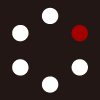
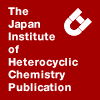
HETEROCYCLES
An International Journal for Reviews and Communications in Heterocyclic ChemistryWeb Edition ISSN: 1881-0942
Published online by The Japan Institute of Heterocyclic Chemistry
e-Journal
Full Text HTML
Received, 31st July, 2011, Accepted, 3rd February, 2012, Published online, 14th February, 2012.
DOI: 10.3987/REV-11-SR(P)8
■ The Hydrogenation of Heterocyclic Calix[4]arenes, a Transformation Leading to Novel Macrocyclic Ligands
Guillaume Journot, Christopher R. Jones, Valeria Blangy, and Reinhard Neier*
Department of Chemistry, University of Neuchâtel, Avenue de Bellevaux 51, Case Postale 2, CH-2000 Neuchâtel, Switzerland
Abstract
The simple and efficient synthesis of calix[4]furan and calix[4]pyrrole has been known for more than 125 years. The connectivity of the heterocyclic calix[4]arenes is identical with the skeleton of the «pigments of life». The chemical properties, especially the ability to form complexes with transition metals, are totally different when comparing the artificial systems with the natural products. A direct way to confer metal binding capabilities to compounds derived from heterocyclic calix[4]arenes is the hydrogenation. We review the known hydrogenation reactions of calix[4]furan and calix[4]pyrrole and present our own work in this field. A short description of the metal binding process of our new calix[4]pyrrolidines (= the fully saturated calix[4]pyrrole) with copper will be discussed as a typical example of the metal binding capacities. The new calix[4]pyrrolidines can be structurally classified as new stiffened macrocyclic crown ethers or as saturated analogues of the porphyrin derived «pigments of life».INTRODUCTION
CONTEXT
« What I cannot create, I do not understand ». (Richard Feynman on his blackboard on the date of his death 1988)
The success of chemists disclosing more and more complex structures of vital natural products and their activity changed profoundly our understanding of important biological processes. In parallel a huge effort went into synthesizing and discovering new, “purely synthetic” pharmaceutics as new efficient drug candidates. The scientific question driving all these efforts is the understanding of the biological world: How are all these beautiful structures functioning? When and how did these structures evolve? And the final challenge: are we capable of deducing the “rules” so as to design new active structures on the drawing board?
Organic chemistry from its inception has been intimately linked to our understanding of the processes of life. The development of organic chemistry can be traced back to the isolation, detection and structure determination of important active natural products.
For more than one hundred years the determination of the structures of natural products was one of the fundamental activities which determined the progress of organic chemistry and in parallel of our understanding of the fundamental processes of life (Figure 1). The experimental approach and to some extent the importance of the classic natural products chemistry changed with the arrival of efficient spectroscopic methods. The “art” of natural product determination by chemical degradation and correlation was replaced by a more systematic analytical approach. Replacing the tedious process of chemical structure determination by spectroscopic measurements allowed much faster progress and gave a higher degree of certainty to the proposed structures.12
However structure determinations based on spectroscopy cannot furnish information on the reactivity or the chemistry of a new natural product. Before the advent of spectroscopic methods this information was gradually accumulated during the process of chemical structure determination. The analytical part of a modern natural products project has been highly automatized. The goal is to achieve an efficient high throughput and a database driven identification of the isolated structures. Chemical manipulations are often limited to the preparation of the samples. These developments of modern analytical technology have induced a radical change. The inherent connection between chemical and biological reactivity and structure determination has been lost. Libraries of natural products have been determined without having acquired knowledge about the use and the properties of the structures. Natural products chemistry has become more similar to medicinal chemistry in this respect. Both approaches can be described as a search of biological activities starting from a library of compounds. The difference being that in natural products chemistry the library of compounds is isolated from natural sources, whereas in medicinal chemistry the library is synthesized by chemists or by machines.
Bioorganic chemistry has become an important complement to the analytical approach trying to fill the gap between a library of compounds and its activity either of the individual compounds or of a mix of compounds.12-15 In a broader sense the main challenge for chemistry has stayed the same over the years independent of the instruments and methods available. The challenge of chemistry has been and will for the foreseeable future be: discover and describe the structural laws of life. Compiling the design principles and understanding the correlations between chemical structures and their functions should enable us to design new active compounds on the drawing board from scratch.
«PIGMENTS OF LIFE» AND CALIX[4]PYRROLES
Many important processes of life like the transport of electron, oxygen, ion and photosynthesis depend on the presence of metal complexes of specific macrocyclic ligands.16-24 These metal complexes have been called «pigments of life» thereby indicating the fundamental roles by these metal complexes (Figure 2).25-32
The macrocycle of the «pigments of life» is formed in two elegant and efficient condensation reactions.33-36 Uroporphyrinogen is the last common intermediate in the biosynthesis of the «pigments of life» (Scheme 1). The first step is the condensation of two molecules of δ-aminolevulinic acid (δ-ALA) forming one molecule of porphobilinogen (PBG) which is catalyzed by a single enzyme.31,32,37-40 The second transformation, where 4 molecules of PBG are condensed to the macrocyclic uroporphyrinogen III (Uro III) requires the presence of two enzymes working in sequence.27,29,30 The first enzyme the porphobilinogen deaminase catalyzes the oligomerization to a linear precursor. The second enzyme the uropophyrinogen III synthase cyclizes the linear precursor and concomitantly inverts the D-ring. Finally it is worth mentioning, that the formation of uroporphyrinogens from PBG has been imitated in the test tube just by heating an acidic solution of PBG.41-48
The uroporphyrinogen chromophore, the first macrocyclic structure of the biosynthesis, does not form Werner complexes with transition metals easily. Forcing conditions have to be applied to achieve the incorporation of metals into the macrocyclic structures and often further transformations are induced under these conditions.49-55 Most of the natural tetrapyrrolic macrocycles derived from uroporphyrinogen III acquire the capacity to complex metals by oxidising the chromophore part of the ligand and by simultanously tautomerizing the double bonds of the chromophore (see Figure 3).14,56-60 Vitamin B12 and factor F430 are exceptions as their chromophore are formed by reductive processes starting from Uro III. The four nitrogen atoms of the pyrrole rings in the uroporphyrinogens are arranged in a pre-structured array, but the free electron pairs are not available for complexation as they are part of the aromatic heterocyclic pyrrole rings. The oxidation of an urophorphyrinogen chromophore is chemically a favorable process thermodynamically favored by the creation of conjugated aromatic systems. For the organisms biosynthesizing the «pigments of life» it is of advantage that uroporphyrinogen does not capture metal ions. The presence of “unwanted” and potentially dangerous metal complexes can thus be avoided. It is a question of precaution to create the highly coloured and reactive «pigments of life» only where and when they are needed. A way to circumvent these “protective measures” is used in photodynamic therapy, where large quantities of the unwanted protopophyrin IX are accumulated (PpIX).61,62
SYNTHETIC NITROGEN CONTAINING MACROCYCLES
Synthetic nitrogen-containing macrocycles like triazacyclononanes, cyclens, cyclams and obviously non-natural porphyrins have been reported in the past (Figure 4). The structures of the synthetic ligands are characterized by short aliphatic chains of two or three methylene groups linking three or four nitrogen atoms. The cyclams and the porphyrins share the same “distance” between the two pairs of nitrogen atoms: a linker composed of three carbon atoms. The other two pairs are connected by a shorter ethylene linker (Figure 4). The chemical, catalytic and structural properties of these synthetic systems have been studied thoroughly.63-65 Metal complexes of aza-macrocycles and their derivatives have been applied as sensors, contrast agents, in the detection and in the destruction of cancer cells or as oxidation and bleaching catalysts.66-75 Azamacrocycles are of great importance for studies of the mechanism of enzyme catalyzed reactions. Spectacular results have been obtained in experiments using simpler artificial systems to mimic the active site of elusive enzyme processes. The quest for high-valent iron or manganese oxo species is an important example of this type of studies.72,73,75,76 Other metal complexes were synthesized to obtain very useful transformations like selective oxidation of small hydrocarbons as methane, ethane or propane.72,77 The properties of the metal complexes of macrocyclic porphyrins have been compared to those of linear chelating ligands like salens and their derivatives.78-80 White and coll. have beautifully demonstrated that rigidifying a linear tetradentate ligand restrains the geometry of the metal complex to one single conformation leading to a more stable metal complex and thereby to a more efficient catalyst (Figure 5a).81 In another example Ready and Jacobsen have improved the catalytic activity of a linear salen derivative by transforming it to a macrocyclic analogue (Figure 5b).82
BRIDGED AZAMACROCYCLES
Cyclens and cyclams can be formally obtained by macrocyclization of linear oligomer type precursors. Adding additional bridges to these azamacrocycles induces topological constraints and enhances the high complex stability. Reducing the conformational space available to macrocyclic ligands has been conceptionally pioneered by Jean-Marie Lehn in his seminal work on cryptands.83-85 For synthetic reasons the additional bridge is often introduced between the nitrogen atoms (Figure 6a and 6b). The availability of the methriol as starting material allowed an easy synthetic access to the macrobicycles of the type illustrated in Figure 6c. Bridged or “strapped” porphyrins have been synthesized with the goal to imitate the properties of natural heme containing enzymes and proteins. A huge effort has been dedicated to the synthesis of such macrobicycles and to studies of the properties of their metal complexes, especially in the context of mimicking the reversible binding of dioxygen to iron complexes.
Numerous examples and variations have been reported in the literature. Only a few examples will be shown illustrating the structures, which have been made available.89,90 The synthetic strategies limited and directed the design of the structures synthesized. The typical approaches to such macrobicycles were: 1) The direct synthesis where a pre-existing cyclen or a cyclam is modified by alkylation or acylation; 2) The template-directed synthesis where a metal complex pre-arranges the precursor for the macrocyclization reaction; 3) The protection/deprotection approach, where the cyclization is effected step by step using protective groups to assure the selectivity; 4) The condensation approach, where a multicyclic hemiaminal is formed which is then reductively opened to leave a bridge in the molecule. The approach 1) suffers from the typical low yields observed for the macrocyclization reactions. The approach 2) is efficient but not very flexible. The approach 3) is often lengthy and complicated. The approach 4) is mechanistically complex but leads often to good results as far as efficiency and yield is concerned. In conclusion the synthetic methodologies available determine the structures, which can be studied and also the design of the structures proposed. Even if a specific compound is available by one of the strategies mentioned its use is severely hampered if the length of the synthesis or the low overall yield is prohibitive for a systematic use of the desired compound for further physicochemical and biomimetic studies. Ease and efficiency of a synthetic access to a desirable structure are crucial for the progress in science and understanding.
