formation
B. Ring-closure By C–C Bond Formation
C. Reduction of β-Lactams and Cycloaddition Reactions
IV. Synthetic Applications of Azetidines
A. Ring-Opening to Acyclic Amines
B. Ring Expansion to Pyrrolidines and Other Five-Membered Heterocycles
C. Ring Expansion to Six-Membered Heterocycles
D. Ring Expansion to Medium-Sized Heterocycles
E. Application of Azetidines in Catalysis
V. References
INTRODUCTION
Azetidine (2) is the parent molecule of a class of small, nitrogen-containing heterocycles, which have been gaining attention for their versatility in many areas of chemistry.1 The increased exploration into the chemistry of these molecules stems from the interesting behaviours they exhibit in synthetic and biochemical settings as well as the development of new methods to synthesize them. It has been found that these strained four-membered rings can display properties of their smaller or larger-ring counterparts, aziridines (1) or pyrrolidines (3), depending on the nature of their substitution pattern and their chemical environment (or the reaction conditions applied).
The physical properties of many different azetidines have been reported in the literature. In general, azetidines are conformationally puckered, ridgid, molecules with bond angles bent 10-20 degrees out of planarity depending on their substitution pattern.2 The diffraction data of azetidine has been used to calculate the bond lengths and bond angles present in the molecule, which are shown in Table 1. The lone pair on nitrogen is favored to be in the pseudoaxial position; however, with an inversion barrier of ~10 kcal/mol, inversion at room temperature does occur.3
The ring strain energy associated with the azetidine ring has been experimentally determined to be 25.2 kcal/mol.4 This number is very close to that observed for aziridine at 26.7 kcal/mol. In contrast, the ring strain energies for pyrrolidine and piperidine are 5.8 and 0 kcal/mol respectively. Interestingly, although strained, azetidines are more similar to pyrrolidines than aziridines when it comes to several of their physical properties. For example, the pKa of azetidine (11.29)2 is much closer to that of pyrrolidine (11.31)2 than to aziridine (7.98).5 In terms of basicity, this means that azetidine can behave like a typical secondary amine in most reactions.
A comparison of the 1H NMR chemical shift data of these three heterocycles also shows a much closer resemblance of azetidine (2) to pyrrolidine (3) rather than aziridine (1), as the bond angles are not deformed enough to induce an upfield chemical shift as seen in aziridine (Figure 2).6 On examination of the coupling constants (J), however, the addition of substituents on either carbon or nitrogen can significantly affect the observed J-values between geminal protons, as commonly observed with aziridines, whereas this effect is not as prominent in pyrrolidines.
Figure 3 shows some examples of the effect changing the substituent on nitrogen (4, 5 and 6) as well as the addition of a substituent on C-4 (7 and 8) can have on the observed J2,2’-values.1a,7 This is most likely due to changes in the puckering angle of the ring, which affects the hybridization on the carbon atoms, and can be explained by application of the geminal Karplus curve.6 These data are especially notable because they give insight into the conformational changes that can occur in these molecules when different substituents are present. This will be an important factor later on in this review when there are significant changes in the reactivity of one azetidine vs. another based on differences in their substitution pattern around the ring.
AZETIDINE-CONTAINING NATURAL PRODUCTS
Although azetidine itself has not been found in nature there are several analogues that have been isolated. L-Azetidine-2-carboxylic acid (9), the first azetidine natural product to be discovered, was isolated form Convallaria majalis (lily of the valley) in 1955.8 Research into the role of this molecule suggests it is a proline receptor antagonist affecting the structure of proteins in its natural predators.9 It has subsequently been found in several other plants and is the parent molecule for a number of other natural products found in both plants and animals (Figure 4).1b
Mugineic acid (10), 2’-deoxymugineic acid (11) and nicotianamine (12) are structurally related phytosidophores which are produced in plants to aid in the uptake of iron for chlorophyll biosynthesis. Due to this interesting biological activitity, there have been a number of synthetic efforts towards their production.10 Penaresidin A and B (13 and 14) have also been the targets of several syntheses as they have been found to exhibit biological activity in the activation of ATPase in actomycin.11 Several members of the polyoxin family of compounds (15) have proven valuable as fungicides in an agricultural setting.12 The most recently reported natural product containing the azetidine moiety, calydaphninone (16), was isolated from the leaves and twigs of Daphniphyllum calycillum in 2007.13 This molecule, containg a 4-azatricyclo[5.2.2.0]undecane core, represents one of the most complex azetidine-containing natural products seen to date.
The natural products in Figure 4 all possess at least one stereogenic center on the azetidine ring. The presence of up to three contiguous stereogenic centers, seen in 13 and 14, as well as an α-quaternary center, seen in 16, illustrate the challenges that can arise in the preparation of such compounds. The need to synthesize the azetidine core with complete control over the stereochemistry is important, as it is well known that the biological activity of a molecule is often directly related to the relative and absolute configuration of its substituents.
SYNTHESIS OF AZETIDINES
Increased activity in the area of azetidine synthesis over the last few decades has been driven by growth in their application in both synthetic and medicinal chemistry. The azetidine ring system, however, is one of the most challenging ring systems to form of the common azaheterocycles (5>3>6>7≈4). The difficulty in its formation lies in the increased ring strain of the target molecule once it is formed. This makes the ring closure significantly uphill in energy. Although there are multiple reported methods for the synthesis of azetidines in the literature, only a few are generally applicable to form a diverse range of products with the ability to place substituents at different positions around the ring.1a-c This section will focus on summarizing the most versatile methods for azetidine synthesis with a highlight of some promising new methodology.
Ring Closure by C–N Bond Formation. The displacement of a leaving group by nitrogen is the oldest and most commonly used route for the formation of the azetidine ring system. This method, which allows for the incorporation of multiple substituents on various positions around the ring, has taken on several variations over the past few decades. Scheme 1 shows some general methods for azetidine formation that are present in the literature. These methods include, but are not limited to: addition of amines (18) to 1,3-dielectrophiles (19), ring closure of γ-haloamines or activated γ-aminoalcohols (20), reductive cyclization of γ-haloimines (21) or α-amino aldehydes/ketones (22), activation of allylamines (23) and ring-opening of epoxides or aziridines (24).
The double SN2 cyclization of 1,3-electrophiles with primary amines is well established in the literature. A classic example is the synthesis of azetidine-2-carboxylic esters 26 from the reaction of primary amines with 2,4-dibromobutyrates 25 (Scheme 2).14 This approach generally allows for a number of different alkyl or aryl substituents on both R1 and R2 to accommodate for subsequent functionalization. A wide variety of other leaving groups have also been utilized, including chlorides, iodides, triflates, mesylates, tosylates and sulfonic esters depending on the nature of the reaction media. Unfortunately, a drawback of this methodology is the tendency for polymerization and/or elimination side reactions to occur during prolonged heating, which contributes to low yields.
The advancement of microwave technology has been fortuitous for the preparation of azetidines by this type of displacement. Burkett and coworkers have reported the synthesis of a variety of different N-substituted azetidines in good yields by reaction of primary amines with the cyclic sulfate of propanediol (27) (Scheme 3).15 Initial formation of 3-(amonio)propyl sulfates (28) followed by 15 minutes of microwave irradiation in basic aqueous media gave rise to analytically pure azetidines (29) in moderate to good yields.
The ability to access enantiopure 1,3-aminoalcohols has been key to the successful construction of enantiopure azetidines. In 2005, the Enders group reported the synthesis of N-tosyl-2,3-disubstituted azetidines (34) with excellent diastereo- and enantioselectivities from the cyclization of 1,3-aminoalcohols derived from SAMP/RAMP-hydrazone methodology (Scheme 4).16 Most notably, the authors were subsequently able to transform the 2-phenyl substituent on 34 to the acid (35) by oxidation with ruthenium tetroxide followed by deprotection of the amine. The small amounts of acyclic products (36) obtained were most likely the result of ring opening followed by oxidation at the benzylic position.
