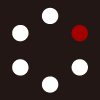
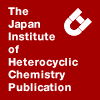
HETEROCYCLES
An International Journal for Reviews and Communications in Heterocyclic ChemistryWeb Edition ISSN: 1881-0942
Published online by The Japan Institute of Heterocyclic Chemistry
e-Journal
Full Text HTML
Received, 20th June, 2011, Accepted, 8th July, 2011, Published online, 20th July, 2011.
DOI: 10.3987/COM-11-12287
■ Estrogen Receptor α/β Ligands Derived from Thalidomide
Tomomi Noguchi-Yachide,* Kazuyuki Sugita, and Yuichi Hashimoto
Institute of Molecular and Cellular Biosciences, The University of Tokyo, 1-1-1 Yayoi, Bunkyo-ku, Tokyo 113-0032, Japan
Abstract
We have developed a series of thalidomide-derived phthalimide-type estrogen receptor (ER) modulators with characteristic subtype (ERα/ERβ) selectivity, and investigated their structure-activity relationship for ER agonistic and antagonistic activities.INTRODUCTION
Estrogen receptors (ERα and ERβ) are members of the nuclear receptor superfamily (a family of ligand-dependent transcription factors that modulate expression of specific genes and thereby influencediverse biological processes, including cell growth, differentiation and metabolism).1,2 The major endogenous ligands of ERs are estrogens, such as estrone (E1), 17β-estradiol (E2) and estriol (E3), and several synthetic ER modulators, including tamoxifen and raloxifene (6) (Figure 1), have been reported.1-3 Estrogens play a central role in female reproductive function and proliferation of uterine and vaginal epithelium cells through binding to the ERs, which function as ERα or ERβ homodimers or ERα/β heterodimer.1,2,4 ERs exist not only in normal cells, including uterine and ovarian cells, but also in a number of cancer cells, such as breast cancer cells and uterine cancer cells, and cancer growth is supported through the ERs.1,5,6 Furthermore, estrogens regulate expression of their target genes and have important roles in a variety of physiological and pathological states in both males and females, including glucose homeostasis, insulin resistance, regulation of the circulating levels of cholesterol and lipids, and regulation of bone density, in addition to their role in the female reproductive system.7-9
We have been engaged in structural development studies of thalidomide (1) (Figure 1). Thalidomide (1) is a sedative/hypnotic agent, which was withdrawn from the market because of its serious teratogenicity, but has subsequently shown potential for the treatment of a range of diseases, including cancers, diabetes, and rheumatoid arthritis.10-12 Tumor necrosis factor alpha (TNF-α) production-inhibitory activity was initially considered to be one of the key mechanisms of thalidomide’s actions, but thalidomide (1) has also been discovered to have various other biological activities, including anti-inflammatory, anti-angiogenic, and cyclooxygenase (COX)-inhibitory activities.10-14 However, these activities are thought to be linked only partially to the pharmacological effects elicited by thalidomide (1). Although the precise molecular mechanisms of its actions remain unclear, current research suggests that thalidomide (1) acts on many target molecules and is thus a multi-target agent. Thalidomide (1) is easily metabolized in vivo, and various metabolites of thalidomide (1), such as compounds 2-4, have been reported.15-22 Not only thalidomide itself, but also its metabolites, play an important role in the pharmacological effects of thalidomide (1).
The multi-target nature of thalidomide (1) suggested that superior lead compounds for various target molecules could be created by structural development of thalidomide (1). The structural resemblance between synthetic ER ligands, including diethylstilbestrol (10) and raloxifene (11) (Figure 2), and thalidomide metabolites 2 and 3 (Figure 1) led us to speculate that (i) the anti-carcinogenic activity of thalidomide (1) might be related to functions of ERs, and/or (ii) novel ER ligands might be created by structural development of thalidomide (1). Therefore, we performed structure-activity relationship studies of thalidomide derivatives for ERα and ERβ agonistic and antagonistic activities.
RESULTS
We investigated the effects of our compounds on ERs using a reporter gene assay method with CMX-GAL4N-hER as the recombinant receptor gene, TK-MH100x4-LUC as the reporter gene, and the CMX β-galactosidase gene for normalization, as previously reported.23-25 As shown in Table 1, agonistic activity of test compounds was expressed as EC50 values, which were estimated from the sigmoidal dose-response curves using R software. The results of ER-antagonistic activity assay in the presence of 0.3 nM E2 are shown in Table 2. Antagonistic activity of test compounds was expressed as IC50 values, which were estimated from the sigmoidal dose-response curves using R software. “N.A.” means no activity at 30 μM in Tables 1 and 2 and “>30” means that the compound does not achieve 50% inhibition at 30 μM in Table 2.