CALIX[4]PYRROLE
Adolf von Baeyer and his group were the first to isolate the meso-octaalkylporphyrinogen 1 more than 120 years ago by mixing pyrrole and acetone in the presence of a mineral acid (Scheme 2).91 This reaction was an extension of the systematic studies in von Baeyer’s group on condensation reactions using phenol and formaldehyde. Von Baeyer called the compound “acetonepyrrole”. The ease of the reaction and especially the ease of the isolation are remarkable. The product of the condensation between pyrrole and acetone precipitates out of the solution in pure, crystalline form. Despite these important factors, favoring the characterization and identification of the new material, the structure determination proofed to be tedious. It took a very long time until the structure of the compound was firmly established using a degradation approach.
Chelintzev et al. were the first to propose the correct structure in 1916.92 Hans Fischer had given the name α, β, γ, δ-octamethylporphinogen. This name suggested the close structural similarity of this compound to the porphyrins. It took almost forty years until Rothemund could definitively prove in 1955 the α,α’-linkage between the pyrrole rings included in the macrocyclic structure.93 Another forty years later the X-ray structure determination of this compound revealed the alternating conformations of the pyrrole rings in the solid state,94 where vicinal pyrrole rings were pointing in opposite directions. The pyrrole rings opposite to each other were nearly parallel forming a cavity. One pair of hydrogen bonds is pointing up and the other pair of hydrogen bonds is pointing down if the disk of the macrocycle is aligned horizontally. The X-ray crystal structures of these macrocycles binding anions like fluoride and chloride disclosed a significant change in the conformation of the macrocycle.94 In this halogenide binding complexes all the NH were directing towards the anion, adopting a cone like conformation similar to that observed for calixarenes.55
Based on this observation and on the similarity of the Rothemund procedure93 for the synthesis of calixarenes with the von Baeyer procedure it was proposed to change the name of these macrocycles once again from meso-octaalkylporphyrinogen to “calix[4]pyrroles”.94 The meso-octaalkylporphyrinogen are not bona fide precursors of porphyrins, an observation which legitimizes the proposed name change.
The calix[4]pyrroles were intensively studied for their capacity to function as anion sensor via the formation of four pyrrole NH-anion hydrogen bonds.95 In contrast to typical Werner type complexes, the nitrogen lone pairs of calix[4]pyrroles are part of the aromatic π system of the pyrrole ring and are therefore not available for complexation of metal species. The metallation of calix[4]pyrroles to give the tetra-anion allows the introduction of metal ions (Scheme 3).96 This procedure is experimentally challenging and requires the use of strong bases and strict exclusion of oxygen and water.
In summary calix[4]pyrroles have been known for a long time and are very easy to obtain and to isolate. Their chemistry has attracted attention only in the nineties of the last century, where the anion binding of the calix[4]pyrroles has been detected in the group of Sessler.94,97,98 This anion binding capacity can be attributed to the four directed H-bonds they are forming. During the same period the capacity of the calix[4]pyrroles to form metal complexes has been studied starting from the tetraanion by the group of Floriani.53,55,96,99 The transformations of these metal complexes is complicated. The reactivity is different from the one observed with the porphyrins, which form Werner complexes with great ease. The calix[4]pyrroles are very poor ligands, because the lone pairs of the four pyrrole rings are involved in the heteroaromatic ring and thereby the lone pairs are not available for metal binding.
MOTIVATION
The analysis of the reported reactivity of calix[4]pyrroles led to the question if the completely hydrogenated macrocycle can be obtained and what would be the chemical characteristics of this saturated system. The lone pairs of the completely saturated macrocycle should become available for metal binding (Figure 9).
A second topological correlation can be envisaged, if one compares the proposed totally reduced calix[4]pyrroles with the simple ligand having the same connectivity linking the nitrogen atoms to each other. The fully flexible ligand and its metal binding properties have been intensively studied (Figure 10). Bridged or “strapped” versions of this ligand have been extensively examined. Typically the bridges were introduced between the nitrogen atoms, for the ease of synthesis. In some cases the bridge connects opposite sides of the macrocycle. The synthesis of this type of macrocycle often starts with methriol as starting material leading to a macrobicyclic structure. We did not find reports on structures, where the additional cycles were added “externally” or in an “exocyclic” fashion to the basic macrocyclic structure. As in the cases reported in the literature, where the introduction of a bridge (=”endocyclic” ring formation) reduces the conformational flexibility, the addition of “exocyclic” rings will stiffen the skeleton and should thereby increase the thermodynamic stability of the metal complexes.
In contrast to most bridged ligands published so far the pentacyclic structure 5 possesses eight chiral centers. Due to the symmetry of the ligand not all combinations of the relative configuration of the eight chiral centers lead to separate diastereoisomers. If this would be the case a total of 256 stereoisomers should be obtainable. A total of 45 different stereoisomers of compound 5 can be drawn.100 The influence of the relative configuration of a specific stereoisomer of 5 on its capacity to act as a ligand for metal atoms is difficult to predict. If the structures with the constitution of 5 will become synthetically available the correlation between metal binding and the relative configuration of the chiral centers of the ligand can be studied. The proposed ligand 5 has symmetry properties, which ressemble to those of Nonactin a natural ligand for potassium. The tetrameric macrolide possesses an unusual S4 symmetry. Recent studies show that this specific relative configuration is a more efficient potassium cation binder than other optical pure artificial isomers synthesized for comparison purposes.101 Studies of the stereoisomers of 5 could contribute to our understanding of the influence of the relative configuration of the ligand on metal binding and complex stability.
KNOWN HYDROGENATIONS OF HETEROCYCLIC CALIX[4]ARENES
All the three simple homo heterocyclic calix[4]arenes (containing four identical aromatic heterocycles) are known: calix[4]furan 6, calix[4]pyrrole 1 and the calix[4]thiophene 8 (Figure 11). The synthetic access to the macrocycles 6 and 1 is easy, via the procedure pioneered by von Baeyer. The fully substituted calix[4]thiophene 8 is more difficult to obtain and the reported yields are low.93 The group of Vogel developed a method where the calix[4]furan 6 could be converted into the calix[4]thiophene 8 using hydrogen sulfide under acidic conditions.102-105 A recent publication reports on the efficient synthesis of highly substituted calix[4]furans and calix[4]thiophenes adding a heteroaryllithium compound to ketones and treating the products with N-iodosuccinimide to achieve the condensation to the macrocycle.106,107
The synthetically easiest way to the reduced macrocycles is the exhaustive hydrogenation of the corresponding heterocyclic calix[4]arenes. In principle the reduced compounds 5 and 7 should be available in two simple synthetic steps: 1. acid catalyzed condensation of the five membered heterocycle with acetone followed by 2. heterogenous catalytic hydrogenation. Only the transformation of the calix[4]furan 6 to the calix[4]tetrahydrofuran 7 has been reported in the literature before our own studies.
HYDROGENATION OF CALIX[4]FURAN 6
The synthesis of calix[4]furan 6, the starting material for the heterogenous reduction, was first described by Ackman et al. in 1955,106,108-110 almost 80 years after the first publication of von Baeyer describing the synthesis of calix[4]pyrrole 1. Historically the first preparation of a calix[4]furan was already reported in 1906 by Hale et al.,111 when they treated ethyl 2-furoate with ethyl magnesium iodide. Based on the limited analytical data available at that time, they incorrectly proposed 2-(pent-2-en-3-yl)furan as the structure of the product obtained (Scheme 4).
The reaction conditions in 1955 and later are similar to the conditions used for the synthesis of calix[4]pyrrole 1, however the yields are generally lower (Scheme 5). The best conditions reported are using a large excess of LiClO4 to accelerate the reaction.112,113 In 1985 de Sousa Healy and Rest published a systematic study of the role of metal salts in the macrocycle synthesis by furan-ketone condensation.114 Alkaline-earth metal salts gave 6 in generally good yields, typically between 14 and 32% for Ca(ClO4)2. The salts of transition metals like chromium, manganese, iron, cobalt, nickel, copper and zinc gave good to excellent yields, situated between 12 and 33% of the macrocycle 6. In all cases the best yields were obtained when perchlorate was used as the anion. They also studied the influence of the type and amount of acid, the amount of water present and the reaction time. The authors studied also the complexation of the macrocycle 6 with the metal salts used. In contrast to the publications of Chastrette, the authors came to the conclusion that the template effect is not the major factor for the outcome of the condensation. They prefer to attribute the increase of yield by the pH change induced in the presence of the metal salts.
The recent report by Lee et al.106 described the efficient synthesis of sterically demanding calix[4]furans e.g. 10 and calix[4]thiophenes which were so far not accessible (Scheme 6). The method is based on the selective direct C-H metallation of furan in the α-position followed by reaction with a ketone as electrophile. The benzylic alcohol obtained is not isolated but converted into arylalkenes by dehydratation. The arylalkenes were tetra-cyclized with the aid of iodonium ion from N-iodosuccinimide. The catalytic hydrogenation of the calix[4]furan 6 was first reported by Kobuke et al. in 1976,115 who made a systematic study of the transport capacities of macrocyclic tetrahydrofuran-containing structures through liquid membranes. Kobuke et al. used ruthenium on carbon as catalyst and had to apply 120 atm H2-pressure and 190 °C for 6 h obtaining 73% yield of the totally reduced macrocycle 7.
Slightly different conditions for the reduction were reported by Rest et al. in 1976,116 by Chastrette113 and by Wiegers and Smith.107 When palladium on carbon was used as catalyst the pressure needed for the reduction varied from 110 atm to 170 atm at a temperature of 105 °C. The yields reported were consistently between 57 and 72%. In contrast to the previous authors de Sousa Healy and Rest114 were not able to carry out the reduction in ethanol. Using THF as solvent, Raney Ni as catalyst and similar conditions of pressure and temperature as those reported by previous authors they isolated and separated a mixture consisting of 38% of the totally reduced macrocycle 7 and 18% of the half-reduced compound 11 by successive crystallization (Scheme 7).
In the publication by Van Beylen et al.,100,117 which appeared in the same year, the authors reported the successful hydrogenation under considerably milder conditions. Hydrogenation was successful at room temperature and under atmospheric H2 pressure (Scheme 8).