On a similar note, Das and coworkers have recently reported the synthesis of N-tosyl-2,4-disubstituted azetidines (41) from N-tosylaldimines (37) and acetophenone (38, Scheme 5).17 The authors treated a mixture of 37 and 38 with BF3⋅OEt2 in dichloromethane to generate β-amino ketone 39, which was subsequently reduced to a mixture of syn and anti 1,3-aminoalcohols (40) in the presence of sodium borohydride. After separation, the anti-isomer of 40 was refluxed with potassium hydroxide and tosyl chloride to give the cis-azetidine product 41 in 57-64% yield over three steps with only one purification step.
The Yadav group has reported a clever way to access activated 1,3-aminoalcohols derivatives in situ for the synthesis of azetidine-3-carbonitriles 44 and azetidine-3-carboxylates 45 by the addition of phosphoramidates (42) to Morita-Baylis-Hillman adducts (43, Scheme 6).18 This one pot protocol involves treatment of diethyl N-arylphosphoramidates (42) with sodium hydride followed by addition of Morita-Baylis-Hillman adduct 43 to form aza-Michael intermediate I. Intramolecular attack of the alkoxide ion on phosphorus generates intermediate II, which opens to III before displacement of the phosphate ester with the amide nitrogen. The resulting azetidine (44/45) is isolated in 94-96% yield with exclusively the trans stereoisomer.
The conversion of α-amino aldehydes 46 to aminoiodohydrins 47 with Sm/CH2I2 followed by treatment with silver tetrafluoroborate has resulted in the formation of stable enantiopure 3-hydroxy-azetidinium salts 48 in good yields which could be almost quantitatively monodebenzylated to the azetidine (49) by hydrogenolysis (Scheme 7).19
The activation of allylic and homoallylic amines for the regioslective synthesis of azetidines has been accomplished with several alkene-activating agents. The most commonly employed reagents are electrophilic halogen sources, which give rise to halonium ion intermediates that are displaced by the pendant amine to form the desired azetidine. In an example of this approach by the Rousseau (Scheme 8), activation of allylic amines (50) with an electrophilic bromine source, results in the construction of azetidines (51) in moderate to good yields by a 4-endo cyclization.20
The Outurquin group has described the activation of homoallylic amines (52) with phenylselenyl halides resulting in the generation of both azetidines (53) and pyrrolidines (54).21 The ratio of the observed products was initially found to be dependent on the number of equivalents of the activating agent used as well as the substitution alpha to the nitrogen (Scheme 9).
More recently, however, the group has been able to fine-tune this methodology to allow for preferential azetidine formation without being constrained by substitution at the α-position. The authors can instead change the substitution on the alkene portion of allylamine 55 to preferentially form either the azetidine (56) or the pyrrolidine (57) without having to rely exclusively on the Thorpe-Ingold effect to induce cyclization to the desired product (Table 2).
In 2011, Szymoniak and coworker published an interesting synthesis of N-Boc-protected azetidines through a diastereoselective hydrozirconation of chiral allylic amines.22 Treatment of enantiomerically pure allyl amines 58 with Schwartz’ reagent allowed for syn-hydrozirconation of the double bond (V), which was then converted to iodide 59. The addition of NaHMDS promoted cyclization to the cis-2,3-disubstituted azetidine 60 in moderate to good yields (Scheme 10). This reaction, which is tolerant of a wide variety of substituent types (alkyl, aryl, CH2OR) and possesses an easily removable nitrogen protecting group, allows for simple access to synthetically useful, diastereometrically enriched azetidines in a short reaction sequence.
Ring Closure by C–C Bond Formation. There are significantly fewer examples of azetidine synthesis by a carbon-carbon bond formation in the literature compared to the carbon-nitrogen bond forming approaches discussed above. One advantage of this disconnection is the ability to place groups on nitrogen that are not typically amenable to nucleophilic displacement reactions. An example of this was reported by Luche and coworkers in 1994 (Scheme 11).23 Starting from glyoxylic acid (61), a one-pot reductive amination/protection sequence gave N-(ω-chloroethyl-Boc-glycine (62). Cyclization of 62 in the presence of LDA led to N-Boc-protected azetidine-2-carboxylic acid 63.
In the same year the Shue group reported the synthesis of cis- and trans-3-phenylazetidine-2-carboxylic acids using a similar ring closing strategy.24 Conversion of tert-butyl bromoacetate (64) to the amine (65), followed by reduction and treatment with thionyl chloride generated chloroeth ylamine 66 in excellent yield. Cyclization of 66 upon treatment with NaHMDS resulted in formation of azetidine 67 (Scheme 12). Removal of the benzyl protecting groups provided a mixture of the cis and trans isomers 68a and 68b in overall yields of 35% and 6% respectively.
More recently, a non-racemic approach to azetidines with varied substitution was reported starting from commercially available enantiopure 1,2-amino alcohols.25 The synthesis of 2,4-disubstituted azetidine 73 was achieved starting from (S)-phenylalaninol (69, Scheme 13). This procedure involved reductive amination of 69 to give N-benzyl (S)-phenylalaninol 70 followed by generation of oxazolidine 71 in the presence of formaldehyde. Subsequent ring opening with potassium cyanide in the presence of citric acid followed by chlorination gave 72, which cyclized to a mixture of diastereomeric azetidines 73a and 73b when treated with LiHMDS.
Disubstituted amino alcohols (1R,2S)-ephedrine (74) and (1S,2S)-pseudoephedrine (75) can also be utilized with this methodology to generate 2,3,4-trisubstituted azetidines (Scheme 14). Both compounds 74 and 75 were alkylated with bromoacetonitrile in the presence of base to give amines 76 and 77. The hydroxyl groups were then converted to the chlorides before treatment with LiHMDS, which led to diastereomeric mixtures of tri-substituted azetidines 80 and 81. The diastereomeric ratios obtained for the tri-substituted products are superior to that of disubstituted azetidine 73 (Scheme 13). The authors were also happy to report that there is no loss of enantiopurity during this process.
Subsequent reports by the Couty group have shown that these 2-cyano-azetidines (82) are very versatile to further transformations (Scheme 15). The cyano group can be hydrolyzed to give azetidinic amino acids or esters (83), reacted with nucleophiles to form α-amino aldehydes and ketones (84) and β-amino alcohols (85), reduced to provide β-amino amines (87) and olefinated to produce 2-vinyl azetidines (88). 1d,26
A practical extension reported by this group has been the synthesis of azetidines by intramolecular Michael addition (Scheme 16). Conversion of the hydroxyl group of 89 to α,β-unsaturated ester 90 by a one-pot oxidation/Wittig protocol, followed by treatment with LiHMDS yields a 1:1 mixture of azetidines 91a and 91b, epimeric at C-2. This method expands the potential of this reaction to include products with different handles on the 3-position.
All of the above ring closures have been the result of selective deprotonation of an acyclic substrate with strong base followed by ring formation. An attractive contrast to this method is the use of photochemical cyclization reported by the Wessig group (Scheme 17).27 Starting from enantiopure aminodiol 92 they were able to synthesize ketone 93, which upon irradiation formed biradical intermediate VI. Recombination of the biradical gives azetidine 94.
