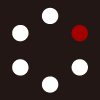
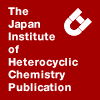
HETEROCYCLES
An International Journal for Reviews and Communications in Heterocyclic ChemistryWeb Edition ISSN: 1881-0942
Published online by The Japan Institute of Heterocyclic Chemistry
e-Journal
Full Text HTML
Received, 11th June, 2011, Accepted, 1st July, 2011, Published online, 12th July, 2011.
DOI: 10.3987/COM-11-S(P)35
■ Unusual Base-Induced Rearrangement of exo-9-Oxabicyclo[4.2.1]non-7-ene Oxide to exo-8-Hydroxybicyclo[3.3.0]octan-2-one
David M. Hodgson,* Matthew A. H. Stent, Robert S. Paton, and Francis X. Wilson
Chemistry Research Laboratory, Department of Chemistry, University of Oxford, Mansfield Road, Oxford OX1 3TA, U.K.
Abstract
Reaction of exo-9-oxabicyclo[4.2.1]non-7-ene oxide with n-BuLi gives exo-8-hydroxybicyclo[3.3.0]octan-2-one. Potential reaction pathways for this unusual rearrangement were evaluated with the aid of computational studies. The process is proposed to proceed by elimination to a transient allene oxide, which rearranges to a trans-epoxide enolate before undergoing epoxide α-lithiation and transannular C−H insertion.INTRODUCTION
Epoxides are versatile synthetic intermediates.4 Their reactions are dominated by the electrophilic nature of the epoxide, generally involve cleavage of the strained three-membered ring, and include a wide range of nucleophilic ring-openings and acid- and base-induced isomerisation reactions. Building on studies by Cope,5 Crandall6 and Mioskowski7 on the carbenoid nature of α-lithiated epoxides,8 we have reported on the (enantioselective) organolithium-induced transannular desymmetrisation of cyclooctene oxides (eg, Scheme 1),9 and alkylative double ring-opening of epoxides of dihydrofurans, dihydropyrans, and of oxabicyclo[n.2.1]alkenes (n = 1, 2) (Scheme 2).10 Examination of this chemistry for the latter substrate class when n = 3 has led to an unusual rearrangement, which constitutes the topic of the present paper.
RESULTS AND DISCUSSION
The synthesis of exo-9-oxabicyclo[4.2.1]non-7-ene oxide (3) (Scheme 3) commenced with the preparation of the precursor 9-oxabicyclo[4.2.1]non-7-ene (2) by oxymercuration – demercuration of 1,3-cyclooctadiene (1) (28% yield, lit.11 37% yield). Epoxidation of 2 with in situ generated methyl(trifluoromethyl)dioxirane12 gave a single isomer (by crude 1H NMR analysis), the epoxide 3 being obtained in 72% yield. By analogy with epoxidations of oxabicyclo[n.2.1]alkenes (n = 1, 2) and homotropenes (2, where O is replaced by N-R),13 the epoxidation of 2 is expected to occur on the less-hindered exo-face. As exo-7,8-epoxy-N-(benzyloxycarbonyl)-9-azabicyclo[4.2.1]nonane (3, where bridge O is replaced by N-Z) exhibits no significant 3J coupling between epoxide and bridgehead H’s (dihedral angle close to 0º), whereas 3.6 Hz is observed for the corresponding endo-epoxide (dihedral angle close to 90º),13a then the absence of any such coupling for 3 provides further support for the exo-configurational assignment.
Application of the standard alkylative double ring-opening reaction conditions used in Scheme 2 [n-BuLi (2.5 equiv.), THF, –78 ºC to 25 ºC, 16 h] to epoxide 3 gave exo-8-hydroxybicyclo[3.3.0]octan-2-one (4)14 (58% yield, Scheme 3), rather than the corresponding cyclooctenediol which would have been expected if chemistry analogous to that shown in Scheme 2 was followed. Exo-4 could also be obtained enantioenriched (85:15 er, absolute configuration of dominant enantiomer not known), albeit in only 16% yield, from epoxide 3 by using i-PrLi (2.5 equiv.) in the presence of the external chiral ligand (−)-sparteine (2.5 equiv.) in ether (–78 ºC to 25 ºC, 16 h).9,10,15 The structural and stereochemical assignment of 4 was unambiguously established through both spectral comparisons and chemical manipulation. Comparison of the 1H and 13C NMR data with that known for both exo- and endo-isomers was indicative for exo-4.14c,16 Reduction of 4 (NaBH4, MeOH, 0 ºC, 1 h) gave the non-meso diol 517 (59% yield), consistent with 4 being the exo-isomer (Scheme 4). To exclude the possibility that hydroxyl-directed reduction of the endo-isomer had resulted in the non-meso diol 5 we further derivatised 4 as the TBS ether 6 (having different spectral characteristics to the known18 endo-TBS ether); reduction to alcohol 7 and desilylation again gave the non-meso diol 5.