Under these conditions at least two different disastereoisomers were formed. The authors could separate the two diastereoisomers 7a and 7b in a 70 : 30 ratio. Both diastereoisomers crystallized and their structures in the solid were determined by X-ray diffraction. Characterization of the two diastereoisomers by additional analytical and spectroscopic data and the detailed experimental description are unfortunately missing. Ten years later the authors described a detailed spectrophotometric study of the metal complexation of these two new ligands, without giving more details describing the ligands.118
THE STRUCTURES AND PROPERTIES OF THE CALIX[4]TETRAHYDROFURANS 7
The interest in the calix[4]tetrahydrofurans 7 was stimulated by the discovery of the crown ethers by Pedersen, Cram and Lehn.85 An additional stimulus came from the discovery of the capability of nonactin to form complexes with alkali cations, showing a remarkable preference for potassium over sodium ions (Figure 12).119 The X-ray structures of the two diastereoisomers 7a and 7b were reported by von Beylen et al. (Scheme 7 and Figure 13).100,117 The conformations of compounds 7a and 7b resemble each other and are quite similar to the conformations found in the unsaturated heterocyclic calix[4]arenes, calix[4]furan 6 and calix[4]pyrrole 1, namely a 1,3-alternate conformation.117 A dramatic change of the conformation is observed when the lithium picrate complex of the macrocycle 7a is studied.
The complex crystallized out from a mixture of lithium picrate and the calix[4]furan 6 in chlorobenzene containing a small amount of THF. The four oxygen atoms of the furan rings are almost in a planar arrangement. The metal binding changes the overall appearance of the macrocycle. The global form of the macrocycle becomes bowl shaped. The central lithium cation is in a position slightly out of the plane deviating towards the picrate, which is in binding distance (Figure 14). The bowl shape creates two distinctively different faces: a concave face limited by the four axial methyl groups and a convex face open to the solvent. As a consequence of the overall shape of the macrocycle four of the methyl groups are in equatorial positions whereas the other four methyl groups are in axial positions forming a tight cleft seriously restraining the access to the metal atom.
The early studies of Kobuke et al. showed that the reduced calix[4]tetrahydrofuran 7 was capable of extracting alkali metal cations into organic media, typically chloroform.117 Lithium and sodium picrates could be extracted from aqueous to the chloroform phase using the macrocycle 7. Transport rates through liquid membranes were determined as well. The selectivity measured in transport rates was fastest for sodium cations with a 2 : 1 preference compared to lithium cations and at least a 6 : 1 preference compared to the transport rate of potassium cations. Wiegers and Smith isolated the complex formed by mixing of one equivalent of LiAlH4 and one equivalent of calix[4]tetrahydrofuran 7 in THF.107 They obtained a crystalline complex which was characterized by its CHN analysis, compatible with a complex containing one equivalent of THF. Using increasing quantities of the calix[4]tetrahydrofuran 7 in the presence of LiAlH4 for the reduction of camphor decreased the rate of the reduction. The influence of the macrocycle 7 on the reduction is however moderate compared with the influence of cryptands, which stopped the reduction completely.120-122 De Sousa, Healy and Rest observed characteristic changes in the NMR signals on the complex formation between calix[4]tetrahydrofuran 7 and lithium salts, like LiI, LiSCN and LiClO4. Van Beylen et al. determined the rate of complex formation and the binding constants separately for the two diastereoisomers 7a and 7b.118 Spectrophotometric studies showed that both diastereoisomers formed complexes with lithium picrate in different solvents like THF, tetrahydropyran, dioxane, diethyl ether or chlorobenzene. The complex formation was slow for both diastereoisomers. The authors attribute the sluggishness of this process to the importance of the conformational changes needed for the complex formation. The complexes formed from the all cis-diastereoisomer 7a were considerably more stable than the complexes formed from 7b. The small changes in the position of the absorption band on complexation are compatible with the presence of a stretched contact ion pair. For the complex with the other diastereoisomer 7b more important bathochromic shifts are observed. This is compatible with the formation of a solvent-separated ion pair.
HYDROGENATION OF CALIX[4]PYRROLE
To the best of our knowledge only one report on the hydrogenation of calix[4]pyrrole 1 has been published in 1955 before our own work published in 2009 (Scheme 9).93 The goal of the study by Rothemund and Gage was to ascertain the structure of calix[4]pyrrole 1 called at the time acetonepyrrole. The conditions used for the hydrogenation were extremely harsh. The objective of this transformation was securing the position of the linkage connecting the neighboring pyrrole rings into the macrocycle. The main result of this degradation experiment was the proof of the position of the links in the 2 and 5 positions of the pyrrole exclusively. Almost 70% of the bridging atoms were accounted for.
Conclusions:
The publication of Rothemund and Gale is the only experiment reported in the literature describing trials to hydrogenate calix[4]pyrrole 1. It is therefore the only valuable source of information if one is interested in the hydrogenation process. Three types of conclusions can be drawn from the described experiments: 1) Experimental conditions for a successful hydrogenation; 2) Difficulty of the hydrogenation of alkyl substituted pyrrole rings; 3) Relative rate of hydrogenation of pyrrole rings compared to the rate of the benzylic hydrogenation.
The first conclusion concerns the experimental conditions. The extreme harshness of the hydrogenation conditions is striking. In a second hydrogenation experiment the authors use even higher pressures and only a slightly lower temperature: 204 atm H2 at 215 °C. Under these strenuous conditions it is somewhat of a surprise that the material balance is relatively good. To account for 69.4% of the bridging atoms is an excellent experimental result considering the aggressive conditions used and the difficulty of the separation process. The authors used only distillation and acid base extractions. The good material balance is clearly a testimony for the quality of the experimental work of the authors.
The second conclusion concerns the inherent difficulty to reduce dialkyl substituted pyrroles. The process must be quite difficult at least under the conditions described by the authors. The experimental conditions are extreme. Pressure and temperature are at the limit of what can reasonably and safely implemented in a typical laboratory. It is worth mentioning that the authors used similarly strenuous conditions for the hydrogenation of the 2-isopropylpyrrole and for the 2,5-diisopropylpyrrole to the corresponding 2-isopropyl-pyrrolidine and the 2,5-diisopropyl-pyrrolidine (Scheme 10). The goal of these hydrogenations was to prove the structures of the compounds that were obtained by degradation by an independent synthetic approach. Obviously the hydrogention of simple alkyl substituted pyrroles as well as the hydrogenation of calix[4]pyrrole require very harsh conditions. One can rationalize these extreme conditions by the difficulty to reduce an electron rich heterocycle and by the steric hindrance imposed by the substituents at positions 2 and 5. The temperatures and pressures needed for the hydrogenation remain a surprise, if one compares the heats of hydrogenation for furan (14.9 kcal/mol), pyrrole (17.1 kcal/mol) and thiophene (18.5 kcal/mol).123 The estimates for the resonance energies of furan (16.3 kcal/mol), pyrrole (21.5 kcal/mol) and thiophene (29.2 kcal/mol) derived from the energy of combustion indicate a more important thermodynamic difference than the figures reported for the hydrogenation. The considerable differences in the resonance energy might be compatible with the differences observed for the hydrogenation.124
The last conclusion concerns the relative rate of hydrogenation of the pyrrole rings compared to the rate of the benzylic hydrogenolysis. Rothemund and Gale isolated and identified only ring opened products. One has to mention however that they report some supposed decomposition during their first distillation, which might be due to the presence of oligomeric or macrocyclic products: 23.6% of the material was present as charred residue, not distillable under the conditions used by the authors. More conclusive is the observation that the relative amount of the pyrrolic degradation products isolated is about ten times as high as the amount of the totally hydrogenated corresponding pyrrolidines (Scheme 9). This observation is compatible with a considerably faster benzylic hydrogenolysis than the hydrogenation of the aromatic pyrrole rings. Even in the dipyrromethane type compound (Scheme 9) the two pyrrole rings are fully aromatic. The amount of the dipyrromethane type compound is almost a factor of eight smaller than the amount of monocyclic compounds isolated under these hydrogenation conditions. Also this observation is consistent with a kinetically faster benzylic hydrogenolysis.
In summary: Harsh conditions are needed for a successful hydrogenation of calix[4]pyrroles. The reasons for these extreme conditions are not easily rationalized based on the thermodynamic data available. Under the conditions applied the benzylic hydrogenolysis seems to be kinetically faster than the hydrogenation of the pyrrole rings. The challenge is therefore to find experimental conditions where the ring opening and thereby the benzylic hydrogenolysis is slowed down and/or to find conditions where the hydrogenation of the pyrrole rings is accelerated sufficiently to allow the isolation of the reduced macrocycle.
OUR RESULTS ON HYDROGENATION OF CALIX[4]PYRROLE
FIRST SUCCESS
To the best of our knowledge the controlled reduction of calix[4]pyrrole (1) has never been reported before the publication of our own work. We embarked on a systematic research program with the goal to develop methods for the reduction of calix[4]pyrrole (1).125 We avoided conditions known to facilitate ring opening processes. As the synthesis of the macrocycle 1 is catalyzed by the presence of strong acids, we reasoned that the acidic reaction conditions leading to the macrocycle should also be able to open the macrocyclic structure. We therefore chose neutral conditions. Based on the reports of Lunn,126 we tested Raney Nickel W2 as catalyst in a THF as solvent. Using freshly prepared Raney Nickel we isolated unreacted starting material applying temperatures up to 120 °C and hydrogen pressures up to 100 atm. Applying temperatures of 150 °C to 220 °C and pressures finally reaching 150 atm partial destruction of the macrocycle 1 was observed, without any hint at the formation of a reduction product. Trials to hydrogenate the macrocycle 1 in ethanol with Pd/C also yielded solely recovered starting material. Following a literature precedence we used Rh/C and acetic acid as solvent as reported in the publication.127 To our surprise no hydrogenation but also no destruction of the starting material occurred using conditions going up to 70 atm and 65 °C. Using a stronger acid such as trifluoroacetic acid led to complete destruction of the macrocycle 1. Using acetic acid as solvent for the hydrogenation probably transforms small amounts of the pyrrole rings into the protonated imminium form, destroying the aromaticity and thereby facilitating the hydrogenation. Even if these series of hydrogenations using Rh/C as catalyst did not achieve our goal, they paved the way to new experimental conditions. The recovery of the starting material proved that protonating conditions can be applied to the calix[4]pyrrole (1), avoiding the detrimental ring opening reaction at least to a large extend. We started testing hydrogenation using acetic acid either as additive or as solvent. This change of the strategy led to the discovery of the first reduction process of the macrocycle 1.125 Using either mixtures of acetic acid in ethanol or pure acetic acid as solvent and catalysts like Pd/C, Rh/C, PdO, PtO2 and Pd/BaSO4 partial reduction of the macrocycle 1 could be observed. The hydrogen pressure for these reactions varied slightly between 80 atm and 90 atm and the temperatures used were between 50 °C and 70 °C. Using higher temperatures under these conditions led to a decrease in the isolated yield, which we attributed to an increase in the rate of the ring opening reaction.