First, we evaluated the ERα and ERβ agonistic and antagonistic activities of thalidomide (1), its major metabolites (2-4) (Figure 1), and some simple phthalimide derivatives, 5-9 (Figure 2). The derivatives 5, 6, 8 and 9 were designed to possess a halogen atom or a hydroxyl group at the position corresponding roughly in the molecular shape to one of the phenolic hydroxyl groups of diethylstilbestrol (10) (a synthetic nonsteroidal estrogen, Figure 2) or raloxifene (11) [a selective estrogen modulator (SERM)1,2, Figure 2]. However, compounds 1〜9 showed neither agonistic nor antagonistic activity towards ERα and ERβ. These results, together with our previous finding that the N-phenylphthalimide skeleton is a superior template for various biologically active compounds,10-14 prompted us to convert the glutalimide group of thalidomide to a substituted or non-substituted N-phenyl group.
Structure-activity relationship of N-phenylphthalimide derivatives: Agonistic activity
PP-00 (12), which has no substituents in the N-phenyl group, did not show agonistic activity toward either ERα or ERβ. Introduction of a hydroxyl group into the phthaloyl group at the 5-position, i.e., 5HPP-00 (21), resulted in the appearance of moderate agonistic activity toward both ERα and ERβ with EC50 values of 1.30 μM and 2.74 μM, respectively. 4HPP-00 (17), which is a regio-isomer of 21, did not show agonistic activities, suggesting the importance of the meta-hydroxyl group for the activity. We then investigated the effects of a hydroxyl group introduced into the N-phenyl group of PP-00 (12). PP-H (13), PP-0H (15) and PP-00H (16), which possess a hydroxyl group at the 2’-, 3’- or 4’-position, respectively, did not show agonistic activity toward either ERα or ERβ. Among the hydroxylated N-phenylphthalimide derivatives so far mentioned (13, 15, 16, 17, 21), only 5HPP-00 (21) showed ER agonistic activity. Next, we converted the hydroxyl group of 5HPP-00 (21) to an amino or a nitro group, i.e., 5APP-00 (25) and 5NPP-00 (26), respectively. Both 5APP-00 (25) and 5NPP-00 (26) showed agonistic activity toward ERα and ERβ. The corresponding regio-isomers, i.e., 4APP-00 (19) and 4NPP-00 (20), respectively, were inactive, as was the case for the hydroxyl compounds 5HPP-00 (21: active)/4HPP-00 (17: inactive).
Then, we investigated the effects of substituents introduced at the 4’-position of the N-phenyl group. The agonistic activity of compounds with a 4’-substituent increased in the order of: 5HPP-0CF (23) < 5HPP-0I (24) < 5HPP-0F (22) < 5HPP-00 (21), for both ER α and ER β. 4HPP-0N0CF (18), which possesses a nitro group at the 4’-position, did not show agonistic activity. Thus, introduction of a substitutent at the 4’-position seems to reduce the activity.
Generally speaking, molecular planarity is one of critical factors affecting the activity of nuclear receptor ligands. Therefore, introduction of two alkyl groups at the 2’- and 6’-positions, which will change the torsion angle between the two aromatic groups of N-phenylphthalimide derivatives, was investigated. Among the prepared N-2’,6’-dialkylphenylphthalimide derivatives [PP-00 (12), PP-11 (27), PP-22 (31), PP-33 (35)], only PP-11 (27) showed agonistic activity toward both ERα and ERβ with EC50 values of 2.77 μM and 12.1 μM, respectively. For 5HPP-00 derivatives, the agonistic activity increased in the order of: 5HPP-22 (33) ~ 5HPP-33 (36) << 5HPP-00 (12) < 5HPP-11 (28), for both ERα and ERβ. The 2’,6’-dimethylphenyl analog 5HPP-11 (28) showed the most potent activity among this series of the compounds, as was the case in the PP-series. 5HPP-11 (28) showed the most potent agonistic activities among our thalidomide-derived test compounds, with EC50 values of 0.0577 μM and 0.768 μM for ERα and ERβ, respectively.