The unusual rearrangement of epoxide 3 to exo-alcohol 4 merits some discussion. Initial epoxide α-lithiation might reasonably be considered to be the first step, in common with the chemistry shown in Schemes 1 and 2. The difference in reactivity seen between the current ether-bridged cyclooctene oxide 3 and the cycloheptene- and cyclohexene-oxide ether-bridged systems studied earlier (Scheme 2) could then relate to the propensity for such medium-ring systems to engage in transannular chemistry (eg, Scheme 1).5,9,19 That is, a combination of steric impediment to the 1,2-metallate shift20 due to the rear methylene groups, together with the closeness of one of the methylene CHs to the carbenoid centre in 3-Li could retard the 1,2-metallate process and accelerate a transannular C−H insertion. This mechanistic scenario would lead to tricyclic alkoxide 8 (Scheme 5). Such tricyclic systems are known,21 but attempted low temperature trapping of the putative tricyclic intermediate 8 [n-BuLi (1.1 equiv.), −78 ºC, 3 h] resulted only in recovery of starting epoxide 3. In the present case, excess base could drive an elimination (shown in 8') with relief of ring strain to give endo-alkoxide enolate 9. A difficulty then arises in satisfactorily progressing 9 to the observed exo-alcohol 4, which would require a retro-aldol − aldol process in the work-up (pH 7 buffer). Even if such an interconversion occurred, endo-alcohol 4 is known to predominate at equilibrium (62:11:27, endo-4 : exo-4 : bridged aldol epimers),14c so any reaction pathway proceeding though endo-alcohol 4 can be ruled out.
An alternative, more speculative suggestion for the generation of exo-alcohol 4 is by way of the concerted 1,2-H shift/retro-aldolate process22 shown in tricyclic intermediate 8" to directly generate an enolate aldehyde 10 (Scheme 5), which might undergo a kinetic aldol ring closure with opposite selectivity to that observed under acidic conditions.23 DFT optimisations performed at the wB97XD/6-31G(d) level of theory in a CPCM solvation model of THF24 were carried out to gauge the feasibility of these steps. The optimised structure of the intermediate tricyclic alkoxide 8 shows the alkoxide C−H to be antiperiplanar to the ethereal C−O bond, and is elongated by 0.02 Å relative to typical C−H distances indicative of σCH – σ*CO hyperconjugation (Scheme 6). In model calculations where the Li cation was replaced with a proton, it was possible to locate a TS for a concerted 1,2-H shift/retro-aldol, forming the enol aldehyde 11, although the barrier is prohibitively high (75 kcal/mol). Attempts to locate a similar structure with Li (cf, 8") were unsuccessful since, on 1,2-hydride shift resulting in ether C−O cleavage, the Li cation moves to stabilise the developing alkoxide and so avoids a concomitant retro-aldolate process which would form the enolate aldehyde 10; an unfeasibly high activation barrier of 46 kcal/mol was also calculated for this process.25 The transition state for the 1,2-hydride shift (8"') leads to the formation of the endo β-lithiooxyketone endo-4-Li (Scheme 6), downhill in free energy by 16 kcal/mol. The retro-aldolate TS endo-4-Li' lies uphill by only 8.3 kcal/mol, and leads to a potential energy surface which is extremely flat, such that further elongation of the C−C bond causes a very slight decrease in energy. The energy minimum found for the enolate aldehyde 10 has a C---C separation of 2.5 Å and so resembles the TS geometry closely.26 Even if enolate aldehyde 10 could be formed by some means, no TS exists (at this level of theory/solvation model)24 for the formation of exo-4-Li (which is 3.5 kcal/mol higher in energy than endo-4-Li). Instead the potential energy falls continuously from enolate to product.