Reduction of β-Lactams and Cycloaddition Reactions. The construction of azetidines by cycloaddition reactions has mainly focused on the formation of β-lactams followed by subsequent reduction of the carbonyl. There are a variety of stereoselective methods available to generate the β-lactam framework. The reaction of a ketene (95) with an imine (96), known as the Staudinger reaction, is one of the most commonly used methods for the formation of β-lactams (97) (Scheme 18). 1b,28
This reaction proceeds through a stepwise mechanism where the imine nitrogen reacts with the ketene carbonyl, forming a zwitterionic intermediate which then cyclizes to give the desired product (Scheme 19). The nitrogen attacks from the less hindered side of the ketene in a perpendicular fashion resulting in intermediate VII with RL(large) and R1 in the same side. Concurrent rotation of the iminium into the plane of the C-C double bond and conrotatory ring closure furnishes the cis-substituted product 97a. Conversely, when R1 can stabilize a positive charge, isomerization of intermediate VII to IX followed by ring closure results in RL and R1 being trans to one another in the final product (97b). This method can tolerate a wide range of functional groups and provides good diastereoselectivity when the substrates are appropriately substituted.
A related process for the generation of β-lactams is the [2+2]-cycloaddition of isocyanates with olefins (Scheme 20). Chlorosulfonylisocyanate (98) is a readily available reagent often used because of its high reactivity and the easy removal the chlorosulfonyl group on nitrogen.29 Although related to the Staudinger reaction, the mechanism of this cycloaddition process is concerted. The geometry of the olefin (99) controls the relative stereochemistry of the products (100), with cis olefins leading to syn products and trans to anti. Diastereomeric mixtures, however, are produced if epimerization occurs during the removal of the chlorosulfonyl protecting group to give the free β-lactams (101).
Reduction of β-lactams, such as those formed using the above-mentioned routes, can be effected with a number of different reagents. That being said, the use of DIBAL-H and chloroalanes has emerged as the most efficient method as it does not lead to reductive ring opening, a problem often observed with the use of diborane, LiAlH4 and Raney nickel.1b
Although the reduction of β-lactams can be a fine approach to azetidines there can be problems associated with the reduction of other functional groups present in the molecule. For that reason several groups have looked into using a cycloaddition methodology to access azetidines directly. In 1995, Uyehara and coworkers reported a Lewis-acid promoted reaction of N-substituted aldimines (102) with allyltrisisopropylsilane (103, Scheme 21).30 The reaction is postulated to go through an intermediate β-silyl cation (X), which is stable at – 78 ºC but upon warming to room temperature cyclizes to a mixture of cis and trans azetidines (104a and 104b).
In 2003, while investigating the aza-Morita-Baylis-Hillman (aza-MBH) reaction of N-tosylimines (105) with ethyl 2,3-butadienoate (106), Shi and coworkers came across an interesting tactic for the synthesis of azetidines (107) when DABCO was used as the catalyst (Scheme 22).31 A very recent expansion of this novel transformation allows for the formation of enantiomerically enriched products (85-98% ee) when DABCO is replaced with cincona alkaloid-derived base 110.32
A plausible mechanism for this “abnormal” aza-MBH reaction is shown in Scheme 23. The authors suggest that the use of DABCO leads to an ambident intermediate (XI) which undergoes preferential attack on the imine at the γ-position of the MBH adduct XII, leading to amide XIII. Closure of the four-membered ring by an addition-elimination mechanism then affords 107.
A recent report from the Fan group allows for the stereoselective construction of azetidines using a conceptually distinct iodine-mediated oxidative cyclization (Scheme 24).33 Addition of 2-aminomalonate 112 into chalcones 113 provided Michael adducts 114, which upon treatment with iodosobenzene in the presence of tetrabutylammonium iodide gave azetidines 115 as single diastereomers.
The mechanistic hypothesis proposed by the group was based on the results of several important control experiments. The authors believe that there are two viable pathways to generate the final products from Michael adduct 114 (Scheme 25). Pathway a starts with reaction of the amide nitrogen of 114 with iodine(III) species 116 generated from reaction of iodosobenzene with tetrabutylammonium iodide. Intermediate XV next undergoes an intramolecular reductive elimination to give azetidine 115. The alternate mechanism, pathway b, involves tautomerization of the ketone followed by hyperiodination of the enol leading to intermediate XVI. Intramolecular attack by nitrogen with concomitant reductive elimination of PhI would also generate azetidine 115.
The ability to synthesize the azetidine framework in good yield from the readily available, versatile building blocks has opened the door for the use of these compounds in other synthetic applications. One possible limitation in many of these approaches is the use of phenyl or aryl groups to direct the stereochemical outcome and increase the stability of the reactants/products. Fortunately, reports in the literature for the conversion of phenyl groups to carboxylic acids on these types of strained ring systems (see Scheme 4) makes this approach more amenable for other applications.
SYNTHETIC APPLICATIONS OF AZETIDINES
As noted in the introduction, the ring strain energy associated with the azetidine system is quite high (~25 kcal/mol), which makes it a good building block for a wide variety of secondary transformations that result in a lower energy product. This section will illustrate the versatility of this small ring system, which provides access to many different types of structures by different modes of nitrogen activation.
Ring-Opening to Acyclic Amines. As mentioned above, azetidines can behave similarly to either aziridines or pyrrolidines under different reaction conditions. One way that azetidines behave similarly to aziridines is in their capacity to undergo nucleophilic ring opening to give acyclic products.
Activating the nitrogen of an azetidine with Lewis or Brønsted acid followed by addition of an external nucleophile has been found to effect ring opening of azetidines. Mann and coworkers have utilized BF3⋅OEt2 to activate the nitrogen of N-nosyl or N-tosyl-2-phenylazetidines (117, Scheme 26).34 After activation, the addition of an allylsilanes (118) provided access to amino-olefins 119. This ring opening was regioselective, occurring at the benzylic position, and is most likely due to polarization of the benzylic C-N bond upon complexation of the Lewis acid with nitrogen.
Aryl borates have also successfully been employed for the ring opening of N-tosyl-2-phenylazetidine (120, Scheme 27).35 In this case, the Lewis acid is playing a dual role as both the activating agent and the nucleophile in the construction of amino aryl ethers (121). Several observations noted by the authors during this work give insight into some of the pros and cons of this methodology for the facilitation of ring opening. First, switching the protecting group on nitrogen from tosyl to methyl resulted in none of the ring-opened product being observed. This suggests that the protecting group on nitrogen must be electron withdrawing and could be a limitation for Lewis acid-promoted ring openings if its removal is difficult. The second observation was that the reaction of enantiopure N-tosyl-2-phenylazetidine with aryl borates gave a racemic product. This racemization, most likely due to ring opening to a carbocation intermediate, suggesting that the strength of Lewis acid complexation with nitrogen and the carbocation stabilizing ability of the group on the 2-position are crucial components that need to be fine-tuned for a successful enantioselective ring opening.
A unique synthesis of enantiomerically pure α,β-unsaturated acyl silanes was accomplished through the Brønsted acid catalyzed ring opening of properly functionalized azetidines (Scheme 28).36 Starting from 1-methoxyallenylsilane 122 and α-imino ester 123 the authors were able to synthesize azetidine-2-carboxylate 124 in 90% yield and 97% ee in the presence of catalytic [Cu(MeCN)4]BF4 and (R)-Tol-BINAP. Treatment with acid then afforded the desired acyl silane 125 with no erosion of enantiopurity.
In 2006, the Couty group reported an investigation into the reaction of N-methyl and N-benzyl azetidines with chloroformates.37 The reaction of tertiary amines with chloroformates is well established in the literature as a method for the selective removal of N-methyl or N-benzyl groups.38 The authors, who wanted to determine whether this process was also applicable to azetidines, found instead that treating azetidines 126 with methylchloroformate 127 resulted in the formation of N-protected γ-chloramines (128) in good to excellent yields (Scheme 29).