Based on these observations we proceeded to a first optimization of the hydrogenation conditions. The hydrogenation of calix[4]pyrrole under 85 atm of hydrogen with a catalytic amount of palladium on charcoal (13 mol% Pd compared to the macrocycle 1) in acetic acid as solvent at 50 °C for 16 hours, provided a mixture of two diastereoisomers of the half-reduced form of the macrocycle 12a and 12b (Scheme 11).128 The structures were rigorously assigned based on spectroscopic data and finally with the help of X-ray analysis of the crystals.129
As in the case of the hydrogenation of the calix[4]furan only two of the seven possible stereoisomers were identified and characterized namely 12a and 12b (Figure 15).
FIRST ISOLATION OF THE TOTALLY REDUCED CALIX[4]PYRROLIDINE 13
The only known reduction of a heterocyclic calix[4]arene, the hydrogenation of calix[4]furan, gave the completely saturated macrocycle (Scheme 8).117 In contrast to these reports in the literature, the hydrogenation of calix[4]pyrrole 1 gave in our hands the partially reduced macrocyclic structure 12. The initial attempts to achieve complete saturation of the calix[4]pyrrole macrocycle failed; no traces of a completely hydrogenated product could be detected. The degradation of the macrocycle was observed when temperatures higher than 150 °C were used. Increasing the hydrogen pressure to 150 atm whilst maintaining the temperature led also to degradation. By varying the type of catalyst (Raney nickel, rhodium on alumina, platinum on carbon, palladium oxide) the solvent (THF, EtOH/AcOH, dioxane, dioxane/AcOH, MeOH) or changing the quantities and the type of acid added (TFA, HCl, HBr) either no reaction occurred or only degradation was observed. After a long fruitless period we were able for the first time to observe the product of the total reduction of calix[4]pyrrole. We had to use harsh but carefully adapted conditions for the catalytic hydrogenation.129 Under these conditions the calix[4]pyrrole (1) was transformed mostly into the partially reduced macrocycles 12a and 12b. Only a very small amount of the totally reduced product 13 could be isolated (initial yields were below 5%) (Scheme 12).
In a first intensive effort we optimized the isolation of the new macrocycle 13 and fully characterized its structure. As mentioned before, using temperatures considerably above 100 °C inevitably led to the destruction of the material. More surprising for us was the fact that increasing the pressure to 150 atm led also to the degradation of calix[4]pyrrole 1. At this stage the conditions for the reduction could not be varied and the yield of the totally reduced macrocycle 13 seemed to be limited to a value between 5% and 10%. We were clearly in the regime of a formation (yields below 20%) and not of a ”real” synthesis. We therefore concentrated our efforts on obtaining reproducible reaction conditions and a reasonably easy isolation procedure. As a consequence of this first optimization the following harsh conditions were systematically applied to create sufficient quantities of the macrocycle 13 for further studies: 100 atm of hydrogen in pure acetic acid at 100 °C for 24 h using 10% palladium on charcoal.
HYDROGENATION OF STRUCTURALLY DIFFERENT CALIX[4]PYRROLES 14 AND 15
In order to check if our conditions could be successfully applied to other calix[4]pyrroles we tested the calix[4]pyrroles 14 and 15. We were able to isolate the partially reduced products 16a, 16b and 17a and 17b in similar yields as for the simpler calix[4]pyrrole 1 (Scheme 13). The recovery of characterized material is relatively poor. We attribute this to the sensitivity of the macrocycles 14 and 15 to the acidic reaction. Only 22% combined yield of 16a and 16b were recovered from the calix[4]pyrrole 14. The amount of the half-reduced macrocycles 17a and 17b was slightly better when starting from compound 15. A total of 30.6% could be isolated. Degradation of the starting material largely accounted for the remaining percentage. In both cases the all-cis compounds 16a and 16a were again the major products of the hydrogenation, with a diastereomeric ratio of: 16a : 16b = 84 : 16 and 17a : 17b = 64 : 36.
STUDIES AND DISCUSSION OF THE MECHANISM
At this stage a series questions arose:
1) Why is the hydrogenation stopping at the stage of the bis-reduced macrocycles of type 12?
2) Why did we identify only the alternating bis-reduced macrocycles of type 12? In all our products the pyrrolidine rings and the pyrrole rings alternate. We have found no signs of products where the two pyrrolidine rings are contiguous.
3) Why is the yield of the totally reduced macrocycle 13 so low?
4) Are the partially reduced macrocycles of type 12 intermediates on the way to the totally reduced macrocycle 13? Or alternatively is the compound 13 formed in a different sequence and if this is the case, what is the sequence?
We propose the following mechanistic hypothesis for the hydrogenation of the calix[4]pyrrole 1, which seems to us to rationalize most of the questions and remarks mentioned above. As the presence of acetic acid is compulsory for a successful hydrogenation, we assume that the reduction is starting from the small quantity of protonated calix[4]pyrrole I (Scheme 14). The protonation of one of the pyrrole rings destroys the aromaticity of the heterocycle and creates an electron poor π-system, avoiding the necessity to reduce an electron rich pyrrole. If this assumption is correct, the central intermediate for the hydrogenation is the protonated form I. The central intermediate I should be amenable to efficient hydrogenation giving an intermediate of the structure II. Alternatively, the hypothetical intermediate I can undergo ring opening to give the intermediate III. This reaction is the opposite reaction to the final step in the synthesis of calix[4]pyrrole 1. In contrast to the hydrogenation process forming II, the ring opening will be reversible. However, it is highly probable that if starting from an intermediate like III, a plethora of side reactions are accessible: further degradation, condensation and/or reduction processes on ring opened linear compounds. Assuming this tentative mechanistic proposal to be accurate, the challenge of the hydrogenation is to ensure, that the hydrogenation of the central intermediate I is faster than the side reactions that become accessible by ring opening to form III. In view of this hypothesis, the reaction conditions applied and the difficulties of the process make perfect sense. High hydrogen pressures are needed to speed up the hydrogenation. Increasing the temperature too much favors the ring opening process as the entropy is increased and leads to degradation. If this analysis is true the hydrogenation process is a walk on razor’s edge between the need to increase the rate of the desired hydrogenation and the necessity to avoid the ring opening process leading to uncontrolled destruction.
If the hydrogenation process of the pyrroles requires initial protonation we can rationalize the isolation of the partially reduced macrocycles of type 12 and give a tentative answer to questions 1 and 2 (Scheme 15). The protonation of the macrocycle 12 leads to an intermediate of type IV. This intermediate IV is “protected” against ring opening, or at least the ring opening reaction is considerably slower than the ring opening of compounds where at least one of the neighbors is a pyrrole ring like in I or in VI. The major postulated pathway to destruction is no longer accessible for compound 7. If intermediates of the type V should be present, the situation is completely different. If the intermediate V is protonated to form VI, this protonated form still is amenable to ring opening and thereby to destruction via compound VII. For simplicity we have omitted the protonations of the pyrrolidine rings at this point in our discussion (Scheme 15). As a consequence of this proposal, the isolation of the partially reduced macrocycle of type 7 can be interpreted as the isolation of the most stable regioisomer under the acidic reaction conditions.
The hydrogenation of the calix[4]pyrrole 1 transforms a neutral compound into a dibasic macrocycle of the type 12 and into a tetrabasic macrocycle of the type 13. The partially and totally reduced macrocycles will be present in their N-protonated form under the reaction conditions (Scheme 16).
For each pyrrole ring, which is hydrogenated, a positive charge is created by protonation of the formed pyrrolidine. The hydrogenation of 7 would therefore demand the additional protonation of one of the two remaining pyrrole rings of a molecule which is already bisprotonated (7 + 2H)2+ (Scheme 16). As a consequence of this hypothesis, the reduction of the calix[4]pyrrole 1 would become more difficult as the hydrogenation is progressing: in the first reduction one has to protonate a pyrrole starting from the neutral compound 1 whereas in the last hydrogenation one would need to protonate a pyrrole starting from a trication. The mechanistic hypothesis predicts therefore that the hydrogenation becomes more and more difficult. This rationale is at least a partial answer to question 3.
As one of the first ways of trying to improve the yield of the reduction to the totally reduced compound 13, we tried to achieve the total reduction of compounds of type 12. Using mixtures of 12a and 12b or using pure 12a as starting material and submitting them to identical hydrogenation conditions as the ones which allow us to obtain small quantities of 13 starting from the calix[4]pyrrole 1, we have not been able to obtain evidence for the formation of the totally reduced macrocycle 13. Based on our mechanistic hypothesis indicating that the hydrogenation should become more and more difficult as we progress in the reduction process, we tried to use harsher conditions starting with 12. The results of many trials, where we modified the catalyst, the temperature or the pressure were that we either isolated unchanged starting material or we observed degradation. The limits between no reaction and degradation were similar to those observed for the initial process (temperatures of 150 °C and higher, and hydrogen pressure of 150 atm). During this long process we achieved one encouraging result. Using a specific sample of palladium on carbon from Fluka we were able to hydrogenate small but reproducible quantities of macrocycle 12a to the fully reduced macrocycle 13.130 When we had to order a new batch of palladium catalyst from the same provider the reduction did not work anymore. Confronted with this irreproducibility we tested more than twenty different batches of diverse palladium catalysts from different providers without success. So far we have not been able to identify the factors which allow the hydrogenation of 12a to take place or that prevent the hydrogenation. The studies of this important fact are still intensively ongoing.
Our tentative mechanistic proposal attributes a considerable importance to the protonation state of the intermediates. So we changed the strength of the acid used for the hydrogenation trials starting from 12. We tried to use stronger acids like trifluoroacetic acid but also mineral acids like HCl or H2SO4 or 2 : 8 mixtures of these mineral acids in acetic acid. Unfortunately, these trials did not give any hints at the formation of the reduction product 13. Using the standard conditions no degradation but also no transformation was observed. The partially reduced macrocycle 12a proved to be quite stable, but also very resistant towards further reduction. As a consequence of these experiments we can exclude 12a as a free intermediate on the way to the totally reduced compound 13 at least with the catalysts we use now. We cannot exclude 12a as an intermediate for the special sample of palladium catalyst we used in the above mentioned series of experiments. In view of these results two mechanistic proposals seem to us the most probable. The hydrogenation to 13 can either pass via an intermediate of the type V (Scheme 15b) or the complete hydrogenation occurs without releasing intermediates from the catalyst.