With no experimental or computational support for the formation of exo-4 from epoxide 3 by either of the two pathways proceeding by way of initial epoxide α-lithiation − transannular C−H insertion and a retro-aldol(ate) process discussed above (Schemes 5 and 6), we considered a pathway passing through a lithiated trans-cyclooctene oxide. Cope and co-workers had originally shown that lithiated trans- and cis-cyclooctene oxides undergo stereospecific transanular insertion chemisty, with the cis-isomer proceeding as in Scheme 1 and, importantly in the current context, the trans-isomer leading to the exo-alcohol (Scheme 7).5,27
A feasible pathway for the formation of exo-alcohol 4 from epoxide 3, which proceeds through a trans-epoxide, is outlined in Scheme 8. 3-Li is aligned for anti-elimination (Li−C−C−O dihedral angle is 169°) to give a transient allene oxide 12.28,29 Such a process is not seen for epoxides of oxabicyclo[n.2.1]alkenes (n = 1, 2) (Scheme 2) due to the more favorable 1,2-metallate shift process and higher energy TSs to more strained allene oxides. In the present case, the ether bridge may also retard the transannular pathway (cf, Scheme 1) relative to the elimination. The allene oxide 12 can rearrange to the trans-epoxide enolate 13. Regioselective lithiation of trans-epoxide enolate 13 to give 13-Li followed by transannular C−H insertion finds precedent in the work of Cope discussed above (Scheme 7) and in the work of Crandall and Chang concerning α-lithiation − transannular C−H insertion of the mono epoxide of 1.30 While transition states could not be located, thermodynamics are favourable for the formation of each of the intermediates proposed.
In summary, an unusual base-induced reaction of exo-9-oxabicyclo[4.2.1]nonene oxide (3) to exo-8-hydroxybicyclo[3.3.0]octan-2-one (4) has been discovered. A rationalisation, which has its origins in classic transannular chemistry studies by Cope over half a century ago, provides a satisfactory explanation as to why only the exo-isomer is observed.
EXPERIMENTAL
All reactions requiring anhydrous conditions were conducted in flame- or oven-dried apparatus under an atmosphere of argon. Syringes and needles for the transfer of reagents were dried at 140 ºC and allowed to cool in a desiccator over P2O5 before use. Ethers were distilled from sodium benzophenone ketyl under argon; CH2Cl2 from CaH2 under argon. External reaction temperatures are reported unless stated otherwise. Reactions were monitored by TLC, using commercially available aluminium-backed plates, precoated with a 0.25 mm layer of silica containing a fluorescent indicator (Merck). Organic layers were dried over MgSO4. Column chromatography was carried out on Kieselgel 60 (40–63 μm). Light petroleum refers to the fraction with bp 40–60 ºC. Melting points were determined using a Gallenkamp hot stage apparatus and are uncorrected. Elemental analysis was performed by Elemental Microanalysis Limited, Okehampton, Devon, UK. IR spectra were recorded as thin films unless stated otherwise. Peak intensities are specified as strong (s), medium (m) or weak (w). 1H and 13C NMR spectra were recorded in CDCl3 with a Brüker JEOL EX400 spectrometer. Chemical shifts are reported relative to CHCl3 [δ H 7.26, δ C 77.0]. Coupling constants (J) are given in Hz. Chiral GC was performed at 100 ºC on a ThermoQuest CE Instruments TRACE GC, fitted with a CYDEX-β column.
9-Oxabicyclo[4.2.1]non-7-ene (2)
1,3-Cyclooctadiene (1) (6.2 mL, 50 mmol) and Hg(OAc)2 (47.8. g, 150 mmol) in a mixture of THF (85 mL) and H2O (85 mL) was stirred at 25 °C for 5 days. Aqueous NaOH (3 M, 65 mL) was added and stirring continued for a few min until the mixture became black. A solution of NaBH4 (3.80 g, 100 mmol) in aqueous NaOH (3 M, 65 mL) was added over 30 min and mercury was observed to form. The mixture was saturated with NaCl and extracted with Et2O (2 x 100 mL). The combined organic layers were washed with H2O (2 x 100 mL), dried, filtered and concentrated under reduced pressure. Bulb-to-bulb distillation (50 °C / 5 mbar; lit.11a 74 °C / 10 mmHg) gave alkene 2 as a colourless oil (1.72 g, 28%); Rf 0.35 (50% Et2O in petrol); νmax/cm–1 2920s br, 2855s, 1444m, 1090s and 1018s; 1H NMR δ 5.80 (2H, s), 4.98 (2H, d, J 6.4), 1.92–1.85 (2H, m), 1.62–1.53 (4H, m) and 1.49–1.43 (2H, m); 13C NMR δ 130.8, 80.0, 33.8 and 25.2.