In general, the ring opening was found to be highly regioselective, with the chloride anion attacking the more electrophilic position, and stereospecific, occurring via a SN2 displacement. No erosion of enantiopurity or change in diastereomeric ratio was detected. This ring opening, unlike those associated with Lewis acid activation as mentioned above, allowed for exocyclic alkyl groups to be present on nitrogen and resulted in an easily removable protecting group thereafter.
An interesting observation was made when the authors examined the influence of relative stereochemistry of the starting materials on the products (Scheme 30). A comparison of 126a-d showed that the all syn azetidine 126c and the anti-syn azetidine 126d produced 128c and 128d as well as products from chloride attack at the less electrophilic center 129a and 129b. Although the authors did not speculate on a reason for this observation it could be possible that having substituents on the 3- and 4-position syn to one another may be affecting the puckering angle of the ring, which allows for competitive attack on the C-4.
Another aspect of azetidine ring activation that has recently been explored by the Couty group deals with ring-opening reactions of azetidinium salts. 1d,39 Starting with enantiopure azetidines (130), alkylation with methyl triflate gives the azetidinium salts (131), which can then be opened with a wide range of nucleophiles to give acyclic products 132-135 (Scheme 31). Successful nucleophiles include hydride, azide, amines, acetates, alkoxides, cyanates and malonate salts. In most cases the yields are good to excellent with no observable competition by eliminative pathways. When enantiopure azetidines are used as substrates the reaction occurs in a stereospecific manner.
It should be noted that a complementary example recently reported by Wang and coworkers using α-amido azetidine 136 allows access to γ-amino amides (137) with quaternary centers alpha to the carbonyl (Scheme 32).40
The high regioselectivity observed in these reactions strongly depends on the substitution pattern of the starting azetidine. In general, SN2 attack at an unsubstituted α-carbon will occur preferentially over that of a substituted one. If both α-carbons possess substituents, however, nucleophilic attack occurs preferentially at the most electrophilic carbon center, which in the case of compound 131b is C-2 (Scheme 33).
As with the observations this group made regarding the ring opening of azetidines with chloroformates (vide supra) the authors again found that the starting geometry of the azetidine plays a major role in the outcome of the reaction. This is exemplified by the result of nucleophilic addition of sodium azide to 2,3-trans azetidine salt 131c and 2,3-cis azetidine salt 131d (Scheme 34).
The authors attribute this drastic difference in yield to the ability of each conformer to undergo nucleophilic opening (Scheme 35). For 2,3-trans azetidine 131c the equilibrium should favour conformer A over conformer B; this arranges all of the substituents in a pseudo-equatorial orientation. Unfortunately, due to blocking by the phenyl group, this preferred conformation (A) does not allow access to the electrophilic center for ring opening. Ring opening can only occur with conformer B, which suffers from several severe 1,3-diaxial interactions, thus rationalizing the poor yield of this transformation. With 2,3-cis azetidine salt 131d, however, the conformer that allows for unhindered attack by the azide (conformer D) suffers less repulsive 1,3-diaxial interactions as the cyano group is in the equatorial position. Although this conformer is still less stable than C, the equilibrium will not be subject to the same level of bias as in the case of conformer A for compound 131c.
Several different hydride sources were tested as nucleophiles to compare the rate of ring opening and to determine whether the ester or cyano moieties could be preserved during the reaction.39d The use of LiAlH4 to open the ring was moderately successful but, as expected, reduced both ester and cyano groups to the alcohol or amine respectively. Making the switch to boron-derived reagents NaBH4 and NaCNBH3 enabled the authors to observe reductive ring opening in much higher yields while preserving the ester or cyano groups. The use of NaBH(OAc)3, on the other hand, surprisingly induced ring opening by attack with an acetate ion. The regioselectivity of the ring opening using the boron reducing agents was the same as that observed with C, N and O-nucleophiles (see Scheme 31).
Exploration into the ring opening of azetidinium salt 131e with carbon nucleophiles was successful with both potassium cyanide and the sodium salt of diethyl malonate (138), generating amines 132e and 132f respectively (Scheme 36).39c Unfortunately, more basic nucleophiles such as the enolates derived from ethyl acetoacetate (139), acetophenone (140) or tert-butyl acetate (141) resulted in the formation of azetidinium ylide 142 by deprotonation at the C-2 position.
Although the authors were initially disappointed by these results they did note that the epoxide 143 was formed from the reaction of azetidine 131e with the enolate of acetophenone (139, Scheme 37). In open-chained or larger heterocycles the formation of an epoxide by displacement of an ammonium leaving group is not very successful due to the high energetic barrier for ring closure. With the use of a strained azetidinium ylide, however, the energy gain associated with breaking the four-membered ring compensates for that energy barrier and increases the feasibility of such an approach.
This observation led to the exploration of epoxide formation/ring opening with aldehydes and ketones.41 The proposed mechanism of the reaction is shown in Scheme 38. The reaction proceeds with the production of a single diastereomer and, when tested with enantiomerically pure starting materials produced enantiomerically pure products.
In order to confirm the importance ring strain to the success of this reaction the authors synthesized the pyrrolidine analogue 144 and subjected it to the same reaction conditions (Scheme 39). The only reactivity observed with 144 was epimerization at the α-position to a mixture of 144/epi-144. The authors state that this is due to the absense of any ring strain to help drive the reaction toward epoxide ring closure and thus results in the reversion back to the starting materials.
This process was further extended to the formation of cyclopropanes (147) by the addition of azetidinium ylide 131d into Michael acceptors (146, Scheme 40).42 The cyclopropanation was shown by the authors to proceed via the mechanism shown. Reversible 1,4-addition and potential ylide epimerization were found to occur during reactions performed by the authors in their search to understand the convergence of epimeric starting materials into one product.
Interestingly, when the authors again tested for the importance of ring strain in the success of the reaction by subjecting pyrrolidine analogue 144 to the same reaction conditions, they were able to isolate small amounts of the homologous cyclopropanated product 149 (Scheme 41). This was in contrast to the result shown in Scheme 39, when the same pyrrolidine ylide was exposed to an aldehyde electrophile, where the only reactivity observed was epimerization at the α-position of the pyrrolidinium salt. This suggests that there are other factors, such as the lesser ability of the cyclopropane product 149 to revert back to starting materials, involved in the outcome of the reaction.
Ring Expansion to Pyrrolidines and Other 5-Membered Heterocycles. Although the ring expansion of azetidines to pyrrolidines was first observed several decades ago, the application of this process for synthetic purposes was not significantly developed until recently. Several research groups have explored the potential of the one-carbon ring expansion of azetidines to pyrrolidines in the last decade.
In 1970, Masuda and coworkers reported the ring expansion of N-tert-butyl-2-methanesulfonyloxy- methylazetidine 150 to pyrrolidine 152 when it was left at room temperature for a few hours.43 A similar result was obtained when they treated 150 with triphenylphosphine dibromide. The authors proposed that the reaction could proceed via either the quaternary ammonium salt XIX or the phosphonium salt XVIII (Scheme 42).
Since the early observations by Masuda and coworkers several other groups have observed this ring expansion process in azetidines possessing reasonable leaving groups on the 2-methyl substutuent. In 1999, Alcaide and coworkers observed the ring expansion of azetidine 154, with either an acetal and thioacetal-protected aldehydes on the 2-position, when treated with diethylaluminum chloride (Scheme 43).44 In these examples the nature of the aldehyde protecting group had a large effect on the outcome of the reaction.
A few years later, a report concerning the formation of 1,2-dialkyl-4-halopyrrolidines (160) was published.45 The authors generated 2-halomethylazetidines 158/159 from selenomethylazetidine precursors (157) and upon subsequent heating these substrates underwent ring expansion to give 160 (Scheme 44).