We next investigated the effects of 2’,6’-dialkyl substitution of derivatives possessing a substituent other than a hydroxyl group at the 5-position, i.e., the 5APP series (5-amino derivatives) and 5NPP series (5-nitro derivatives). In the 5APP series, the ERα/β-agonistic activity increased in the order of: 5APP-33 (40) << 5APP-00 (25) << 5APP-11 (29) < 5APP-22 (33). In the 5NPP series, the activity increased in the order of: 5NPP-00 (26) < 5NPP-33 (41) << 5NPP-11 (30) < 5NPP-22 (34), for both ER α and ER β. The 2’,6’-diethyl derivatives [5APP-22 (33) and 5NPP-22 (34)] showed the most potent ERα/β-agonistic activities among the 5-amino- or 5-nitro-substituted derivatives, while the 2’,6’-dimethyl derivative was the most potent among the 5-hydroxy-substituted derivatives. 5APP-22 (33) and 5NPP-22 (34) showed ERα/β-agonistic activity with EC50 values of 0.0820 μM and 0.705 μM for ERα, respectively, and 0.397 μM and 3.20 μM for ERβ, respectively. The results suggest that a 5-substituted N-2’,6’-dimethylphenyl- or 5-substituted N-2’,6’-diethylphenylphthalimide skeleton is a structural requirement for potent ERα/β-agonistic activity, with a tendency for ERα-selectivity (Fig. 3). Interestingly, 5NPP-33 (41) was found to be a selective ERα agonist and its EC50 value was estimated to be 3.95 μM for ERα under our experimental conditions. A regio-isomer of 5APP-11, i.e., 5APP-0101 (51), with 3’,5’-dimethyl substituents, possesses quite weak (if any) ERα agonistic activity and moderate ERβ agonistic activity (EC50 is 3.20 μM for ERβ), so that it is a selective ERβ agonist. 5NPP-33 (41) possesses ERα agonistic activity, while its 1-decarbonylated analogue, 5NIDO-33 (49), is inactive. The 1,3-decarbonylated analogues (43-49, 52-55) tested in our experiments were all inactive. These results suggest an important role of the 1,3-dicarbonyl moiety on the phthalimide group in ERα/β-agonistic activity.
Structure-activity relationship of N-phenylphthalimide derivatives: Antagonistic activity
As shown in Table 2, PP-00 (12), N-hydroxylphenyl phthalimide derivatives [PP-H (13), FPP-H (14), PP-0H (15) and PP-00H (16)] and 4-substituted-phenyl phthalimide derivatives [4HPP-00 (17), 4HPP-H0N0CF (18), 4APP-00 (19) and 4NPP-00 (20)] showed no or very weak ERα/β-antagonistic activity. Among the 5-substituted phenylphthalimide derivatives [5HPP-00 (21), 5HPP-0F (22), 5HPP-0CF (23), 5HPP-0I (24), 5APP-00 (25) and 5NPP-00 (26)], only 5HPP-0F (22) showed moderately selective ERα antagonistic activity.
Among the N-2’,6’-dialkylphenylphthalimide derivatives [PP-00 (12), PP-11 (27), PP-22 (31), PP-33 (35)], only PP-33 (35) showed ERβ antagonistic activities, with an IC50 value of 13.0 μM under our experimental conditions. All the N-3’,5’-dialkylphenylphthalimide analogues investigated in our experiments [4APP-0101 (50), 5APP-0101 (51), 5AIDO-0101 (52), 6AIDO-0101 (53), 7AIDO-0101 (54) and 5AIDOL-0101 (55)] were inactive. These results suggest that 2’,6’-disubstitution of the N-phenyl group is a structural requirement for both ERα- and ERβ-antagonistic activity, as was the case for ERα/β-agonistic activity.