exo-7,8-Epoxy-9-oxabicyclo[4.2.1]nonane (3)
To a solution of alkene 2 (1.46 g, 11.8 mmol) and aqueous Na2EDTA (400 μM, 60 mL, 24 μmol) in MeCN (90 mL) at 0 °C was added trifluoroacetone (11.6 mL, 130 mmol) from a pre-cooled syringe. The resulting homogeneous mixture was treated with a mixture of Oxone® (18.1 g, 58.8 mmol) and NaHCO3 (7.90 g, 94.1 mmol) portionwise over 30 min, then stirred at 0 °C for 4.5 h. H2O (450 mL) was added and the reaction mixture extracted with CH2Cl2 (3 x 450 mL). The combined organic layers were dried, filtered and concentrated under reduced pressure. Purification of the residue by column chromatography (SiO2, 50% Et2O in petrol) gave epoxide 3 as a white solid (1.18 g, 72%); Rf 0.25 (50% Et2O in petrol); mp. 58 – 60 °C; (Found: C, 68.3; H, 8.7. C8H12O2 requires C, 68.5; H, 8.6%); νmax/cm–1 (KBr) 2928m, 2859m, 1456m, 1090s and 957s; 1H NMR δ 4.32 (2H, d, J 6.9), 3.47 (2H, s), 1.98–1.83 (2H, m), 1.65–1.51 (4H, m) and 1.47–1.37 (2H, m); 13C NMR δ 75.7, 58.4, 31.3 and 24.3; m/z [APCI +] 141 (M + H+, 100%), 121 (30) and 105 (45).
exo-8-Hydroxybicyclo[3.3.0]octan-2-one (4)
To a solution of epoxide 3 (80 mg, 571 μmol) in THF (5 mL) at –78 °C was added n-BuLi (2.2 M in hexanes, 0.65 mL, 1.4 mmol) dropwise. The mixture was stirred at –78 °C for 5 h and then allowed to warm to 25 °C over 16 h. Phosphate buffer (pH 7, 5 mL) was added and the mixture extracted with EtOAc (2 x 10 mL). The combined organic layers were dried, filtered and concentrated under reduced pressure. Purification of the residue by column chromatography (SiO2, 75% Et2O in petrol) gave hydroxy ketone 414 as a colourless oil (46 mg, 58%); Rf 0.15 (75% Et2O in petrol); νmax/cm–1 3414s br, 2951s, 2872m, 1732s, 1461m and 1224m; 1H NMR δ 4.15 (1H, br s), 2.85–2.78 (1H, m), 2.39 (1H, d, J 9.0), 2.11–2.05 (2H, m), 2.00–1.91 (2H, m), 1.53–1.43 (3H, m) and 1.27–1.02 (1H, m); 13C NMR δ 220.8, 76.6, 61.7, 39.2, 37.9, 35.2, 30.6 and 26.4; m/z [CI + (NH3)] 158 (M + NH4+, 100%) (Found: M + NH4+, 158.1183. C8H16NO2 requires 158.1181).
(+)-exo-8-Hydroxybicyclo[3.3.0]octan-2-one (4)
To a solution of (−)-sparteine (0.33 mL, 1.44 mmol) in Et2O (5 mL) at –78 °C was added i-PrLi31 (1.5 M in petrol, 0.95 mL, 1.43 mmol) dropwise. After stirring at –78 °C for 1 h a solution of epoxide 3 (80 mg, 571 μmol) in Et2O (3 mL) was added dropwise. The mixture was stirred at –78 °C for 5 h and then allowed to warm to 25 °C over 16 h. Aqueous HCl (0.5 M, 5 mL) was added and the mixture extracted with EtOAc (2 x 10 mL). The combined organic layers were dried, filtered and concentrated under reduced pressure. Purification of the residue by column chromatography (SiO2, 75% Et2O in petrol) gave hydroxyketone 4 as a colourless oil (13 mg, 16%); [*] +56 (c 1.0, CHCl3); Chiral GC: 85:15 er (tR(mn) 79.3 min, tR(mj) 83.2 min); other data as above.