Around the same time, the Couty group had also begun an investigation into this process, looking in depth at how the nature of the leaving group, relative substitution and stereochemistry of the starting material affect the outcome of the reaction.26b, 46 To get a good idea of the effect each factor had on the reaction the authors synthesized a variety of differently substituted, enantiopure azetidines with primary, secondary or tertiary hydroxyalkyl groups being present on the 2-position.
Conversion of primary α-hydroxymethylazetidines 161 and 164 to α-chloromethylazetidines 162 and 165 using thionyl chloride proceeded in excellent yields. Subsequent ring expansion to 3-chloropyrrolidines 163 and 166 could be initiated upon heating to 120 ºC in DMF, albeit in low to moderate yields (Scheme 45).
Treatment of 161 and 164 with mesyl chloride at only room temperature, however, resulted in formation of both mesylates 167 and 169 as well as some of the pyrrolidine products. Full conversion to pyrrolidines 168 and 170, in an overall higher yield than that observed with the chloride case, was accomplished by heating the crude mesylates in chloroform at reflux (Scheme 46).
Both of the above transformations resulted in formation of a single pyrrolidine product with the same relative stereochemistry as the starting azetidines. This suggested to the authors that the ring expansion is occurring via the intermediate aziridinium ion XX (Scheme 47). Recent computational experiments performed by the same group support this mechanism.47 Additional reactions involving other primary α-hydroxymethylazetidines suggest that multiple substituents on the ring are well tolerated and do not change the stereochemical outcome of the reaction.
Activation of the secondary α-hydroxymethylazetidines 173 and 175 with either the chlorination or mesylation conditions led to a diastereomeric mixture of activated products 174 and 176 (Scheme 48). This is may be due to the possibility of chlorination occurring via a SN1 mechanism rather then the SN2 mechanism seen with the primary α-hydroxymethylazetidines 161 and 164 (Schemes 45 and 46). Subsequent ring expansion of either of the separable diastereomers occurred without incident. It should be also noted that in a recent publication by De Kimpe and coworkers that this problem can be solved by setting the stereochemistry of the chloride earlier in the azetidine synthesis.48 Unfortunately, all attempts to ring expand tertiary α-hydroxymethylazetidines only resulted in elimination products.
Addition of external nucleophiles such as hydroxide, cyanide, azide, acetate or fluoride allowed for generation of pyrrolidines (178) with a variety of substitutions at C-3 (Scheme 49). Most of these transformations were performed on the easily isolable chloro-substrates (177). The temperature of the reactions was found to be crucial for the formation of the desired products. Too low a temperature resulted in ring expansion with only chloride acting as the nucleophile while too high a temperature led to eliminative byproducts.
Nucleophilic ring openings with fluoride were performed using diethylaminosulfur trifluoride (DAST), which works as both the hydroxyl activator and the source of fluoride (Scheme 50).49
Ring-expansion of azetidines is also possible using Lewis acid activation. The BF3⋅OEt2-mediated rearrangement of 2-aminomethylazetidines (181) provides access to 3-aminopyrrolidines 182 in moderate to good yields.50 The mechanism for this ring expansion is shown in Scheme 51 and involves coordination of the azetidine nitrogen to the Lewis acid (XXV), followed by concomitant aziridination and azetidine ring opening to give intermediate XXVI. Migration of the Lewis acid to the aziridine nitrogen then allows for a second intramolecular nucleophilic displacement to occur, opening aziridinium intermediate XXVII and to provide 3-aminopyrrolidine 182.
This process, which provides access to highly substituted 3-aminopyrrolidines, was also applied to the synthesis of the pyrrolizidine alkaloid (-)-absouline (189, Scheme 52). The synthesis began with the addition of 3-benzyloxypropyl lithium to enantiomerically pure azetidine 183 followed by reduction of the resulting imine to give the desired 2-aminomethylazetidine (184) in 64% yield and a dr of 25:1. Activation of the azetidine nitrogen with BF3⋅OEt2 initiated the ring expansion to pyrrolidine 185, which was obtained in 80% yield with no change in the diastereomeric ratio. Protection of the primary amine as the carbamate followed by removal of the benzyl protecting groups on both oxygen and the ring nitrogen by hydrogenolysis gave amino-alcohol 186. Activation of the alcohol of 186 with triphenylphosphine resulted in a smooth cyclization to pyrrolizidine 187. The synthesis was completed by standard removal of the N-Boc protecting group and DCC-mediated coupling of the newly revealed primary amine with (E)-p-methoxycinnamic acid (188).
In the same year, the West group published a different approach to the pyrrolizidine alkaloid framework using the ring expansion of an azetidinium ylide and demonstrated its applicability in the synthesis of the natural products turneforcidine (198) and platynecine (199).51 This synthesis relied on a different mode of activation for the azetidine than any of those discussed above, utilizing the in situ generation of an ammonium ylide through reaction of a tertiary amine with a metallocarbene, followed by ring expansion via a Stevens [1,2]-shift.
To examine the potential of this project the authors first decided to test the ring expansion of the azetidine in an intermolecular fashion (Scheme 53). Readily available 1-benzylazetidine-2-carboxylate 190 was reacted with ethyl diazoacetate (EDA) in the presence of a copper catalyst. In general, the Stevens rearrangement involves a migration of the group, which is best able to stabilize a radical intermediate. In unstrained systems this would result in preferential benzyl migration over ring opening (migration of a CH2CO2Me group).52 The authors hoped that the ring strain associated with the azetidine in this case would be a driving force for its expansion. The resulting product, pyrrolidine 191, was the result of the Stevens [1,2]-shift of the internal C-N bond bearing the CH2CO2Me group. This promising result convinced the authors that this process would be viable intramolecularly as well.
The synthesis of the natural products also began with 1-benzylazetidine-2-carboxylate 190 (Scheme 54). Removal of the N-benzyl protecting group under transfer hydrogenation conditions followed by alkylation to incorporate the tethered diazo moiety provided the desired substrate 193 in excellent yield. Next, 193 was treated with 10 mol% Cu(acac)2 and heated to reflux in benzene to initiate the formation of an ammonium ylide intermediate and promote the ring expansion to diastereomeric pyrrolizidines 194 and 195 in a ratio of 3.6:1. Subsequent reduction of the ketones in the presence of Adams catalyst gave hydroxyester 196 and lactone 197 respectively. Separation and subsequent reduction of 192 and 193 with LiAlH4 led to the natural products turnaforcidine (198) and platynecine (199).
Recently, West and coworkers have reported a more thorough examination into the ring expansion of azetidines (200) to pyrrolidines (202) via the Stevens 1,2-shift (Scheme 55).53 This methodology, which is shown to tolerate a wide range of functional groups on both nitrogen and on the α-position of the ring, has led to a general procedure for the preparation of highly-substituted pyrrolidines in one step from simple building blocks.
Alongside the obvious success in the ring expansion of azetidines to pyrrolidines there have also been reports of the successful conversion of azetidines to other 5-membered heterocycles. In 2000, the Concellón group reported the formation of 1,3-oxazolidines 204 when primary amines were added to a solution of enantiopure 3-hydroxyazetidinium salts 203 in dichloromethane (Scheme 56).19 The intermediate ring-opened diaminoalcohol XXXI reacts in situ with dichloromethane to generate the 1,3-oxazolidine.
Another class of 5-membered ring heterocycles that is commonly used in organic synthesis and can be accessed from azetidines is the oxazolidinones. Oxazolidinones are very important molecules for asymmetric synthesis and are commonly employed as chiral auxiliaries.54 In 2011, the Couty group reported a synthesis of chiral oxazolidinones by activation of α-hydroxymethylazetidines 205 with bis(trichloromethyl) carbonate (BTC), which is a safer substitute for phosgene.55 This reaction, which proceeds through bicyclic azetidinium intermediate XXXII, followed by nucleophilic ring opening with chloride ion, allows for the formation of a wide variety of enantiomerically pure oxazolidinones (206) in moderate yields (Scheme 57).