The ERα antagonistic activity of 5-hydroxy-2’,6’-dialkylphenylphthalimide derivatives [5HPP-11 (28), 5HPP-22 (32) and 5HPP-33 (36)] increased in the order of 5HPP-11 (dimethyl: 28) < 5HPP-22 (diethyl: 32) < 5HPP-33 (di-isopropyl: 36). In the case of 5-amino-2’,6’-dialkylphenylphthalimide derivatives [5APP-11 (29), 5APP-22 (33) and 5APP-33 (40)], the order of antagonistic potency toward ERβ showed the same tendency as in the case of the 5HPP series of compounds, i.e., 5APP-11 (dimethyl: 29) < 5APP-22 (diethyl: 33) < 5APP-33 (di-isopropyl: 40). Interestingly, the order was just reversed in the case of antagonistic activity toward ERα, i.e., 5APP-33 (di-isopropyl: 40) < 5APP-22 (diethyl: 33) < 5APP-11 (dimethyl: 29). These results suggest that (i) introduction of two small alkyl groups (that is, methyl groups) at the 2’- and 6’-positions of the N-phenyl group is favorable for ERα-antagonistic activity, while (ii) introduction of larger alkyl groups (isopropyl groups) at the corresponding positions is favorable for ERβ-antagonistic activity (Figure 3). Among 5-nitro-2’,6’-dialkylphenylphthalimide derivatives [5NPP-11 (30), 5NPP-22 (34) and 5NPP-33 (41)], only 5NPP-33 (41) showed ERα/β-antagonistic activity, though it was weak. Possibly the introduction of an electron-donating group at the 5-position is critical for potent ERα/β-antagonistic activity (Figure 3). Overall, 5HPP-33 (36) is the most potent ERα/β dual antagonist among our test compounds, and its IC50 values are 0.772 μM for ERα and 0.630 μM for ERβ. Further, 5HPP-11 (28) and 5APP-33 (40) were ERα-selective and ERβ-selective antagonists, respectively, as shown in Table 2. The IC50 values are 0.915 μM for 5HPP-11 (28) toward ERα (at least more than 33-fold selectivity over ERβ) and 1.53 μM for 5APP-33 (40) toward ERβ.
CONCLUSION
Although thalidomide and its metabolites do not show ER-modulating activity, ERα/ERβ agonists and antagonists were structurally developed based on the thalidomide-derived phthalimide skeleton. Their structure-activity relationship was examined. We discovered compounds with ERα- and ERβ-selective activity, including 5NPP-33 (41) (an ERα selective agonist), 5APP-0101 (51) (an ERβ selective agonist), and 5APP-33 (40) (an ERβ selective antagonist). In addition, we discovered compounds with potent ERα/ERβ dual agonist/antagonist activity, such as 5HPP-11 (28), 5APP-22 (33) and 5HPP-33 (36).
EXPERIMENTAL
Synthesis
The synthesis of compounds (5-31, 33, 35-37, 40-55) has been reported in references 27-31.
General
2-(2,6-Diethylphenyl)-substituted isoindoline-1,3-dione (32, 34)
A mixture of substituted phthalic anhydride and 2,6-diethylaniline was heated at 180 ºC for 2 h. The reaction mixture was cooled to 0 ºC, and n-hexane was added. The precipitated solid was collected by filtration, washed with cold n-hexane, and dried under reduced pressure. The structure were confirmed by NMR and mass spectroscopy, and gave appropriate analysis data.
2-(2,6-Diethylphenyl)-5-hydroxyisoindoline-1,3-dione (5HPP-22, 32):
Mp 174.0-178.0 ºC. FAB-MS m/z 296 (M+H)+; 1H-NMR (500MHz, CDCl3): δ 7.83 (d, J = 7.9 Hz, 1H), 7.37 (t, J = 7.9 Hz, 1H), 7.36 (d, J = 1.8 Hz, 1H), 7.23 (d, J = 7.9 Hz, 2H), 7.15 (dd, J = 7.9, 1.8 Hz, 1H), 6.17 (s, 1H), 2.46 (q, J = 7.3 Hz, 2H), 1.14 (t, J = 7.3 Hz, 3H), 3.38. Anal. Calcd for C18H17NO3: C, 73.20; H, 5.80; N, 4.74. Found: C, 73.13; H, 5.91; N, 4.66.
2-(2,6-Diethylphenyl)-5-nitroisoindoline-1,3-dione (5NPP-22, 34):
Mp 145.5-147.0 ºC. FAB-MS m/z 325 (M+H)+; 1H-NMR (500MHz, CDCl3): δ 8.80 (d, J = 1.8 Hz, 1H), 8.70 (dd, J = 8.5, 1.8 Hz, 1H), 7.17 (d, J = 8.5 Hz, 1H), 7.43 (t, J = 7.9 Hz, 1H), 7.27 (d, J = 7.9 Hz, 2H), 2.44 (q, J = 7.3 Hz, 2H), 1.14 (t, J = 7.3 Hz, 3H). HRMS (FAB) Calcd for C18H17N2O4: 325.1188. Found: 325.1226 (M+H)+.