OPTIMIZATION OF THE HYDROGENATION
We decided to optimize the hydrogenation using our “best” conditions as the starting point. Andrea Gualandi developed a very efficient GC method using n-eicosane as internal standard, which gave us good and reliable analytics. With this method in hand we could make a thorough study of the transformation and we became independent of the lengthy isolation process. We fine-tuned the reaction conditions (Scheme 17).130
As a first important result Andrea Gualandi could show that a third, so far undiscovered, isomer of the partially reduced macrocycle of type 12 is formed under these conditions: 12c. Compounds 12a and 12b could be characterized by their spectra and by X-ray diffraction of the crystals. The new diastereoisomer 12c did not crystallize and was tentatively characterized by its spectral data. The NMR spectra of 10c are lacking any element of symmetry. This data are compatible with the structure 12c. We are still working on the structure. If we can confirm our structure attribution this will be the first time we observed a trans-pyrrolidine ring as a product of the hydrogenation process.
A large number of different hydrogenation catalysts were tested by Frédéric Bruyneel, postdoc in our group, keeping the conditions (hydrogen pressure, temperature and solvent) for the process constant. A small selection of the numerous trials made in our group has been reported in our publication (Table 1 slightly modified).130 Heterogeneous catalysts supported on graphite were specifically prepared by the Savoia group because they had been applied very successfully to the hydrogenation of heterocycles (Table 1, Entry 5 – 7).130
For this comparison the Platinum group metals Rh, Ru and Pd were tested. All the catalysts used in Table 1 achieved good to very good transformation of the starting material with little degradation (81% up to 96% transformation). In all cases the set of reduction products was formed: 12a-c and 13. The ratio between the different diastereoisomers of the partially reduced products of type 12 depended on the metal used as catalyst and was only slightly dependent on the matrix (Table 1 compare entry 2, 3 with 7 and entry 4 with 5). In all but one case, entry 2, the totally reduced macrocycle 13 was the product with the lowest yield. Palladium on carbon 10% turned out to be the catalyst capable of producing the highest amount of the totally reduced macrocycle 13.
Palladium on carbon remained the best catalyst and we could obtain up to 16% of the totally reduced calix[4]pyrrolidine 13, which doubled the yield compared to our earlier experiments. Using the optimal conditions described above we carried out the hydrogenation on a gram scale. Taking advantage of the difference in solubility and pH behavior or the products of the hydrogenation we developed a method of separating the products by successive crystallization and thereby minimizing the use of chromatography for the separation.
THE X-RAY STRUCTURES OF CALIX[2]PYRROLIDINO[2]PYRROLE AND OF THE CALIX[4]PYRROLIDINE
NUMBER OF POSSIBLE STEREOISOMERS
The partially reduced calix[2]pyrrolo[2]pyrrolidine possesses 4 carbon atoms with 4 different substituents. The maximum number of possible stereoisomers should be 16. Due to the high symmetry of the compound only 7 different stereoisomers exist: 3 meso compounds (12a, 12b and 12e) and 2 pairs of enantiomers (12c, ent-12c, 12d and ent-12d) (Figure 12). So far we have only been able to isolate and characterize three stereisomeric structures: the two meso structures 12a and 12b and the racemate composed of 12c and ent-12c, which has been tentatively identified so far. The calix[4]pyrrolidine 13 posseses eight carbon atoms with four different substituents. The maximum number of stereoisomers is therefore 256. Only 45 stereoisomers can exist as has already been mentioned by van Beylen.117
STRUCTURES OF CALIX[2]PYRROLO[2]PYRROLIDINE 12a AND 12b
To ascertain the structures deduced from the spectroscopic data of the two partially reduced macrocycles 12a and 12b the determination of the structures in the solid state by X-ray diffraction was essential (Figure 16).129,130 Due to the high symmetry of these two meso-compounds the unequivocal assignment of the structures was delicate. The result of the X-ray diffraction enabled us to attribute the relative configurations, but more importantly the structures gave important hints to understand the properties of the calix[2]pyrrole[2]pyrrolidine structures 12a and 12b.
Both structures are characterized by a tight hydrogen bond network in the center, connecting the pyrrole N-H with the lone pair of the neighboring pyrrolidine nitrogen. In the case of 12a the network is a two by two network, where one pyrrole N-H is bound to one of the pyrrolidine neighbors, whereas the second pyrrole N-H is linked to the other pyrrolidine neighbor. The hydrogen bond network of 12b is more complicated. One pyrrole forms two hydrogen bonds to the two neighboring pyrrolidine rings. The bifurcated hydrogen bond emanating from this pyrrole is almost symmetric. In both macrocycles the hydrogen bond network forms an inner core, which fixes the conformation and thereby the properties of the molecules. The N-H from the two pyrrolidine rings in 12a are directed towards the same face of the macrocycle, whereas in 12b the N-H bonds of the pyrrolidine rings are pointing in opposite directions. The structures are very tight and access to the inner core is not easy, without breaking the hydrogen bond network. This fixed inner core determines by a sort of conformational coupling the arrangement at the outside of the macrocycles. For both molecules four of the eight methyl groups are arranged in a quasi equatorial position, whereas the four other methyl groups are arranged in pairs in quasi axial positions: two methyl groups are up and two methyl groups are down.
STRUCTURES OF CALIX[4]PYRROLIDINE 13
The structure determination of the calix[4]pyrrolidine 13 from the spectral data alone was easier. The high symmetry of the compound led to an extremely simple 1H- and 13C-NMR spectrum. These spectra together with the MS and the high-resolution MS did not leave any doubts about the structure. Crystallization proved to be difficult, but finally good crystals could be obtained (Figure 17).130
The solid state structure reflects the beauty of the symmetrical chemical structure 11. Once again the nitrogen atoms and the N-H bonds form a tight inner core. Due to the symmetry of the molecule this inner core is C4 symmetric. The arrangement of the methyl groups also reflects this C4 symmetry. Therefore 4 out of the 8 methyl groups are quasi equatorial. The other 4 methyl groups are quasi axial, but in contrast to the macrocycles 12a and 12b the 4 axial methyl groups are pointing all to the same face of the disk-like compound.
Looking at the molecule from the edge reveals additional, remarkable structural aspects of the calix[4]pyrrolidine 13 (Figure 18). The macrocycle 13 has a disk-like overall shape, creating two half spaces: one face is characterized by the four hydrogens bonded to the pyrrolidine nitrogens and the other face is forming a shallow cleft limited by the four quasi axial methyl groups. The four lone pairs of the nitrogens are arranged in an almost perfect square, directed towards the center of the macrocycle 1. If one compares the structure of the calix[4]pyrrolidine 13 with the structure of a porphyrin the similarities and differences of the two systems are stricking.
The porphyrins are flat sytems. The two faces of the disk-like molecule are identical. The porphyrins are characterized by an arrangement of four nitrogen atoms in a flat and square arrangement perfectly aligned for complex formation. The N-H bonds in the ligand are in the plane of the disk and engaged in an efficient network. The tautomerism allows quick exchange of the positions of the H-atoms. The ligand has to be deprotonated twice for the formation of metal complexes, thereby the H-bond donation is lost when a metal complex is formed. The prophyrins offer four lone pairs for an efficient σ-interaction with the metal. The aromatic 18 electron aromatic π-system offers the opportunity for π-back donation. The electronic properties on the metal can be tuned via the π-system of the ligand.
The macrocycle 13 has a disk-like overall shape as well. The four nitrogen atoms are in a flat and square arrangement, well aligned for complex formation. The two faces of the disk are clearly differentiated: one face is characterized by the N-H bonds directing away from the disk, the other face is characterized by a shallow cleft formed by the four axial methyl groups. However the four N-H bonds are orthogonal to the plane of the disk. The ligand can complex metals without deprotonation therefore the H-bond donating property remains intact when a metal complex is formed.
In summary our ligand 13 is geometrically very similar to the porphyrins, but electronically very different. The ligand 13 has inherently two different faces and should be highly resistant to oxidation or other side reactions in view of the fact that the ligand 13 is completely saturated.
COMPLEXATION OF CALIX[4]PYRROLIDINE
Having the ligand 13 in our hands complexation studies were undertaken using Cu2+ as the first metal. Spectrophotometric studies indicated immediately the formation of the copper complex 18 (Figure 19).
The complexation is a relatively slow process with a second order constant of 48 mol-1 min-1. The observed isosbestic point is in accordance with a process where only the starting materials and the product can be observed. No intermediate is detectable under those conditions. The chloride of the copper complex 18 crystallized and the X-ray structure of this complex was the first of a series of metal complexes studied in our group (Figure 20).
The structure of complex 18 proved to be prototypical for all the metal complexes, whose structures have been determined so far. The metal sits in the center of the macrocycle bound to the lone pairs of the four pyrrolidine nitrogen atoms. The coordination sphere of the metal is a distorted octahedron. One of the chlorides is in binding distance to the copper (2.4320(9) Å). The second chloride is kept in place by four hydrogen bonds to the N-H bonds of the pyrrolidine rings. The four N-H bonds form a sort of picket fence. The two faces of the macrocycle 18 are clearly differentiated in all the complexes we have studied so far.129,130
After the characterization of the Cu(II) complex 18 the analogous Mn(II) complex 19 could also be characterized (Figure 21). The comparison of the edge-on view of the free ligand 13 and the Mn(II) complex 19 show that only minimal geometric and conformational changes are induced by the metal complex formation. One of the chlorides is directly bound to the Mn(II) whereas the other chloride is held in place with four hydrogen bonds. The two faces of the metal complex 19 are clearly differentiated. Preliminary experiments showed that manganese complexes of our ligand can function as a catalyst for epoxidation reactions on olefins. A detailed study of the scope and limitation of the epoxidation reaction, the influence of additives and preliminary studies on the mechanism has been submitted for publication.12
SUMMARY
Starting from a simple concept, the hydrogenation of calix[4]pyrrole 1, we have embarked on a research project which has been full of obstacles and unexpected results. Almost all the steps were more complicated than anticipated. Most of the time the obstacles we encountered were not known, so that we were forced to discover new territory. The adventure has been beautiful and rewarding but strenuous. Detecting new and unprecedented processes and properties has always been a strong motivation for chemists to look at new structures and to explore them. The research project became considerably more complex than what was expected at the beginning. We have been lucky to achieve the partial and then the total hydrogenation of calix[4]pyrrole 1, a first achieved more than 100 years after the first reported synthesis of this macrocycle. The mechanistic analysis of the postulated mechanism has helped to advance and to establish a reproducible procedure which allows the isolation of three diastereoisomers 12a, 12b and 12c of the partially reduced macrocycle in good yields, using an easy to apply isolation procedure based on the differences of solubility and pH behavior. The yield of the totally reduced calix[4]pyrrolidine 13 has been more than doubled since we isolated this compound for the first time. The most rewarding and chemically the easiest part so far was the formation of the metal complexes. The structural studies of the ligand and of the metal complexes have shown, that the ligand 13 is quasi prepared to receive a metal atom. Minimum changes to the geometry and the conformation are necessary to form metal complexes. The ligand is an aliphatic “geometric surrogate” for the porphyrin ligands. The complete lack of double bonds avoids the sensitivity to oxidation of the ligand in catalytic oxidation processes. The ligand 13 is disk-like but not planar. The two faces of the ligand 13 and of its metal complexes e.g. 19 should allow us to obtain face selective reactivity. The shallow cleft formed by the four methyl groups gives a handle to stereoselective processes.