One drawback the authors noted was that the placement of a substituent on the azetidine at the 4-position resulted in conversion of the α-hydroxymethylazetidine 207 into the α-chloromethylazetidine 208 without any of the desired ozazolidinone being detected. This is most likely due to steric hindrance by the substituent at C-4 preventing attack by the chloride at that position (Scheme 58).
Ring Expansion to Six-Membered Heterocycles. The expansion of the azetidines to piperidines was first reported by the De Kimpe group as an extension of their work towards pyrrolidine synthesis mentioned previously.56 The authors were able to synthesize enantiopure 2-(2-bromoalkyl)azetidines (209) which, upon heating, closed to the bicyclic azetidinium intermediate XXXIII (Scheme 59). Nucleophilic attack by the bromide ion opened XXXIII to 4-bromopiperidine 210. Ring opening with external nucleophiles such as hydroxide, azide or cyanide has also been successful, allowing access to other 4-substituted piperidine derivatives.
The Ghorai group has published several papers on the activation of 2-aryl-N-tosylazetidine 211 with either Zn(II) or Cu(II) Lewis acids followed by ring opening of the activated intermediate (XXXIV) with either a nitrile or carbonyl nucleophile.57 This approach is similar to that of the activation with BF3⋅OEt2 described previously and resulted in [4+2] adducts 212 and 213 (Scheme 60). Their recent expansion of this methodology to include more highly substituted and enantiopure starting materials adds a great deal of potential to this process.
Ring Expansion to Medium-Sized Heterocycles. The formation of medium-sized rings (between 7-13 atoms) is one of the more challenging problems faced by synthetic chemists. This is largely due to the transannular effects (unfavourable interactions) experienced in these systems.58 In the beginning of this chapter it was noted that the ease of forming azaheterocycles generally follows the order of 5>3>6>7≈4. This being said, there has been a great deal of interest in the formation of medium-sized 7- and 8-membered azaheterocycles, largely due to the substantial number of natural products that contain these moieties.
As discussed in the above section, the formation and subsequent ring expansion of azetidinium ylides is a viable method for the synthesis of functionalized pyrrolidines. The Couty group has reported two variations on this methodology to access both azepines and azocine dereivatives.59 The first method, reported in 2006, was found to generate either 2,3,6,7-tetrahydroazepines (215) or pyrrolidines (216) depending on the geometry of the starting azetidine. The authors synthesized a variety of 2-alkenyl-azetidines (214), which were then alkylated to give the azetidinium triflate salts in excellent yields. Treatment of these salts with a strong base generated the ylide intermediate XXXVII which subsequently underwent a [1,2]-shift to give pyrrolidine 216 or a [2,3]-sigmatropic rearrangement to give 2,3,6,7-tetrahydroazepine 215 (Scheme 61).
The outcome of the reaction was dependent on the geometry of the alkenyl substituent on the 2-position of the azetidine ring relative to the newly formed bond to nitrogen after alkylation. The [2,3]-rearrangement occurs when these substituents are cis to one another while the [1,2]-shift product is the result of a trans relationship (Scheme 62).
A slight modification to this procedure allowing for access to azocines derivatives with the same 2-alkenyl azetines (214) was reported a few years later. In this case, activation of the nitrogen by alkylation with activated alkynes leads to intermediate XXXIX, which can subsequently undergo a [3,3]-sigmatropic rearrangement to give the 1,2,3,6-tetrahydroazocines (217, Scheme 63).
The formation the desired product (217) was found to be sensitive to the relative configuration of the substituents on the ring. The authors found that ring opening of 214a to give acyclic product 219 was competitive with the formation of 217a, which was consistent in other cases where R4 and/or R5 ≠ H (Scheme 64). This is believed to be the result of steric congestion inpeding the requisite conformation for the rearrangement.
The formation of azocinone derivatives is also possible through the rhodium-catalyzed ring opening of 3-alkylideneazetidines (216, Scheme 65).60 The ring expansion is hypothesized to occur via C-H activation of 220 to give XLII, followed by hydrometallation of the C-C double bond to give metallocyclopentanone XLIII. Rearrangement of XLII to XLIII followed by reductive elimination would lead to the observed product 221.
In 2009, Ghorai and coworkers reported the enantioselective synthesis of 1,4-oxazepanes and 1,5-oxazocines via ring opening of azetidines with bromoalcohols (Scheme 66).61 This procedure, which took enantiopure N-tosyl azetidines 222 and activated then with Cu(OTf)2 in the presence of the alcohol, resulted in ring opening to the acyclic amine 223. Subsequent addition of potassium hydroxide facilitated closure of the larger ring to generate diastereomers 224a and 224b.
With mono-substituted azetidines (R = H) it was found that the enantiomeric excess (e.e.) decreased during the reaction. The authors believe that this is due to partial racemization at the benzylic center of the starting azetidines in the presence of copper triflate, which has been experimentally observed with aziridines. The 2,4-disubstituted substrates, however, remained at >99% e.e. This is because the necessary epimerization of the benzylic position for racemization to occur would lead to the substituents on the ring being cis to one another, which is less favourable (Figure 5).
The Roy group has also reported a ring expansion involving Lewis acid activation of 2-substituted N-tosyl-azetidines to give hydrooxazocenes.62 This methodology utilizes Ag(I)-catalyzed dual activation of both azetidine 225 and propargylic alcohol 226. This activation triggers a ring opening/closure cascade, which results in formation of 8-membered heterocycles 227 (Scheme 67).
The number of reports for successful ring expansion of azetidines to larger ring systems over the last few years has started to increase. This is most likely due to the ability to access a large array of enantiopure azetidines from readily available and affordable starting materials. The following section will show some of the interesting ring systems that have been accessed recently.
Application of Azetidines in Catalysis. With the recent development of methodologies to quickly synthesize azetidines in enantiomerically pure form, it is not suprising that their implementation as either ligands for metal-catalyzed reactions or as chiral auxiliaries in organocatalysis is increasing. The first examples of the successful application of azetidines as chiral ligands were reported in the 1990’s and involved the borane reduction of aldehydes and ketones (Scheme 68).63 The success in these cases is presumed to derive from the rigid framework of the oxaborolidines (229) as both acyclic and 5-memberd ring analogues resulted in lower enantiomeric excesses.
Along the same lines, the asymmetric alkylation of aldehydes with diethylzinc has also been examined using azetidines with alcohol substituents as bidentate chiral ligands (Scheme 69). 26,64 Over the last decade there have been several reports in which the transformation is both highly enantioselective and high yielding with a variety of differently substituted chiral azetidines (233-241).
Azetidines have also been used as ligands with palladium in Suzuki-Miyaura cross-coupling reactions,65 and with rhodium for enantioselective cyclopropanation reactions.66 The enantiocontrol observed in the cyclopropanation using azetidine-4-carboxylate-derived rhodium complexes (246, Scheme 70) is notable as it generally leads to the preferential formation of cis-cyclopropanes, which is in contrast to the preference for the trans-cyclopropanes observed with other similar 5-membered ring analogues 247.
The success of proline and its derivatives in organocatalysis is well documented in the literature.67 Not surprisingly, the application of azetidines as organocatalysts for in situ formation of chiral iminium or enamine species has also been successful. In 2006, Greck and coworkers reported the asymmetric α-amidation of ketones with dibenzyl azodicarboxylate (DBAD) using L-azetidine 2-carboxylic acid (9) as the catalyst (Scheme 71).68 It should be noted that the authors also performed the reactions using L-proline as the catalyst but the yields and enantiomeric excesses were lower than those observed with 9.