endo,exo-Bicyclo[3.3.0]octane-2,8-diol (5)
To a solution of hydroxyketone 4 (30 mg, 214 μmol) in MeOH (2.5 mL) at 0 °C was added NaBH4 (8 mg, 210 μmol). The resulting mixture was stirred at 0 °C for 1 h, neutralised by addition of aqueous HCl (2 M, few drops) and concentrated under reduced pressure. Purification of the residue by column chromatography (SiO2, EtOAc) gave diol 516 as a colourless oil (18 mg, 59%); Rf 0.20 (EtOAc); νmax/cm–1 3351s, 2946s, 1455m, 1350m, 1110m and 1065m; 1H NMR δ 4.32–4.25 (2H, m), 3.06 (2H, br s), 2.61–2.52 (1H, m), 2.25 (1H, dd, J 16.4 and 7.2), 2.01–1.89 (2H, m), 1.79–1.73 (1H, m), 1.64–1.47 (3H, m), 1.31–1.25 (1H, m) and 1.21–1.11 (1H, m); 13C NMR δ 74.1, 73.9, 54.8, 40.9, 35.7, 32.9, 30.9 and 29.3; m/z [CI + (NH3)] 160 (M + NH4+, 100%) 124 (30), 107 (20), 96 (20) and 80 (30) (Found: M + NH4+, 160.1336. C8H18NO2 requires 160.1338).
exo-8-(tert-Butyldimethylsilyloxy)bicyclo[3.3.0]octan-2-one (6)
A mixture of alcohol 4 (40 mg, 285 μmol), TBSCl (55 mg, 365 μmol) and imidazole (50 mg, 734 μmol) in DMF (0.5 mL) was stirred for 16 h and then partitioned between Et2O (2 mL) and H2O (2 mL). The organic layer was separated and washed with H2O (2 x 1 mL), dried, filtered and concentrated under reduced pressure. Purification of the residue by column chromatography (SiO2, 10% Et2O in petrol) gave silyl ether 6 as a colourless oil (57 mg, 79%); Rf 0.30 (10% Et2O in petrol); νmax/cm–1 2955s, 2857m, 1738s, 1463w, 1257m and 1050m; 1H NMR δ 4.31 (1H, br s), 3.00–2.91 (1H, m), 2.50 (1H, d, J 9.2), 2.30–2.03 (4H, m), 1.64–1.55 (3H, m), 1.42–1.33 (1H, m), 0.88 (9H, s), 0.08 (3H, s) and 0.07 (3H, s); 13C NMR δ 220.5, 77.9, 62.7, 39.5, 38.4, 36.5, 31.4, 26.9, 26.2, 18.4 and –4.4; m/z [GCMS CI + (NH3)] 272 (M + NH4+, 60%), 255 (M + H+, 65) and 197 (100) (Found: M + H+, 255.1788. C14H27O2Si requires 255.1780).
exo,endo-8-(tert-Butyldimethylsilyloxy)bicyclo[3.3.0]octan-2-ol (7)
To a solution of ketone 6 (52 mg, 204 μmol) in MeOH (2 mL) at 0 °C was added NaBH4 (12 mg, 317 μmol). The resulting mixture was stirred at 0 °C for 1 h, neutralised by addition of aqueous HCl (2 M, few drops) and concentrated under reduced pressure. Purification of the residue by column chromatography (SiO2, 10% Et2O in petrol) gave alcohol 7 as a colourless oil (31 mg, 59%); Rf 0.20 (10% Et2O in petrol); νmax/cm–1 3436m br, 2952s, 2858s, 1471m, 1463m and 1253m; 1H NMR δ 4.33–4.29 (2H, m), 2.57–2.51 (1H, m), 2.32–2.26 (1H, m), 2.01–1.92 (1H, m), 1.85–1.49 (5H, m), 1.32–1.26 (1H, m), 1.23–1.14 (1H, m), 0.88 (9H, s), 0.08 (3H, s) and 0.07 (3H, s); 13C NMR δ 74.3, 74.2, 56.0, 40.8, 36.7, 34.4, 31.0, 29.2, 25.8, 17.9, –4.3 and –4.8; m/z [GCMS CI + (NH3)] 257 (M + H+, 100%) (Found: M + H+, 257.1935. C14H29O2Si requires 257.1935).
endo,exo-Bicyclo[3.3.0]octane-2,8-diol (5)
To a solution of silyl ether 7 (25 mg, 98 μmol) in MeCN (0.5 mL) was added aqueous HF (48%, 100 μL). After 1 h the mixture was neutralised with solid NaHCO3, filtered and concentrated under reduced pressure. Purification of the residue by column chromatography (SiO2, EtOAc) gave diol 5 as a colourless oil (12 mg, 87%); data as above.