Despite the progress made we still work hard to improve the yield of the reduction to the totally saturated macrocycle 13. Despite our intensive efforts part of the hydrogenation mechanism is still an enigma. We intend to do mechanistic studies with the goal to discover the probable sequence of the hydrogenations of the four pyrrole rings. Another important directions of our research are studies towards the understanding of the reactivity of the partially reduced macrocycles of type 12. Preliminary results indicate unusual reactivities, which might be attributed to the tight hydrogen bond network which characterizes these macrocycles.
ACKNOWLEDGMENTS
This research project would not have been possible without the enthusiasm and the hard work of the collaborators in our group, who participated in this adventure. Important parts of their work have not been published yet. I would like to thank all of them for their contributions. In the sequence of their collaboration with our group I have to thank: Dr. Christoph Heiss, Michael Schmid, Vsevolod Khlebnikov, Dr. Christophe Letondor, Dr. Andreas Gualandi and Dr. Anca Pordea. The collaboration with Professor Diego Savoia (Bologna) has been crucial. Without the help of the X-ray structures done by Professor Helen Stoeckli-Evans (Neuchatel) and Dr. Lydia Karmazin-Brelot we would not have been able to make the progress we achieved. This project was launched as the consequence of a fruitful collaboration initiated by Privatdozent Dr. Josef Dannacher. Finally we have to thank CIBA Specialty Chemicals (today BASF Schweiz), the Swiss National Science Foundation and the University of Neuchatel for financing this research project.
EXPERIMENTAL
General
Hydrogenation of 5, 10, 15, 20, 22, 24-hexahydroporphine-5, 10, 15, 20-tetraspirocyclopentane (14): To a glass cylinder inside an autoclave vessel were added AcOH (100 mL), compound 14 (2.00 g, 3.75 mmol) and 10% Pd/C (0.66 g, 0.61 mmol of Pd). The autoclave was closed, put under magnetic stirring, filled with hydrogen (100 atm) and heated at 100 °C for 24 h. After cooling to room temperature, the reaction mixture was filtered through Celite and the solid was washed with AcOH (2 x 20 mL). The filtered solution was diluted with water (160 mL) and was neutralized with 2M NaOH aq. (50 mL). The solid was filtered off, washed with Et2O (30 mL) and then dried under vacuum to give a mixture of two diastereoisomers 16a and 16b as a white solid. The isomers were separated and purified by column chromatography (Al2O3, gradient: CH2Cl2/EtOAc, 10/1, 5/1, 1/1) to yield 0.555 g (18.5%) of 16a and 0.105 g (3.5%) of 16b as white solid.
Isomer 16a: 1H-NMR (400 MHz, CDCl3, 298 K): 10.30 (s br, 2H, NH-pyrrole), 5.76 (d, 4J(pyrrole-H, NH = 2.7, 4H, β-CH-pyrrole, H(2,3,12,13)), 3.09 (m, 4H, H(6,9,16,19)), 1.95-1.89 (m, 8H, CH-cyclopentane), 1.80 (s, 2H, NH-pyrrolidine), 1.78-1.66 (m, 4H, H(7,8,17,18) + 8H-CH2-cyclopentane), 1.65-1.53 (m, 16H, CH-cyclopentane), 1.42-1.38 (m, 4H, H(7,8,17,18)). 13C-NMR (100 MHz, CDCl3, 298K): 137.1 (C(1,4,11,14)), 103.3 (C(2,3,12,13)), 68.1 (C(6,9,16,19)), 49.4 (C(5,10,15,20)), 38.8 (CH2-cyclopentane), 35.0 (CaH2-cyclopentane), 26.9 (CH2–β-pyrrolidine, C (7,8,17,18)), 25.5 (CbH2-cyclopentane), 25.0 (CbH2-cyclopentane). IR (KBr): 3380m, 3337s, 3105w, 3092w, 2943s, 2868s, 2842w, 1625w, 1564m, 1450m, 1384m, 1232m, 1034m, 951w, 758s, 511w. MS (ESI(+)): 563.5 (11, [M+Na]+), 541.3 (100, [M + H]+). Anal. Calcd for C36H52N4: C 79.95, H 9.69, N 10.36. Found: C 80.01, H 9.90, N 10.41.
Isomer 16b: 1H-NMR (400 MHz, CDCl3, 298K): 11.08 (s, 2H, NH-pyrrole), 5.78 (d, 4J(pyrrole-H, NH = 2.6, 4H, H(2,3,12,13)), 3.13 (d, 3J = 5.0, 4H, N-CH-pyrrolidine, H(6,9,16,19)), 2.12 (m, 2H, NH-pyrrolidine), 1.97-1.91 (m, 8H, CH2-cyclopentane), 1.78-1.74 (m, 12H, CH2-pyrrolidine, H(7,8,17,18) + CH2-cyclopentane), 1.68-1.56 (m, 16H, CH2-pyrrolidine, H(7,8,17,18) + CH2-cyclopentane), 1.54-1.44 (m, 4H, CH2-cyclopentane). 13C-NMR (100 MHz, CDCl3, 298K) : 137.7 (C(1,4,11,14)), 102.2 (C(2,3,12,13)), 67.7 (C(6,9,16,19)), 49.0 (C(5,10,15,20)), 38.9, 35.0 (CaH2-cyclopentane), 26.6, 26.0 (CH2-β-pyrrolidine, C(7,8,17,18)), 25.0 (CH2-cyclopentane). IR (KBr): 3280s, 3096w, 2943-2839s, 1620w, 1563m, 1448m, 1392m, 1259m, 1090m, 1039m, 945w, 891m, 829m, 763s, 631w, 531w. MS (ESI(+)): 563.5 (11, [M+Na]+), 541.3 (100, [M + H]+). Anal. Calcd for C36H52N4: C 79.95, H 9.69, N 10.36. Found: C 79.82, H 9.91, N 10.32.
Hydrogenation of 1,3-alternate-5,10,15,20-tretraisobutyl-5,10,15-tretramethyl-5,10,15,20,22- hexahydroporphine (15): To a glass cylinder inside of an autoclave vessel were added AcOH (100 mL), compound 15 (3.00 g, 5.02 mmol) and 10% Pd/C (1 g, 0.93 mmol of Pd). The autoclave was closed, put under magnetic stirring, filled with hydrogen (100 atm) and heated at 100 °C for 24 h. After cooling to room temperature, the reaction mixture was filtered through Celite and the solid was washed with AcOH (2 x 20 mL) and CH2Cl2 (2 x 20 mL). The filtered solution was concentrated to give a slurry residue which was dried under high vacuum. The residue was dissolved in CH2Cl2 (50 mL) and washed with 10% Na2CO3aq (3 x 50 mL). The collected organic layers were washed with brine (25 mL), dried over K2CO3 and concentrated under reduced pressure to give colorless slurry. 15 mL of EtOH was added and the resulting precipitate was filtered off and then dried under vacuum to give a mixture of two diastereoisomers 17a and 17b as a white solid. The isomers were separated and purified by column chromatography (SiO2, gradient: hexane/EtOAc, 4/1, 3/1, 1/1) to yield 0.593 g (19.7%) of 17a and 0.327 g (10.9%) of 17b as white solid.
Isomer 17a: 1H-NMR (400 MHz, CDCl3, 298K): 9.47 (s br, 1H, NH-pyrrole), 5.75 (m, 2H, H (pyrrole)), 5.71 (m, 2H, H (pyrrole)), 3.01-2.94 (m, 4H, H(6,16) and (9,19)), 1.80 (dd, 2J = 13.7, 3J = 5.7, 2H, H (CH2-iBu)), 1.71-1.56 (m, 10H, H(7,8,17,18) + CH2-iBu), 1.44-1.38 (m, 4H, CH2-iBu), 1.33 (d, J = 4.6, 2H, H(CH-iBu)), 1.28, 1.20 (2xs, 12H, CH3-méso), 0.89 (d, 3J = 6.6, 12H, CH3-iBu), 0.87 (d, 3J = 6.5, 6H, CH3-iBu), 0.58 (d, 3J = 6.6, 6H, CH3-iBu). 13C-NMR (100 MHz, CDCl3, 298K): 136.5, 136.0 (C(1,4,11,14)), 104.5, 103.3 (C(2,3,12,13)), 70.7, 67.9 (C(6,9,16,19)), 49.9, 42.7 (CH2-iBu), 40.9, 40.7 (C(5,10,15,20)), 26.0, 25.5 (CH2, C(7,8,17,18)), 26.0, 25.9, 25.3, 24.8, 24.4, 24.3 (CH3-méso + CH3-iBu), 24.1, 22.8 (CH-iBu). IR (KBr): 3409w, 3309s, 3099w, 2932s, 2861, 2840s, 1645w, 1566m, 1490w, 1468m, 1410, 1398s, 1962, 1264m, 1092m, 929m, 773s, 727m, 599w, 537w. MS (ESI (+)): 605.4 (100, [M + H]+), 303.3 (21). Anal. Calcd for C40H60N4: C 79.41, H 11.33, N 9.26. Found C 79.50, H 11.63, N 9.21.