The use of azetidinic 1,2-diamines as organocatalysts for asymmetric Michael additions has also been reported recently.69 In this paper, the authors screened a variety of thioureas derived from differently substituted primary and seconday α-aminomethyl-azetidines 255 and 3-aminopyrrolidines 256 for the addition of diethylmalonate (251) to β-nitrostyrene (250, Scheme 72). They found that only catalyst 257 showed high catalytic activity and after optimization led to the formation of the Michael adduct 253 in 77% yield and an e.r. of 68:32 (S:R).
Interestingly, when diethyl malonate 251 was replaced with acetoacetone 252 the absolute configuration of the Michael adduct (254) was reversed (e.r. = 12:88 (S:R)). The authors believe this reversal is due to the difference in pKa of the two nucleophiles. Scheme 72 shows the proposed transitions state for the reaction with diethyl malonate, which has a pKa of 13. In this case, hydrogen bonding between the nitro group and the thiourea along with concomitant deprotonation of the enol form of diethylmalonate by the proximate tertiary amine leads to attack on the Si face of the nitrostyrene and generation of (S)-253 as the major enantiomer.
The reaction with acetoacetone 252 (pKa = 9), on the other hand, would lead to attack on the Re face. This is due to the more facile deprotonation of 252 resulting in the formation of an ammonium species, which can then hydrogen bond with the nitro group. If the enolate then coordinates to the thiourea, the result is the transition state shown in Scheme 73, which illustrates how (R)-254 is formed preferentially.
CONCLUSION
Overall, the advancements made in the chemistry of azetidines in the last few decades say a great deal about the potential of these small molecules. The above summary has highlighted some of the most versatile methods for the generation of both simple and highly functionalized azetidines, as well as some of the new methods that hold potential to be so in the future. Their application in the synthesis of a wide range of molecules, from enantiopure acyclic amines to ordinarily challenging medium-sized heterocycles, shows they are great asset in multiple areas of chemistry.
ACKNOWLEDGEMENTS
We thank NSERC for ongoing support, and TMB thanks the University of Alberta for Queen Elizabeth II Graduate Scholarship.
References
1. N. De Kimpe, ‘Comprehensive Heterocyclic Chemistry II’ 1996, 1B, p. 507; CrossRef A. Brandi, S. Cicchi, and F. M. Cordero, Chem. Rev., 2008, 108, 3988; CrossRef G. S. Singh, M. D'hooghe, N. De Kimpe, ‘Comprehensive Heterocyclic Chemistry III’ 2008, 2, pp. 3-110; F. Couty and G. Evano, Synlett, 2009, 3053. CrossRef
2. J. A. Moore and R. S. Ayers, ‘Small Ring Heterocycles, Pt. 2: Azetidines, β-Lactams, Diazetidines, and Diaziridines,’ Vol. 42, ed. by A. Hassner, Wiley, New York, 1983, p. 656.
3. F. A. L. Anet, J. Am. Chem. Soc., 1985, 107, 4335. CrossRef
4. S. W. Benson, F. R. Cruickshank, D. M. Golden, G. R. Haugen, H. E. O'Neal, A. S. Rodgers, R. Shaw, and R. Walsh, Chem. Rev., 1969, 69, 279; CrossRef T. Dudev and C. Lim, J. Am. Chem. Soc., 1998, 120, 4450; CrossRef R. D. Bach and O. Dmitrenko, J. Org. Chem., 2002, 67, 3884. CrossRef
5. A. Padwa, ‘Comprehensive Heterocyclic Chemistry III,’ Vol. 1, ed. by A. R. Katritzky, Elsevier, 2008, pp. 1-105. CrossRef
6. R. M. Silverstein and F. X. Webster, ‘Spectrometric Identification of Organic Compounds,’ 6th ed., John Wiley & Sons, New York, 1997.
7. M. Laguerre, C. Boyer, J.-M. Leger, and A. Carpy, Can. J. Chem., 1989, 67, 1514. CrossRef
8. L. Fowden, Nature (London), 1955, 176, 347. CrossRef
9. F. Couty and G. Evano, Org. Prep. Proced. Int., 2006, 38, 427. CrossRef
10. T. Shioiri, Y. Hamada, and F. Matsuura, Tetrahedron, 1995, 51, 3939; CrossRef K. Miyakoshi, J. Oshita, and T. Kitahara, Tetrahedron, 2001, 57, 3355; CrossRef S. Singh, G. Crossley, S. Ghosal, Y. Lefievre, and M. W. Pennington, Tetrahedron Lett., 2005, 46, 1419. CrossRef
11. S. Knapp and Y. Dong, Tetrahedron Lett., 1997, 38, 3813; CrossRef J. Beauhaire and P.-H. Ducrot, Synth. Commun., 1998, 28, 2443; CrossRef D.-G. Liu and G.-Q. Lin, Tetrahedron Lett., 1999, 40, 337; CrossRef K. Ohshita, H. Ishiyama, Y. Takahashi, J. Ito, Y. Mikami, and J. Kobayashi, Biorg. Med. Chem., 2007, 15, 4910; CrossRef S. Raghavan and V. Krishnaiah, J. Org. Chem., 2009, 75, 748. CrossRef
12. K. Isono, K. Asahi, and S. Suzuki, J. Am. Chem. Soc., 1969, 91, 7490. CrossRef
13. Y.-T. Di, H.-P. He, Y.-S. Wang, L.-B. Li, Y. Lu, J.-B. Gong, X. Fang, N.-C. Kong, S.-L. Li, H.-J. Zhu, and X.-J. Hao, Org. Lett., 2007, 9, 1355. CrossRef
14. H. H. Wasserman, B. H. Lipshutz, A. W. Tremper, and J. S. Wu, J. Org. Chem., 1981, 46, 2991. CrossRef
15. B. A. Burkett, S. Z. Ting, G. C. S. Gan, and C. L. L. Chai, Tetrahedron Lett., 2009, 50, 6590. CrossRef
16. D. Enders and J. Gries, Synthesis, 2005, 3508. CrossRef
17. B. Das, P. Balasubramanyam, B. Veeranjaneyulu, and G. Chinna Reddy, J. Org. Chem., 2009, 74, 9505. CrossRef
18. L. D. S. Yadav, V. P. Srivastava, and R. Patel, Tetrahedron Lett., 2008, 49, 5652. CrossRef
19. J. M. Concellón, P. L. Bernad, and J. A. Pérez-Andrés, Tetrahedron Lett., 2000, 41, 1231. CrossRef
20. S. Robin and G. Rousseau, Eur. J. Org. Chem., 2000, 3007. CrossRef
21. X. Pannecoucke, F. Outurquin, and C. Paulmier, Eur. J. Org. Chem., 2002, 995; CrossRef X. Franck, S. Leleu, and F. Outurquin, Tetrahedron Lett., 2010, 51, 4437. CrossRef
22. T. K. Pradhan, K. S. Krishnan, J.-L. Vasse, and J. Szymoniak, Org. Lett., 2011, 13, 1793. CrossRef