ACKNOWLEDGEMENTS
We thank the EPSRC and Roche for a CASE award (to M. A. H. S.), the John Fell OUP Research Fund and the Oxford Supercomputing Centre (support to R. S. P.), the EPSRC National Mass Spectrometry Service Centre for mass spectra, and Prof. M. Node (Kyoto) for NMR data of exo- and endo-4.
References
1. Current address: Key Organics Ltd, Highfield Industrial Estate, Camelford, Cornwall, PL32 9RA, UK.
2. Author to whom correspondence concerning the computational studies should be addressed (robert.paton@chem.ox.ac.uk).
3. Current address: Summit plc, 91 Milton Park, Abingdon, Oxfordshire, OX14 4RY, UK.
4. (a) G. Drake, 'Comprehensive Heterocyclic Chemistry III', Vol. 1, ed. by A. Padwa, A. R. Katritzky, C. A. Ramsden, E. F. V. Scriven, and R. J. K. Taylor, Elsevier, Oxford, 2008, pp. 173-233; (b) D. M. Hodgson and M. A. H. Stent, 'Comprehensive Heterocyclic Chemistry III', Vol. 1, ed. by A. Padwa, A. R. Katritzky, C. A. Ramsden, E. F. V. Scriven, and R. J. K. Taylor, Elsevier, Oxford, 2008, pp. 235-298. CrossRef
5. A. C. Cope, M. M. Martin, and M. A. McKervey, Q. Rev. Chem. Soc., 1966, 20, 119. CrossRef
6. J. K. Crandall and M. Apparu, Org. React. (N. Y.), 1983, 29, 345.
7. E. Doris, L. Dechoux, and C. Mioskowski, Synlett, 1998, 337. CrossRef
8. (a) T. Satoh, Chem. Rev., 1996, 96, 3303; CrossRef (b) G. Boche and J. C. W. Lohrenz, Chem. Rev., 2001, 101, 697; CrossRef (c) D. M. Hodgson and C. D. Bray, 'Aziridines and Epoxides in Organic Synthesis', ed. by A. K. Yudin, Wiley-VCH, Weinheim, Germany, 2006, pp. 145-184; CrossRef (d) D. M. Hodgson, C. D. Bray, and P. G. Humphreys, Synlett, 2006, 1; CrossRef (e) D. M. Hodgson, P. G. Humphreys, and S. P. Hughes, Pure Appl. Chem., 2007, 79, 269; CrossRef (f) D. M. Hodgson, Latvian J. Chem., 2009, 313.