2-(2,6-Diisopropylphenyl)-4,7-dihydroxy-5-substituted isoindoline-1,3-dione (38, 39)32
To a prepared THF solution of (TMP)2Cu(CN)Li2 was added 5-substituted PP-33 at -78 ℃ under Ar. The reaction mixture was stirred for 3 h at 0 ℃, then tBuOOLi was added at -78℃ and the mixture was stirred at -78 ℃ for 16 h. It was then poured into saturated aqueous NH4Cl and NaHS2O3, and extracted with AcOEt. The AcOEt layer was dried over Na2SO4, and the solvent was removed by evaporation in vacuo. Purification by silica gel flash chromatography gave 5OMe4,7DHPP-33 (38) and 4,7DHPP-33 (39), which were recrystallized from AcOEt/n-hexane to give yellow solids. Each structure were confirmed by NMR and mass spectroscopy, and gave appropriate analysis data (TMP: 2,2,6,6-tetramethylpiperidino).
2-(2,6-Diisopropylphenyl)-4,7-dihydroxy-5-methoxyisoindoline-1,3-dione (5OMe4,7DHPP-33, 38):
Mp 136.5-138.0 ºC. FAB-MS m/z 339 (M+); 1H-NMR (400MHz, CDCl3): δ 7.47 (d, J = 8.0 Hz, 1H), 7.28 (m, 4H), 2.73 (sept, J = 6.8 Hz, 2H), 1.18 (d, J = 6.8 Hz, 12H). HRMS (FAB) Calcd for C20H21NO4: 339.1471. Found: 339.1450.
2-(2,6-Diisopropylphenyl)-4,7-dihydroxyisoindoline-1,3-dione (4,7DHPP-33, 39):
Mp 162.0-163.5 ºC. FAB-MS m/z 369 (M+); 1H-NMR (400MHz, CDCl3): δ 7.45 (t, J = 7.8 Hz, 1H), 7.28 (d, J = 8.1 Hz, 2H), 6.68 (s, 1H), 3.92 (s, 3H), 2.74 (sept, J = 6.6 Hz, 2H), 1.17 (d, J = 6.6 Hz, 12H). HRMS (FAB) Calcd for C21H23NO5: 369.1576. Found: 369.1551; Anal. Calcd for C21H23NO5: C, 68.28; H, 6.28; N, 3.69. Found: C, 67.33; H, 6.77; N, 3.35.
Reporter Gene Assay23-25
Human embryonic kidney (HEK) 293 cells were cultured in Dulbecco’s modified Eagle’s medium (phenol red free) containing 5% fetal bovine serum at 37 ºC in a humidified atmosphere of 5% CO2 in air. Transfections of CMX-GAL4N-hERα, CMX-GAL4N-hERβ and CMX-β-GAL into HEK 293 cells were performed by the calcium phosphate coprecipitation method. Test compounds, with or without 17β-estradiol, were added 7 hours after the transfection. After overnight incubation, luciferin was added and luminescence was measured on an Wallac ARVOTM-SX (Perkin Elmer) microplate reader. Then, β-galactosidase was added and the absorbance was measured on the microplate reader with emission detection at 405 nm. Reporter gene assay was performed in triplicate (n=3).
ACKNOWLEDGEMENTS
The work described in this paper was partially supported by Grants-in-Aid for Scientific Research from The Ministry of Education, Culture, Sports, Science and Technology, Japan, and the Japan Society for the Promotion of Science.
References
1. V. C. Jordan, J. Med. Chem., 2003, 46, 883. CrossRef
2. V. C. Jordan, J. Med. Chem., 2003, 46, 1081. CrossRef
3. G. G. J. M. Kuiper, B. Carlsson, K. Grandien, E. Enmark, J. Haggblad, S. Nillson, and J. Gustafsson, Endocrinology, 1997, 138, 863. CrossRef
4. X. Li, J. Huang, P. Yi, R. A. Bambara, R. Hilf, and M. Mutan, Mol. Cell. Biol., 1999, 19, 1919.
5. S. Green, P. Walter, V. Kumar, A. Krust, J. Bornert, P. Argos, and P. Chambon, Nature, 1986, 320, 134. CrossRef
6. G. G. J. M. Kuiper, E. Enmark, M. Pelto-Huikko, S. Nilsson, and J. Gustafsson, Pro. Nat. Acad. Sci. USA, 1996, 93, 5925. CrossRef
7. P. Chambon, Mol. Endocrinol., 2005, 19, 1418. CrossRef
8. S. Nilsson, S. Makela, E. Treuter, M. Tujague, J. Thamsen, G. Andersson, E. Enmark, K. Pettersson, M. Warner, and J. Gustafsson, Physiol. Rev., 2001, 81, 1535.