Isomer 17b: 1H-NMR (400 MHz, CDCl3, 298 K): 10.20, 9.50 (2xs br, 2 x 1H, NH-pyrrole), 5.78, 5.73 (2xm, 4H, H(2,3,12,13)), 3.04, 2.95 (2xm, 4 H, H(6,9,16,19)), 1.77-1.26 (m, 22H, H(7,8,17,18) + CH2-iBu + CH-iBu + NH-pyrrolidine), 1.28, 1.20 (2xs, 2x6H, CH3-méso), 0.91 (d, 3J = 6.7, 12H, CH3-iBu), 0.88 (d, 3J = 6.5, 6H, CH3-iBu), 0.59 (d, 3J = 6.6, 6H, CH3-iBu,). 13C-NMR (100 MHz, CDCl3, 298K) : 137.4, 135.2 (C(1,4,11,14)), 105.4, 103.5 (C(2,3, 12,13)), 69.7, 68.5 (C(6,9,16,19)), 50.5, 43.0 (CH2-iBu), 40.9, 40.1 (C(5,10,15,20)), 26.7, 26.3(CH2, C(7,8,17,18)), 26.1 (2xCH3-iBu), 24.8, 24.7 (CH-iBu), 24.6 (2xCH3-iBu), 23.5, 20.9 (CH3-méso). ESI-MS: 605.5 (100, [M+H]+), 370.3 (4). Anal. calcd for C40H60N4: C 79.41, H 11.33, N 9.26. Found C 79.65, H 11.66, N 9.19.
Synthesis of meso-octamethylcalix[4]pyrrolidine copper(II) complex (18): In a solution of calix[4]pyrrolidine 11 (100 mg, 0.22 mmol) in EtOH (6 mL) was added copper(II) chloride (39 mg, 0.2 mmol). The reaction was heated to reflux for 2 hours giving a blue solution. The solvent was removed under vacuum to give a blue solid (103 mg, 72%) which was purified by column chromatography (Al2O3, CH2Cl2/MeOH, 99/1) to yield 95.9 mg (67%) of 18. IR (KBr): 3435br, 3214w, 2975s, 1476-1453m, 1416s, 1373m, 1154w, 1018s, 1053s, 986s, 936w, 915s. MS (ESI(+)): 542.3 (100, [M(18) -Cl]+).
References
1. A. Baeyer and V. Drewsen, Ber. Dtsch. Chem. Ges., 1882, 15, 2856. CrossRef
2. O. Wallach, Liebigs Ann. Chem., 1889, 252, 106. CrossRef
3. E. Fischer, Ber. Dtsch. Chem. Ges., 1890, 23, 799. CrossRef
4. R. G. Ault, D. K. Baird, H. C. Carrington, W. N. Haworth, R. Herbert, E. L. Hirst, E. G. V. Percival, F. Smith, and M. Stacey, J. Chem. Soc., 1933, 1419. CrossRef
5. R. G. Ault, W. N. Haworth, and E. L. Hirst, J. Chem. Soc., 1934, 1722. CrossRef
6. D. K. Baird, W. N. Haworth, R. W. Herbert, E. L. Hirst, F. Smith, and M. Stacey, J. Chem. Soc., 1934, 62. CrossRef
7. W. N. Haworth, E. L. Hirst, J. K. N. Jones, and F. Smith, J. Chem. Soc., 1934, 1192. CrossRef
8. P. Karrer and K. Schopp, Helv. Chim. Acta, 1932, 15, 745. CrossRef
9. P. Karrer, B. von Euler, and H. von Euler, Ark. Kemi, Mineral. Geol., 1928, 10B, 6.
10. H. Wieland and F. Weil, Z. physiol. Chem., 1913, 80, 287.
11. A. Butenandt, Trends Biochem. Sci., 1979, 4, 215. CrossRef
12. A. Eschenmoser, Helv. Chim. Acta, 2010, 93, 1439. CrossRef
13. K.-H. Altmann, J. Buchner, H. Kessler, F. Diederich, B. Krautler, S. Lippard, R. Liskamp, K. Muller, E. M. Nolan, B. Samori, G. Schneider, S. L. Schreiber, H. Schwalbe, C. Toniolo, C. A. A. van Boeckel, H. Waldmann, and C. T. Walsh, ChemBioChem, 2009, 10, 16. CrossRef
14. A. Eschenmoser, Angew. Chem., Int. Ed. Engl., 1988, 27, 5. CrossRef
15. A. Eschenmoser, Tetrahedron, 2007, 63, 12821. CrossRef
16. B. Kraeutler and S. Ostermann, 'The Porphyrin Handbook'. Vol. 11 ed. by K. M. Kadish, K. M. Smith, and R. Guilard, Academic Press. Inc., San Diego, 2003; pp. 229-276.
17. B. Kraeutler, Chimia, 1987, 41, 277.
18. B. Kraeutler, Biochem. Soc. Trans., 2005, 33, 806. CrossRef
19. H. Scheer, 'Advances in Photosynthesis and Respiration,' Vol. 25, ed. by B. Grimm, R. J. Porra, W. Ruediger, and H. Scheer, Springer, Dordrecht, 2006, pp. 1-26.
20. H. Scheer, Compr. Ser. Photochem. Photobiol. Sci., 2008, 8, 101.
21. H. Scheer, Wiley Encycl. Chem. Biol., 2009, 1, 341.
22. W.-D. Woggon, Chimia, 1999, 53, 234.
23. W.-D. Woggon, Acc. Chem. Res., 2005, 38, 127. CrossRef
24. W.-D. Woggon, Met. Ions Life Sci., 2007, 3, 27.
25. A. R. Battersby and E. McDonald, Acc. Chem. Res., 1979, 12, 14. CrossRef
26. A. R. Battersby, C. J. R. Fookes, G. W. J. Matcham, and E. McDonald, Nature (London), 1980, 285, 17. CrossRef
27. A. R. Battersby, Nat. Prod. Rep., 2000, 17, 507. CrossRef
28. A. R. Battersby, Acc. Chem. Res., 1986, 19, 147. CrossRef
29. P. M. Jordan, Ciba Found. Symp., 1994, 180, 70.
30. A. I. Scott and C. A. Roessner, Biochem Soc Trans, 2002, 30, 613. CrossRef
31. E. K. Jaffe, Open Conf. Proc. J., 2010, 1, 1.
32. E. K. Jaffe, Bioorg. Chem., 2004, 32, 316. CrossRef
33. A. R. Battersby and F. J. Leeper, Top. Curr. Chem., 1998, 195, 143. CrossRef
34. H.-J. Kwon, C. Smith Wyatt, A. J. Scharon, H. Hwang Sung, J. Kurth Mark, and B. Shen, Science, 2002, 297, 1327. CrossRef
35. A. I. Scott, J. Org. Chem., 2003, 68, 2529. CrossRef
36. S. S. Lamb and G. D. Wright, Proc. Natl. Acad. Sci. U. S. A., 2005, 102, 519. CrossRef
37. F. Stauffer, E. Zizzari, C. Soldermann-Pissot, J.-P. Faurite, and R. Neier, Chimia, 2001, 55, 314.
38. P. Bobal and R. Neier, 'Trends in Organic Chemistry,' Vol. 6, Research Trends Ltd., 1997, pp. 125-144.
39. C. P. Soldermann, R. Vallinayagam, M. Tzouros, and R. Neier, J. Org. Chem., 2008, 73, 764. CrossRef
40. R. Neier, 'Advances in Nitrogen Heterocycles,' Vol. 2, ed. by C. J. Moody, JAI Press Inc., Greenwich CT, 1996, pp. 35-146.
41. D. Mauzerall, J. Am. Chem. Soc., 1960, 82, 2605. CrossRef
42. D. Mauzerall, J. Am. Chem. Soc., 1960, 82, 2601. CrossRef
43. G. R. Geier, III and J. S. Lindsey, J. Chem. Soc., Perkin Trans. 2, 2001, 687. CrossRef
44. F. Li, K. Yang, J. S. Tyhonas, K. A. MacCrum, and J. S. Lindsey, Tetrahedron, 1997, 53, 12339. CrossRef
45. J. S. Lindsey, V. Chandrashaker, M. Taniguchi, and M. Ptaszek, New J. Chem., 35, 65. CrossRef
46. J. S. Lindsey, M. Ptaszek, and M. Taniguchi, Origins Life Evol. Biospheres, 2009, 39, 495. CrossRef
47. P. M. Shoolingin-Jordan, 'Vitamin B12 and B12-Proteins, (Proceedings of the 4th European Symposium held in Innsbruck, Austria, in September 1996.),' ed. by B. Kraeutler, D. Arigoni, and B. Golding, Wiley-VCH, Weinheim, 1998, p. 101.
48. P. M. Shoolingin-Jordan and K.-M. Cheung, 'Comprehensive Natural Products Chemistry,' Vol. 4, ed. by J. W. Kelly, Elsevier Science B.V., Amsterdam, 1999, pp. 61-107. CrossRef
49. D. Jacoby, C. Floriani, A. Chiesi-Villa, and C. Rizzoli, J. Chem. Soc., Chem. Commun., 1991, 790. CrossRef
50. D. Jacoby, S. Isoz, C. Floriani, A. Chiesi-Villa, and C. Rizzoli, J. Am. Chem. Soc., 1995, 117, 2805. CrossRef
51. D. Jacoby, S. Isoz, C. Floriani, A. Chiesi-Villa, and C. Rizzoli, J. Am. Chem. Soc., 1995, 117, 2793. CrossRef
52. J. Jubb, D. Jacoby, C. Floriani, A. Chiesi-Villa, and C. Rizzoli, Inorg. Chem., 1992, 31, 1306. CrossRef
53. L. Bonomo, E. Solari, R. Scopelliti, and C. Floriani, Chemistry, 2001, 7, 1322. CrossRef
54. C. Floriani and R. Floriani-Moro, 'The Porphyrin Handbook,' Vol. 3, ed. by K. M. Kadish, K. M. Smith, and S. R. Guilard, Academic Press. Inc., San Diego, 2000, pp. 385-403.