9. D. M. Hodgson, I. D. Cameron, M. Christlieb, R. Green, G. P. Lee, and L. A. Robinson, J. Chem. Soc., Perkin Trans. 1, 2001, 2161. CrossRef
10. (a) D. M. Hodgson, M. A. H. Stent, and F. X. Wilson, Org. Lett., 2001, 3, 3401; CrossRef (b) D. M. Hodgson, M. A. H. Stent, and F. X. Wilson, Synthesis, 2002, 1445; CrossRef (c) D. M. Hodgson, C. R. Maxwell, T. J. Miles, E. Paruch, M. A. H. Stent, I. R. Matthews, F. X. Wilson, and J. Witherington, Angew. Chem., Int. Ed., 2002, 41, 4313, corrigendum, 2002, 41, 4611; CrossRef (d) D. M. Hodgson, M. A. H. Stent, B. Štefane, and F. X. Wilson, Org. Biomol. Chem., 2003, 1, 1139. CrossRef
11. (a) S. Moon, J. M. Takakis, and B. H. Waxman, J. Org. Chem., 1969, 34, 2951; CrossRef (b) A. J. Bloodworth, J. A. Khan, and M. E. Loveitt, J. Chem. Soc., Perkin Trans. 1, 1981, 621; CrossRef (c) L. A. Batory, C. E. McInnis, and J. T. Njardarson, J. Am. Chem. Soc., 2006, 128, 16054. CrossRef
12. D. Yang, M.-K. Wong, and Y.-C. Yip, J. Org. Chem., 1995, 60, 3887. CrossRef
13. (a) D. E. Justice and J. R. Malpass, Tetrahedron, 1996, 52, 11947; CrossRef (b) J. Armbruster, F. Stelzer, P. Landenberger, C. Wieber, D. Hunkler, M. Keller, and H. Prinzbach, Tetrahedron Lett., 2000, 41, 5483. CrossRef
14. (a) H. Nagata and K. Ogasawara, Tetrahedron Lett., 1999, 40, 6617; CrossRef (b) T. Inoue, K. Hosomi, M. Araki, K. Nishide, and M. Node, Tetrahedron: Asymmetry, 1995, 6, 31; CrossRef (c) G. C. Tsantali and I. M. Takakis, J. Org. Chem., 2003, 68, 6455. CrossRef
15. D. M. Hodgson and M. A. H. Stent, 'Organolithiums in Enantioselective Synthesis, Topics in Organometallic Chemistry', Vol. 5, ed. by D. M. Hodgson, Springer, Berlin, 2003, pp. 1-20. CrossRef
16. Comparison data for the three lowest field 13C NMR shifts (CDCl3) are as follows. Present work: δ220.8, 76.6, 61.7; M. Node, Personal Communication: exo-4: δ220.8, 76.7, 61.8 (endo-4: δ222.1, 74.6, 56.7); Ref. 14c: exo-4: δ220.9, 76.7, 61.9 (endo-4: δ222.1, 74.7, 56.9).
17. A. Pfenninger, A. Roesle, and R. Keese, Helv. Chim. Acta, 1985, 68, 493. CrossRef
18. F. Tang and K. D. Moeller, Tetrahedron, 2009, 65, 10863. CrossRef
19. (a) R. K. Boeckman, Jr., Tetrahedron Lett., 1977, 18, 4281; CrossRef (b) D. M. Hodgson, G. P. Lee, R. E. Marriot, A. J. Thompson, R. Wisedale, and J. Witherington, J. Chem. Soc., Perkin Trans. 1, 1998, 2151. CrossRef
20. (a) A. N. Kasatkin and R. J. Whitby, Tetrahedron Lett., 2000, 41, 5275; CrossRef (b) P. Kocienski and C. Barber, Pure Appl. Chem., 1990, 62, 1933. CrossRef
21. T. Hudlicky and M. Natchus, J. Org. Chem., 1992, 57, 4740. CrossRef
22. J. Clayden and S. A. Yasin, New J. Chem., 2002, 26, 191. CrossRef
23. For an example, see: E. Fujita, M. Shibuya, S. Nakamura, Y. Okada, and T. Fujita, J. Chem. Soc., Perkin Trans. 1, 1974, 165. CrossRef
24. Gaussian 09, Revision A.02, Gaussian, Inc., Wallingford CT, 2009.
25. Optimisations with one explicit molecule of solvent (THF) provide geometries and energetics much in line (activation barrier 45 kcal/mol) with the continuum solvation results. Calculations with two molecules of THF failed to converge (due to a rather flat energy surface).
26. Calculations with an explicit THF molecule show very similar geometries, and while absolute free energies are shifted down in favour of the products, the preference of the endo-β-lithiooxyketone endo-4-Li is maintained over the exo-diastereomer, albeit by a smaller margin of 1 kcal/mol.
27. A. C. Cope, H.-H. Lee, and H. E. Petree, J. Am. Chem. Soc., 1958, 80, 2849. CrossRef
28. A related allene oxide has been proposed as an intermediate in the base-induced rearrangement of the diepoxide (epoxy groups trans) of 1, see: M. Apparu and M. Barrelle, Bull. Soc. Chim. Fr., 1984, II, 156.
29. T. H. Chan and B. S. Ong, Tetrahedron, 1980, 36, 2269. CrossRef
30. J. K. Crandall and L.-H. Chang, J. Org. Chem., 1967, 32, 532. CrossRef
31. For the preparation of i-PrLi, see: M. Stent, ChemSpider SyntheticPages, 2002, http://cssp.chemspider.com/195.