9. R. P. A. Barros, U. F. Machado, and J. Gustafsson, Trends. Mol. Med., 2006, 12, 425. CrossRef
10. Y. Hashimoto, Curr. Med. Chem., 1998, 5, 163.
11. Y. Hashimoto, Bioorg. Med. Chem., 2002, 10, 461. CrossRef
12. Y. Hashimoto, A. Tanatani, K. Nagasawa, and H. Miyachi, Drugs Future, 2004, 29, 383. CrossRef
13. J. B. Bartlett, K. Dredge, and A. G. Dalglish, Nat. Rev. Cancer, 2004, 4, 314. CrossRef
14. J. B. Bartlett, A. Tozer, D. Stirling, and J. B. Zeldis, British J. Cancer, 2005, 93, 613. CrossRef
15. J. Lu, N. Hesby, B. D. Palmer, M. Tingle, B. C. Baguley, P. Ketstell, and L.-M. Ching, J. Pharm. Exp. Ther., 2004, 310, 571. CrossRef
16. J. Lu, B. D. Palmer, P. Kestell, P. Browett, B. C. Baguley, G. Muller, and L.-M. Ching, Clin. Cancer Res., 2003, 9, 1680.
17. M. G. Marks, J. Shi, M. O. Fry, Z. Xiao, M. Trzyna, V. Pokala, M. A. Ihnat, and P.-K. Li, Biol. Pharm. Bull., 2002, 25, 597. CrossRef
18. S. Robin, J. Zhu, H. Galons, C. Pham-Huy, J. R. Vlaude, A. Tomas, and B. Viossat, Tetrahedron: Assymetry, 1995, 6, 1249. CrossRef
19. U. Teubert, K. Zwingenberger, S. Wnendt, and K. Eger, Arch. Pharm., 1998, 331, 7. CrossRef
20. F. A. Luzzio, D. Y. Duveau, E. R. Lepper, and W. D. Figg, J. Org. Chem., 2005, 70, 10117. CrossRef
21. M. Doi, Y. Ijiri, M. Akagi, M. Uenishi, and H. Urata, Anal. Sci., 2003, 19, x51.
22. M. Meyring, J. Muehlbacher, K. Messer, N. Kastner-Pustet, G. Bringmann, A. Mannschreck, and G.Blaschke, Anal. Chem., 2002, 74, 3726. CrossRef
23. M. Makishima, T. T. Lu, W. Xie, G. K. Whitfield, H. Domoto, R. M. Evans, M. R. Haussler, and D. J. Mangelsdorf, Science, 2002, 296, 1313. CrossRef
24. M. Makishima, A. Y. Okamoto, J. J. Repa, H. Tu, R. M. Learned, A. Luk, M. V. Hull, K. D. Lustig, D. J. Mangelsdorf, and B. Shan, Science, 1999, 284, 1362. CrossRef
25. J. Kasuga, M. Makishima, Y. Hashimoto, and H. Miyachi, Bioorg. Med. Chem. Lett., 2006, 16, 554. CrossRef
26. A. M. Brzozowsk, A. C. W. Pike, Z. Dauter, R. E. Hubbard, T. Bonn, O. Engström, L. Öhman, G. L. Greene, J. Gustafsson, and M. Carlquist, Nature, 1997, 389, 753. CrossRef
27. Y. Shibata, K. Sasaki, Y. Hashimoto, and S. Iwasaki, Biochem. Biophys. Res. Commun., 1994, 205, 1992. CrossRef
28. Y. Shibata, K. Sasaki, Y. Hashimoto, and S. Iwasaki, Chem. Pharm. Bull., 1996, 44, 156.
29. M. Tetsuhashi, M. Ishikawa, M. Hashimoto, Y. Hashimoto, and H. Aoyama, Bioorg. Med. Chem., 2010, 18, 5323. CrossRef
30. R. Shimazawa, H. Takayama, Y. Fujimoto, M. Komoda, K. Dodo, R. Yamasaki, R. Shirai, Y. Koiso, K. Miyata, F. Kato, M. Kato, H. Miyachi, and Y. Hashimoto, J. Enz. Inhib. Med. Chem., 1999, 14, 259. CrossRef
31. M. Suizu, Y. Muroya, H. Kakuta, H. Kagechika, A. Tanatani, K. Nagasawa, and Y. Hashimoto, Chem. Pharm. Bull., 2003, 51, 1098. CrossRef
32. S. Usui, Y. Hashimoto, J. V. Morey, A. E. H. Wheatley, and M. Uchiyama, J. Am. Chem. Soc., 2007, 129, 15102. CrossRef