23. A. De Nicola, C. Einhorn, J. Einhorn, and J. L. Luche, J. Chem. Soc., Chem. Commun., 1994, 879. CrossRef
24. D. J. Blythin, M. J. Green, M. J. R. Lauzon, and H.-J. Shue, J. Org. Chem., 1994, 59, 6098. CrossRef
25. C. Agami, F. Couty, and G. Evano, Tetrahedron: Asymmetry, 2002, 13, 297. CrossRef
26. F. Couty and D. Prim, Tetrahedron: Asymmetry, 2002, 13, 2619; CrossRef F. Couty, F. Durrat, and D. Prim, Tetrahedron Lett., 2003, 44, 5209; CrossRef F. Couty, G. Evano, and N. Rabasso, Tetrahedron: Asymmetry, 2003, 14, 2407. CrossRef
27. P. Wessig and J. Schwarz, Helv. Chim. Acta, 1998, 81, 1803. CrossRef
28. G. S. Singh, Tetrahedron, 2003, 59, 7631. CrossRef
29. J. K. Rasmussen and A. Hassner, Chem. Rev., 1976, 76, 389. CrossRef
30. T. Uyehara, M. Yuuki, H. Masaki, M. Matsumoto, M. Ueno, and T. Sato, Chem. Lett., 1995, 789. CrossRef
31. G.-L. Zhao, J.-W. Huang, and M. Shi, Org. Lett., 2003, 5, 4737. CrossRef
32. J.-B. Denis, G. Masson, P. Retailleau, and J. Zhu, Angew. Chem. Int. Ed., 2011, 50, 5356. CrossRef
33. Y. Ye, H. Wang, and R. Fan, Org. Lett., 2010, 12, 2802. CrossRef
34. M. Domostoj, I. Ungureanu, A. Schoenfelder, P. Klotz, and A. Mann, Tetrahedron Lett., 2006, 47, 2205. CrossRef
35. F. Bertolini, S. Crotti, V. D. Bussolo, F. Macchia, and M. Pineschi, J. Org. Chem., 2008, 73, 8998. CrossRef
36. T. Akiyama, K. Daidouji, and K. Fuchibe, Org. Lett., 2003, 5, 3691. CrossRef
37. M. Vargas-Sanchez, S. Lakhdar, F. Couty, and G. Evano, Org. Lett., 2006, 8, 5501. CrossRef
38. J. Campbell, J. Org. Chem., 1957, 22, 1259; CrossRef R. A. Olofson, J. T. Martz, J. P. Senet, M. Piteau, and T. Malfroot, J. Org. Chem., 1984, 49, 2081. CrossRef
39. F. Couty, F. Durrat, and G. Evano, Targets in Heterocyclic Systems, 2005, 9, 186; F. Couty, O. David, F. Durrat, G. Evano, S. Lakhdar, J. Marrot, and M. Vargas-Sanchez, Eur. J. Org. Chem., 2006, 3479; CrossRef F. Couty, O. David, and B. Drouillat, Tetrahedron Lett., 2007, 48, 9180; CrossRef F. Couty, O. David, and F. Durrat, Tetrahedron Lett., 2007, 48, 1027. CrossRef
40. D.-H. Leng, D.-X. Wang, J. Pan, Z.-T. Huang, and M.-X. Wang, J. Org. Chem., 2009, 74, 6077. CrossRef
41. A. Alex, B. Larmanjat, J. Marrot, F. Couty, and O. David, Chem. Commun., 2007, 2500. CrossRef
42. F. Couty, O. David, B. Larmanjat, and J. Marrot, J. Org. Chem., 2007, 72, 1058. CrossRef
43. T. Masuda, A. Chinone, and M. Ohta, Bull. Chem. Soc. Jpn., 1970, 43, 3287. CrossRef
44. B. Alcaide, P. Almendros, C. Aragoncillo, and N. R. Salgado, J. Org. Chem., 1999, 64, 9596. CrossRef
45. F. Outurquin, X. Pannecoucke, B. Berthe, and C. Paulmier, Eur. J. Org. Chem., 2002, 1007. CrossRef
46. F. Durrat, M. V. Sanchez, F. Couty, G. Evano, and J. Marrot, Eur. J. Org. Chem., 2008, 3286. CrossRef
47. F. Couty and M. Kletskii, J. Molec. Struct: THEOCHEM, 2009, 908, 26. CrossRef
48. S. Dekeukeleire, M. D'Hooghe, K. W. Tornroos, and N. De Kimpe, J. Org. Chem., 2010, 75, 5934. CrossRef
49. B. Drouillat, F. Couty, O. David, G. Evano, and J. Marrot, Synlett, 2008, 1345. CrossRef
50. M. Vargas-Sanchez, F. Couty, G. Evano, D. Prim, and J. Marrot, Org. Lett., 2005, 7, 5861. CrossRef
51. J. A. Vanecko and F. G. West, Org. Lett., 2005, 7, 2949. CrossRef
52. F. G. West, K. W. Glaeske, and B. N. Naidu, Synthesis, 1993, 977. CrossRef
53. T. M. Bott, J. A. Vanecko, and F. G. West, J. Org. Chem., 2009, 74, 2832. CrossRef
54. Y. Gnas and F. Glorius, Synthesis, 2006, 1899. CrossRef
55. F. Couty, B. Drouillat, and F. Lemee, Eur. J. Org. Chem., 2011, 794. CrossRef
56. W. Van Brabandt, R. Van Landeghem, and N. De Kimpe, Org. Lett., 2006, 8, 1105. CrossRef
57. M. K. Ghorai, K. Das, A. Kumar, and A. Das, Tetrahedron Lett., 2006, 47, 5393; CrossRef M. K. Ghorai, K. Das, and A. Kumar, Tetrahedron Lett., 2007, 48, 4373; CrossRef M. K. Ghorai, K. Das, and A. Kumar, Tetrahedron Lett., 2009, 50, 1105. CrossRef
58. E. V. D. Anslyn and D. A. Dougherty, ‘Modern Physical Organic Chemistry,’ University Science, Sausalito, 2006.
59. F. Couty, F. Durrat, G. Evano, and J. Marrot, Eur. J. Org. Chem., 2006, 4214; CrossRef B. Drouillat, F. Couty, and V. Razafimahaleo, Synlett, 2009, 3182. CrossRef
60. D. Crépin, J. Dawick, and C. Aïssa, Angew. Chem. Int. Ed., 2010, 49, 620.
61. M. K. Ghorai, D. Shukla, and K. Das, J. Org. Chem., 2009, 74, 7013. CrossRef
62. M. Bera and S. Roy, J. Org. Chem., 2009, 74, 8814. CrossRef
63. W. Behnen, C. Dauelsberg, S. Wallbaum, and J. Martens, Synth. Commun., 1992, 22, 2143; CrossRef P. D. Rao, C. H. Chen, and C. C. Liao, Chem. Commun., 1998, 155. CrossRef
64. W. Behnen, T. Mehler, and J. Martens, Tetrahedron: Asymmetry, 1993, 4, 1413; CrossRef M. Shi and J.-K. Jiang, Tetrahedron: Asymmetry, 1999, 10, 1673; CrossRef J. Wilken, S. Erny, S. Wassmann, and J. Martens, Tetrahedron: Asymmetry, 2000, 11, 2143; CrossRef P. J. Hermsen, J. G. O. Cremers, L. Thijs, and B. Zwanenburg, Tetrahedron Lett., 2001, 42, 4243; CrossRef Z. Zhang, M. Li, and G. Zi, Chirality, 2007, 19, 802. CrossRef
65. L. Keller, M. V. Sanchez, D. Prim, F. Couty, G. Evano, and J. Marrot, J. Organomet. Chem., 2005, 690, 2306. CrossRef
66. M. P. Doyle, S. B. Davies, and W. Hu, Chem. Commun., 2000, 867. CrossRef
67. H. Kotsuki, H. Ikishima, and A. Okuyama, Heterocycles, 2008, 75, 757; CrossRef H. Kotsuki, H. Ikishima, and A. Okuyama, Heterocycles, 2008, 75, 493. CrossRef
68. C. Thomassigny, D. Prim, and C. Greck, Tetrahedron Lett., 2006, 47, 1117. CrossRef
69. L. Menguy and F. Couty, Tetrahedron: Asymmetry, 2010, 21, 2385. CrossRef