55. C. Floriani, Chem. Commun., 1996, 1257. CrossRef
56. C. Angst, M. Kajiwara, E. Zass, and A. Eschenmoser, Angew. Chem., Int. Ed. Engl., 1980, 19, 140. CrossRef
57. A. Eschenmoser, Nova Acta Leopold., 1982, 55, 47.
58. A. Eschenmoser, Ann. N. Y. Acad. Sci., 1986, 471, 108. CrossRef
59. R. Schwesinger, R. Waditschatka, J. Rigby, R. Nordmann, W. B. Schweizer, E. Zass, and A. Eschenmoser, Helv. Chim. Acta, 1982, 65, 600. CrossRef
60. R. Waditschatka, C. Kratky, B. Jaun, J. Heinzer, and A. Eschenmoser, J. Chem. Soc., Chem. Commun., 1985, 1604. CrossRef
61. N. Fotinos, M. A. Campo, F. Popowycz, R. Gurny, and N. Lange, Photochem. Photobiol., 2006, 82, 994. CrossRef
62. A. E. O'Connor, W. M. Gallagher, and A. T. Byrne, Photochem. Photobiol., 2009, 85, 1053.
63. M. Studer, A. Riesen, and T. A. Kaden, Helv. Chim. Acta, 1989, 72, 1253. CrossRef
64. E. Kimura, Y. Kodama, M. Shionoya, and T. Koike, Inorg. Chim. Acta, 1996, 246, 151. CrossRef
65. P. S. Bryan and J. C. Dabrowiak, Inorg. Chem., 1975, 14, 296. CrossRef
66. Z. Guo and P. J. Sadler, Angew. Chem. Int. Ed., 1999, 38, 1512. CrossRef
67. R. Hage, J. E. Iburg, J. Kerschner, J. H. Koek, E. L. M. Lempers, R. J. Martens, U. S. Racherla, S. W. Russell, T. Swarthoff, M. R. P. van Vliet, J. B. Warnaar, L. van der Wolf, and B. Krijnen, Nature, 1994, 369, 637. CrossRef
68. D. De Vos and T. Bein, Chem. Commun., 1996, 917. CrossRef
69. J. T. Groves and T. E. Nemo, J. Am. Chem. Soc., 1983, 105, 5786. CrossRef
70. B. Meunier, E. Guilmet, M. E. De Carvalho, and R. Poilblanc, J. Am. Chem. Soc., 1984, 106, 6668. CrossRef
71. W. Nam, R. Ho, and J. S. Valentine, J. Am. Chem. Soc., 1991, 113, 7052. CrossRef
72. M. R. Bukowski, K. D. Koehntop, A. Stubna, E. L. Bominaar, J. A. Halfen, E. Muenck, W. Nam, and L. Que, Jr., Science, 2005, 310, 1000. CrossRef
73. F. Tiago de Oliveira, A. Chanda, D. Banerjee, X. Shan, S. Mondal, L. Que, Jr., E. L. Bominaar, E. Muenck, and T. J. Collins, Science, 2007, 315, 835. CrossRef
74. Y. Kang, H. Chen, Y. J. Jeong, W. Lai, E. H. Bae, S. Shaik, and W. Nam, Chem. Eur. J., 2009, 15, 10039. CrossRef
75. Y. Gao, T. Akermark, J. Liu, L. Sun, and B. Akermark, J. Am. Chem. Soc., 2009, 131, 8726. CrossRef
76. A. J. McGown, W. D. Kerber, H. Fujii, and D. P. Goldberg, J. Am. Chem. Soc., 2009, 131, 8040. CrossRef
77. M. C. White, A. G. Doyle, and E. N. Jacobsen, J. Am. Chem. Soc., 2001, 123, 7194. CrossRef
78. A. L. Gavrilova and B. Bosnich, Chem. Rev., 2004, 104, 349. CrossRef
79. K. Nehru, S. J. Kim, I. Y. Kim, M. S. Seo, Y. Kim, S.-J. Kim, J. Kim, and W. Nam, Chem. Commun., 2007, 4623. CrossRef
80. J. England, M. Martinho, E. R. Farquhar, J. R. Frisch, E. L. Bominaar, E. Munck, and L. Que, Jr., Angew. Chem. Int. Ed., 2009, 48, 3622. CrossRef
81. M. S. Chen and M. C. White, Science, 2007, 318, 783. CrossRef
82. J. M. Ready and E. N. Jacobsen, Angew. Chem. Int. Ed., 2002, 41, 1374. CrossRef
83. J. M. Lehn, Pure Appl. Chem., 1979, 51, 979. CrossRef
84. J. M. Lehn, 'Supramolecular Chemistry: Concepts and Perspectives' VCH, Weinheim, 1995.
85. C. J. Pederson, J. M. Lehn, and D. J. Cram, Resonance, 2001, 6, 71.
86. R. J. Geue, T. W. Hambley, J. M. Harrowfield, A. M. Sargeson, and M. R. Snow, J. Am. Chem. Soc., 1984, 106, 5478. CrossRef
87. J. M. Harrowfield, A. J. Herlt, P. A. Lay, A. M. Sargeson, A. M. Bond, W. A. Mulac, and J. C. Sullivan, J. Am. Chem. Soc., 1983, 105, 5503. CrossRef
88. P. M. Angus, A. M. T. Bygott, R. J. Geue, B. Korybut-Daszkiewicz, A. W. H. Mau, A. M. Sargeson, M. M. Sheil, and A. C. Willis, Chem. Eur. J., 1997, 3, 1283. CrossRef
89. T. J. Hubin, Coord. Chem. Rev., 2003, 241, 27. CrossRef
90. J. C. Timmons and T. J. Hubin, Coord. Chem. Rev., 254, 1661.
91. A. Baeyer, Ber. Dtsch. Chem. Ges., 1886, 2184.
92. V. V. Chelintzev, B. V. Tronov, and S. G. Karmanov, J. Russ. Chem. Soc., 1916, 48, 1210.
93. P. Rothemund and C. L. Gage, J. Am. Chem. Soc., 1955, 77, 3340. CrossRef
94. P. A. Gale, J. L. Sessler, V. Kral, and V. Lynch, J. Am. Chem. Soc., 1996, 118, 5140. CrossRef
95. P. A. Gale and J. L. Sessler, 'The Porphyrin Handbook,' Vol. 6, ed. by K. M. Kadish, K. M. Smith, and S. R. Guilard, Academic Press. Inc., San Diego, 2000, pp. 257-278.
96. J. Jubb, D. Jacoby, C. Floriani, A. Chiesi-Villa, and C. Rizzoli, Inorg. Chem., 1992, 31, 1306. CrossRef
97. W. E. Allen, P. A. Gale, C. T. Brown, V. M. Lynch, and J. L. Sessler, J. Am. Chem. Soc., 1996, 118, 12471. CrossRef
98. J. L. Sessler, A. Andrievsky, P. A. Gale, and V. Lynch, Angew. Chem., Int. Ed. Engl., 1997, 35, 2782. CrossRef
99. D. Jacoby, C. Floriani, A. Chiesi-Villa, and C. Rizzoli, J. Chem. Soc., Chem. Commun., 1991, 220. CrossRef
100. M. Van Beylen, B. Roland, G. S. D. King, and J. Aerts, J. Chem. Res., M., 1985, 4201.
101. B. R. Kusche, A. E. Smith, M. A. McGuirl, and N. D. Priestley, J. Am. Chem. Soc., 2009, 131, 17155. CrossRef
102. E. Vogel, P. Roehrig, M. Sicken, B. Knipp, A. Herrmann, M. Pohl, H. Schmickler, and J. Lex, Angew. Chem., Int. Ed. Engl., 1989, 28, 1651. CrossRef
103. M. Ahmed and O. Meth-Cohn, Tetrahedron Lett., 1969, 1493. CrossRef
104. G.-A. Lee, W.-C. Wang, M. Shieh, and T.-S. Kuo, Chem. Commun. (Cambridge, U. K.), 2010, 46, 5009. CrossRef
105. A. Nagarajan, J. W. Ka, and C. H. Lee, Tetrahedron, 2001, 57, 7323. CrossRef
106. G.-A. Lee, W.-C. Wang, M. Shieh, and T.-S. Kuo, Chem. Commun. (Cambridge, U. K.), 2010, 46, 500.
107. K. E. Wiegers and S. G. Smith, J. Org. Chem., 1978, 43, 1126. CrossRef
108. R. G. Ackman, W. H. Brown, and G. F. Wright, J. Org. Chem., 1955, 20, 1147. CrossRef
109. R. E. Beals and W. H. Brown, J. Org. Chem., 1956, 21, 447. CrossRef
110. W. H. Brown, B. J. Hutchinson, and M. H. MacKinnon, Can. J. Chem., 1971, 49, 4017. CrossRef
111. W. J. Hale, W. D. McNally, and C. J. Pater, Amer. Chem. J., 1906, 35, 68. CrossRef
112. M. Chastrettea and F. Chastrette, J. Chem. Soc., Chem. Commun., 1973, 534. CrossRef
113. M. Chastrette, F. Chastrette, and J. Sabadie, Org. Synth., 1977, 57, 74.
114. M. de Sousa Healy and A. J. Rest, J. Chem. Soc., Perkin Trans. 1, 1985, 973. CrossRef
115. Y. Kobuke, K. Hanji, K. Horiguchi, M. Asada, Y. Nakayama, and J. Furukawa, J. Am. Chem. Soc., 1976, 98, 7414. CrossRef
116. A. J. Rest, S. A. Smith, and I. D. Tyler, Inorg. Chim. Acta, 1976, 16, L1. CrossRef
117. M. Van Beylen, B. Roland, G. S. D. King, and J. Aerts, J. Chem. Res. (S), 1985, 388.
118. B. Roland, M. Van Beylen, and J. Smid, J. Phys. Chem., 1994, 98, 4707. CrossRef
119. L. A. R. Pioda, A. H. Wachter, R. E. Dohner, and W. Simon, Helv. Chim. Acta, 1967, 50, 1373. CrossRef
120. J. L. Pierre and H. Handel, Tetrahedron Lett., 1974, 2317. CrossRef
121. H. Handel and J. L. Pierre, Tetrahedron, 1975, 31, 997. CrossRef
122. H. Handel and J. L. Pierre, Tetrahedron Lett., 1976, 2029. CrossRef
123. C. W. Bird and G. W. H. Cheeseman, 'Comprehensive Heterocyclic Chemistry,' Vol. 4, ed. by C. W. Bird and G. W. H. Cheeseman, Pergamon Press, Oxford, p. 29.
124. K. Mills and J. A. Joule, 'Heterocyclic Chemistry,' 5th Ed., Blackwell Publishing, Oxford, 2010.
125. C. Heiss, Diploma work 2000, M. Schmid, Progress report, summarized in V. Blangy, Ph.D., University of Neuchatel, 2007.
126. G. Lunn, J. Org. Chem., 1987, 52, 1043. CrossRef
127. C. G. Overberger, L. C. Palmer, B. S. Marks, and N. R. Byrd, J. Am. Chem. Soc., 1955, 77, 4100. CrossRef
128. Paper submitted.
129. V. Blangy, C. Heiss, V. Khlebnikov, C. Letondor, H. Stoeckli-Evans, and R. Neier, Angew. Chem. Int. Ed., 2009, 48, 1688. CrossRef
130. G. Journot, C. Letondor, R. Neier, H. Stoeckli-Evans, D. Savoia, and A. Gualandi, Chem. Eur. J., 2010, 16, 4224. CrossRef